Abstract
We model the observed precision differential abundance patterns of three G-type dwarfs, assuming a mass of planetary or disk material was added to or subtracted from the atmosphere of the star. The two-parameter model is fit by linear regression. The stellar abundance patterns are corrected for Galactic chemical evolution (GCE). The differential abundances can be highly correlated with the elemental condensation temperature. We show how it is possible to match not only the slope, but the quantitative abundance differences, assuming a composition for the added material equal to that of the bulk earth. We also model the wide pair HIP 34407 and HIP 34426, where GCE corrections are assumed unnecessary. An appendix discusses issues of volatility and condensation temperature.
Export citation and abstract BibTeX RIS
1. Introduction
The literature on precision differential abundances (PDAs) in stars is extensive. Surveys include Sun-like stars in the solar neighborhood, binary systems, and Galactic clusters. Numerous references as well as a discussion of relevant mechanisms may be found in papers by Ramírez et al. (2019) and Nagar et al. (2020). A strong impetus for this work is the probability that the abundances have been influenced by exoplanetary systems and their evolution.
We calculate the resulting differential abundances ([El/H]) assuming a given amount of material with the composition of the bulk Earth (Wang et al. 2018, henceforth, WN18) was added to the stellar convection zone of a dwarf G-type star. The mass of the convection zone is uncertain and variable, depending on the spectral type. Here, we assume a mass of 5 · 1031 gm for the stellar convection zone (SCZ). This is 0.025 M⊙ (see Pinsonneault et al. 2001; Chambers 2010). For other SCZ masses, the parameters must be adjusted accordingly. In general, the Sun-like star will not have exactly the solar composition. This contingency is roughly taken into account in our model.
2. The Model
Let MSCZ be the mass of the stellar convection zone. We use a bulk Earth mass of MBE = 6 · 1027 gm, with the composition from WN18. Our model assumes that as a result of the addition of q Earth masses of material, the stellar abundance of all elements will be increased by a factor of

Note that the ratio of the masses, bulk Earth and SCZ, is the same as the ratio of the number of atoms in the bulk Earth and the SCZ.
The parameter p allows for the possibility that the star may have abundances that differ slightly from those of the Sun. If p < 1, the star will be slightly metal-poor, and conversely, if p > 1, it will be slightly metal-rich. Generally, we expect that p will be close to unity. We assume that the abundances vary in lockstep, and neglect small changes in the atmospheric structure resulting from the added material.
Using bracket notation, where ,

Rewriting Equation (2) yields

We may obtain p and q by linear regression, as there is one equation for every differential elemental abundance. The regression fit includes the results of an F-test in which the F statistic (the ratio of the varance for a one-parameter fit to the variance for a two-parameter fit) is calculated and used to establish a significance based on the Snedecor F distribution. In this formalism, larger values of the F statistic yield smaller significance measures; outcomes with significances substantially less than 0.01 demonstrate the superiority of the linear (two parameter) fit over that of the flat line (one parameter or mean) fit. Values for p, q, F, and the significance are included in the discussions of the examples below.
3. Examples
Adibekyan et al. (2014) pointed out that, in addition to correlations of differential abundances of solar-like stars with condensation temperature, Tc, 4 the PDAs also correlated with the stars' ages and/or locations in the disk. These connections are readily seen as a consequence of Galactic chemical evolution (GCE), whose overall effect is to convert mostly light, volatile elements like carbon, nitrogen, and oxygen into heavier, refractory elements like iron, zirconium, and barium. Thus, stars younger than the Sun are expected to be depleted in volatiles like C, N, and O but enhanced in refractories, such as Fe, Zr, and Ba, and heavier elements. This leads to a positive correlation of their differential abundances with condensation temperature. Note that most of the observable heavy elements have high Tc. There are, of course, exceptions to this rough trend. Some heavy elements, such lead and mercury, are volatile, and the heavier noble gasses are ultra volatile. However abundance determinations for these elements are not usually available for G dwarfs.
