Highlights
A bioinspired strategy is proposed to construct slippery hydrogel with desiccation-tolerant 'skin'.
The problems of dehydration, collapse, and deformation of hydrogel materials are solved.
Lubrication properties of slippery hydrogel are improved by harnessing natural moisturizing factors.
High-precision manufacturing of hydrogels with complicated geometries and customizable microstructures is realized.
Abstract
Hydrogels inevitably undergo dehydration, structural collapse, and shrinkage deformation due to the uninterrupted evaporation in the atmosphere, thereby losing their flexibility, slipperiness, and manufacturing precision. Here, we propose a novel bioinspired strategy to construct a spontaneously formed 'skin' on the slippery hydrogels by incorporating biological stress metabolites trehalose into the hydrogel network, which can generate robust hydrogen bonding interactions to restrain water evaporation. The contents of trehalose in hydrogel matrix can also regulate the desiccation-tolerance, mechanical properties, and lubricating performance of slippery hydrogels in a wide range. Combining vat photopolymerization three-dimensional printing and trehalose-modified slippery hydrogels enables to achieve the structural hydrogels with high resolution, shape fidelity, and sophisticated architectures, instead of structural collapse and shrinkage deformation caused by dehydration. And thus, this proposed functional hydrogel adapts to manufacture large-scale hydrogels with sophisticated architectures in a long-term process. As a proof-of-concept demonstration, a high-precision and sophisticated slippery hydrogel vascular phantom was easily fabricated to imitate guidewire intervention. Additionally, the proposed protocol is universally applicable to diverse types of hydrogel systems. This strategy opens up a versatile methodology to fabricate dry-resistant slippery hydrogel for functional structures and devices, expanding their high-precision processing and broad applications in the atmosphere.
Export citation and abstract BibTeX RIS

Original content from this work may be used under the terms of the Creative Commons Attribution 4.0 license. Any further distribution of this work must maintain attribution to the author(s) and the title of the work, journal citation and DOI.
1. Introduction
Hydrogel is a kind of soft material with hydrophilic three-dimensional (3D) cross-linked network structure, which has been extensively applied in tissue engineering [1], wound dressings [2], flexible wearable devices [3, 4], electronic skin [5], and soft robots [6] due to their flexibility, mechanical tunability, biocompatibility, lubricity and so on. Nevertheless, traditional hydrogels exposed in atmosphere will inescapably arise the dehydration due to water evaporation, resulting in losing their flexibility, slipperiness, and adaptability in long-term applications [7]. Besides, to expand practical application in various fields, vat photopolymerization 3D printing