It is necessary to subtract the effects of GCE prior to examining the differential abundances for possible effects of addition or subtraction of planetary material. This was done by Cowley et al. (2020a) 5 in their study of Tc correlations among the 79 stars of Bedell et al. (2018, henceforth BD18) which included the first three examples below. BD18 give precision differential abundances for the following 30 elements: C, O, Na, Mg, Al, Si, S, Ca, Sc, Ti, V, Cr, Mn, Fe, Co, Ni, Cu, Zn, Sr, Y, Zr, Ba, La, Ce, Pr, Nd, Sm, Eu, Gd, and Dy.
In addition to GCE, the fascinating results of Meléndez et al. (2014) for 18 Sco show that the abundances of neutron-addition (nA) elements (Sr–Dy in BD18) may be affected by local nucleosynthetic events unrelated to general GCE. In the current article we have not, therefore, included the nA elements in the fits of [El/H] to Tc for HD 196390, HD 42618, and HD 140583.
3.1. Data Sources
The calculations presented below require the use of literature sources for condensation temperature, the composition of the bulk Earth, and the composition of the convection zone. We have made numerous calculations based on different combinations of data sources, with the conclusion that for our purposes, differences in numerical values in the data sources are not significant.
In the current article, we report calculations from models based on two sets of data sources:
- 1.set I: solar photospheric abundances from Asplund et al. (2009), Amarsi et al. (2018, 2019, 2020), Grevesse et al. (2015), Amarsi & Asplund (2017), Scott et al. (2015), Lind et al. (2017), Bergemann et al. (2017, 2019), Norlander & Lind (2017), Carlos et al. (2019), Lawler et al. (2019), Osorio et al. (2019), Riggiani et al. (2019), Young (2018), condensation temperatures from Wood et al. (2019), and bulk Earth composition from Wang et al. (2018); and
- 2.
We emphasize that for our purposes, which involve only the elements between carbon and zinc, the choice of data sources is not critical. As an illustration, we show in Table 1 that the derived model parameters of Equation (3), p and q, in data sets I and II agree well.
Table 1. Model Parameters from Two Data Sets
Set | Star(s) | p | q | F | Sig |
---|---|---|---|---|---|
Set I | HD 196390 | 1.07 | 5.0 | 54.0 | 1.4 E-07 |
Set II | 1.09 | 5.4 | 38.8 | 1.2 E-06 | |
Set I | HD 42618 | 0.81 | −1.4 | 6.3 | 1.0E-02 |
Set II | 0.80 | −1.6 | 6.3 | 1.0E-02 | |
Set I | HD 140538 | 0.97 | 4.6 | 19.1 | 7.5 E-05 |
Set II | 1.00 | 4.4 | 8.3 | 3.7E-03 | |
Set I | HIP34407/34426 | 1.28 | 8.2 | 19.7 | 2.9E-05 |
Set II | 1.31 | 6.6 | 14.9 | 1.5E-04 |
Download table as: ASCIITypeset image
3.2. HD 196390 (HIP 101905)
In Cowley et al. (2020a), six stars had differential abundances with significant ( <6.0 ·10−5) Tc correlations; the tightest correlation was for HD 196390.
Model fits are shown in Figure 1 based on data sets I and II of Table 1. The straight line is an unweighted least-squares fit to [El/H] versus Tc for carbon through zinc. The linear regression solution yielding p and q is also for these elements. Diffferences between observations and models, for both sets I and II, are typically within the total uncertainties of the abundance measures, including the observational and analysis errors, plus those associated with the GCE correction parameters from BD18. These errors are indicated in the left plot. The small black dots designate the nA elements which were excluded from the modeling trials and the regression fits.
Figure 1. Left: model predictions (red crosses) and observed differential stellar abundances (large black points) for HD 196390 vs. condensation temperature, Tc, after GCE corrections. The parameters are from set I of Table 1. The predicted abundances are from a slightly metal-rich model (p = 1.07). The parameter q = 5.0 implies that five Earth masses were ingested. See the text for further explanation of the symbols. Right: model predictions and observed differential stellar abundances for HD 196390 based on the parameters of set II of Table 1. Symbols have the same meaning as they do in the left panel. The two plots are closely similar. The predicted point (red plus sign at Tc = 1158 K) some 0.02 dex below the mean relation indicated by the solid black line is for Mn.
Download figure:
Standard image High-resolution image3.3. HD 42618 (HIP 29432)
Among the stars of BD18 noted by Cowley et al. (2020a), HD 42618 was unusual because of its negative slope, and because it has a known planet containing some 14.4 Earth masses. The formal probability that an unweighted linear fit of [El/H] with Tc occurs by chance was 2.1 · 10−5, but the two-parameter linear regression fit is only of marginal significance. Snedecor's F-parameter is 6.3, leading to a significance level 0.010 (see Figure 2, left). Data sets I and II yield p <1 for this star, indicating that HD 42618 is slightly metal-poor with respect to the Sun. The negative values of q suggest a deficit of Earth-like material relative to the Sun.
Figure 2. Left: model predictions and observed differential stellar abundances for HD 42618 vs. condensation temperature after GCE corrections. The model abundances are slightly metal-poor. The negative q-parameter implies that refractory mass is missing from HD 42618 relative to the Sun. Right: model predictions and observed differential abundances for HD 140538 vs. condensation temperature after GCE corrections. The model abundances are close to solar, with ≈4.5 Earth masses of added material needed to optimize the fit to the observed PDAs. Both plots use data and results from set I.
Download figure:
Standard image High-resolution image3.4. HD 140538 (HIP 77052)
HD 140538 (ψ Ser, Figure 2, right panel) is among the stars of Cowley et al. (2020a) which have a significant [El/H] versus Tc plot. Although classified as G2.5 V, it is not considered a solar twin possibly because it is thought to be in a triple system, with two dwarf M companions (Mahdi et al. 2016). The p-parameters for both sets I and II are near unity, indicating solar abundances for the 4 to 5 Earth masses (q parameter) added to HD 140538.
3.5. The Wide Double, HIP 34407 and HIP 34426
In the case of the binary pair, HIP 34407/HIP 34426, abundances are from Ramírez et al. (2019) for 21 elements: Li, O, Na, Mg, Al, Si, S, K, Ca, Sc, Ti, V, Cr, Mn, Fe, Co, Ni, Cu, Zn, Y, and Ba. No GCE corrections were applied in this case, as we assume the stars are coevil. Moreover, if abundances for the two nA elements were perturbed by some isolated nucleosynthetic event, we assume both stars were equally influenced; thus, we include the nA elements Y and Ba in the model fit, based on data set I.
The comoving, closely similar G-stars, HIP 34407 and HIP 34426, were part of a larger work by Nagar et al. (2020), who suggested that the differential abundance pattern could result from the engulfment of a gas giant. The differential abundances are fit by addition of some 7 Earth masses of material, the largest of the modeled stellar masses of this work. This is much lower than the total mass of a Jupiter-sized gas giant, but not so different from estimates of the core mass of heavy elements in Jupiter itself derived from models based on 1995 Galileo probe data (14–18 ME ; Militzer et al. 2008) and more recent results from the Juno mission (7–25 ME ; Wahl et al. 2017). We assume that the more volatile elements forming the bulk of the mass of captured gas giants would be lost during the engulfment process and not incorporated into the SCZ.
The lithium abundance in both stars is much higher than one would expect from their ages, which are greater than 6.5 Gyr for all plausible models according to Ramírez et al. (2019), who give for HIP 34407 and 2.31 for HIP 34426. Figures 2–5 of Carlos et al. (2019) show that only the youngest stars in the 79-star BD18 sample have comparable values with
. Ramírez et al. (2019) discuss lithium abundances in both stars, but find no likely scenario to account for either the high individual abundances or the small abundance difference between the two stars. They compute
this element is clearly above the trend for neighboring elements with similar condensation temperatures in their Figure 4. In our Figure 3, in which the roles of the two stars are reversed and [El/H] is
, lithium would again be an obvious outlier in a plot with [Li/H] = 0.62 at Tc = 1148 K. Li is not included in the current model fit or plot.
Figure 3. Model predictions and observed differential stellar abundances for HIP34407/HIP34426, where [El/H] is . Symbols have the same meaning as in Figure 1. The model is based on data and results of set I.
Download figure:
Standard image High-resolution image4. Summary
In this paper, we have shown that some G-type stellar PDAs can be meaningfully interpreted by assuming that Earth-like material was ingested by or missing from the modeled stars, perhaps through planetary formation processes. Four examples are presented in the form of plots of PDAs versus Tc, three with positive slopes and one with a negative slope. The derived model parameters confirm that the mean composition of the SCZs of these stars is essentially solar (pavg = 1.03 ± 0.20) as expected, but show a range of mass adjustments from −1.4 to +8.2 bulk Earths. Predicted model abundances typically replicate the observed PDA distributions to within the observational errors which have mean values for the elements C through Zn ranging from 0.011 to 0.023 dex, including uncertainties associated with the parameters used to effect GCE corrections. We have tested our approach using two different sets of source data for the condensation temperatures, and the compositions for the bulk Earth material and the initial stellar convection zone and find that, for the 30 elements included in our models, the results from the two sets are in good agreement.
While linear fits like those shown in Figures 1–3 are adequate to describe the current results of PDA investigations which have generally been limited to 30 or so well-studied elements, such models would not properly describe Tc trends if precison abundance data for the full range of naturally occuring elements in the periodic table were available (see the Appendix). We argue that if the full range of abundances were available, the slope in the low-Tc volatile region should be essentially zero, while in the high-Tc refractory regime, the slope should also approach zero, although with much greater scatter.
We thank N. Grevesse for updated solar abundances, references, and evaluation of their accuracies. We also thank W. Clarkson, H. Palme, and our referee B. Fegley for the useful comments. This research has made use of the NASA Exoplanet Archive, which is operated by the California Institute of Technology, under contract with the National Aeronautics and Space Administration under the Exoplanet Exploration Program. We also made use of the SIMBAD database, operated at CDS, Strasbourg, France (Wenger et al. 2000).
Appendix: Condensation Temperatures: Accuracy and Relevance
The relevance of condensation temperatures to cosmic solids was established by Wasson & Chou (1974). The 50% condensation temperatures of Lodders (2003), and the more recent values by Wood et al. (2019), are in quite good agreement, apart from a few elements, namely Cl, Pb, As, and Bi, none of which are included in our modeling calculations (see Figure 4). The assumptions underlying the determinations are more relevant. Both calculations begin with a gas of solar composition at a pressure of 10−4 bar, and assume strict chemical equilibrium gas–solid reactions are allowed. A detailed discussion of equilibrium calculations and the geochemical classification of elements is given by Fegley & Schaefer (2010).
Figure 4. Condensation temperatures from Wood et al. (2019) vs. those from Lodders (2003). The highlighted points are for the 18 elements used for the present models. Of those elements, sodium falls slightly above the 50–50 line, while yttrium falls slightly below. The latter element was used only in the model for HIP 34407/34426. Both deviations are small relative to the uncertainties of the overall model calculations presented in Section 3.
Download figure:
Standard image High-resolution imageBarshay & Lewis (1976, p. 83) discuss an alternate approach: "At the opposite extreme from the equilibrium model is the disequilibrium condensation model, which prohibits reactions between gas and already condensed phases, or between two or more condensed phases." Details of their calculations, available in graphic form (see their Figures 4 and 5), show qualitatively similar results. These figures also show that changes in the gas pressure from 10−2 to 10−6 bar do not significantly change the order of condensation. A useful recent discussion is by Li et al. (2020) who give 2% condensation temperatures, also for a solar mix and a pressure of 10−4 bar, based on the GRAINS code of Petaev (2009). In all these calculations, volatile elements remain volatile and refractories refractory.
Figure 5. Left: model calculations for the change in abundances associated with the ingestion of two bulk Earths from WN18 for the 30 elements of the BD18 study. The error bars are from WN18; the largest is for carbon (leftmost point), whose abundance uncertainty is some 83%. Errors for other elements scale proportionally; the vertical scale does not apply to the errors. Right: same as the left panel, except plotted for all species in WN18. Points for elements whose solar and/or bulk Earth (WN18) abundances are uncertain by ≥0.08 dex are designated by small open circles. A point for lithium is not plotted. The line is a simple trial and error fit of
Download figure:
Standard image High-resolution imageTable 2 shows three estimates of the bulk Earth abundance for representative highly volatile, intermediate volatile, and refractory elements. Horizontal lines divide the three categories of volatility. Abundances are in parts per million by mass, from McDonough (2003, Mc03), McDonough & Arvelo (2008, Mc08), and WN18. The fifth column gives WN18's estimate of their errors in percent. The condensation temperatures are from Wood et al. (2019). The final column is a measure of the element's volatility (VM). It gives the ratio
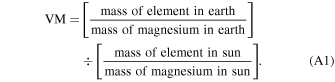
Table 2 illustrates the persistance of the property of volatility for different choices of Earth models. Note that oxygen has an abundance that would put it with the involatiles, in spite of its low Tc. This, of course, is because of the high reactivity of oxygen, so that it combines to form the ubiquitous silicates.
Table 2. Bulk Earth: Representative Element Abundance Estimates
Element | Mc03 | Mc08 | WN18 | % Error | 50% Tc(K) | VM |
---|---|---|---|---|---|---|
C | 730 | 730 | 2 648 | 84 | 40 | 0.00469 |
N | 25 | 57 | 31 | 87 | 123 | 0.000196 |
O | 297 000 | 297 000 | 308 000 | 2.3 | 183 | 0.229 |
Zn | 40 | 35 | 45.9 | 8.7 | 704 | 0.113 |
S | 6 350 | 6 300 | 6 096 | 24 | 672 | 0.084 |
Cu | 60 | 60 | 69 | 2.8 | 1034 | 0.420 |
Na | 1 800 | 1 800 | 2 201 | 8.5 | 1035 | 0.345 |
Fe | 320 000 | 319 000 | 312 000 | 0.3 | 1338 | 108 |
Mg | 154 000 | 153 000 | 151 000 | 1.3 | 1343 | 1.00 |
Download table as: ASCIITypeset image
We conclude that statistically significant fits of [El/H] data as a function of 50% Tc, whether strictly linear, piece-wise linear, or logarithmic (see Wang et al. 2019), are robust indicators of the condensation of cosmic material, Earth-like planets, or meteorites. The slopes of the relevant relations may be positive, zero, or negative.
A.1. Insights from the Full Abundance Table
In Figure 5 (left panel), we plot the change in abundance values for the 30 elements studied in BD18 for an additional two Earth masses to a solar convection zone. Many stellar studies consider even fewer elements, and include only one or a few neutron-addition elements (e.g., Liu et al. 2020). It is difficult to justify a more elaborate fit than a linear one, because of the scatter and sparseness of points especially at low Tc. Workers typically attempt to fit the points with a straight line or line segments (see Liu et al. 2020; Nissen et al. 2020).
Figure 5 (right panel) shows all elements from WN18 apart from lithium, which would be a high outlier at Tc = 1148 K. It shows a much more detailed picture than Figure 5 (left panel). Using the full data from the WN18, we can explore the hypothesis of planet (or interplanetary debris) addition in greater detail when more stellar data points become available.
Elements with Tc <∼ 500K are generally depleted and the logarithm of (1 + BE/SCZ) is essentially zero. The slope at low Tc would become appreciable only when some 20 bulk Earth masses or more are added. If the peculiar abundance revealed by PDAs is due to the ingestion of only one or a few Earth-like masses, the slope at small Tc should be close to zero.
Oxygen with Tc = 40 K stands out above the trend for nearby elements in Figure 5 (see Table 2) because much of it is incorporated in refractory silicate minerals. That explanation is not relevant for Pb (Tc = 727, 495 K), Ag (Tc = 996, 699 K), Au (Tc = 1060, 967 K), and Mn (Tc = 1158, 1123 K) (the first value is from Lodders (2003), the second from Wood et al. (2019). These points follow the overall trend as high outliers.
The plot for elements with Tc > ∼ 1300K shows a wide scatter but little evidence of a meaningful trend with Tc. Some insight may be gained from plots similar to Figure 5 (right panel), but with compositions taken from meteorites (see Cowley et al. 2021). A recent discussion of these questions is given by Fegley et al. (2020), who show that much of the scatter at high Tc arises because of systematic abundance differences between refractory lithophile and siderophile elements (see their Figures 3–5).
Footnotes
- 4
- 5
see Table 1 only in arXiv:2003.14336.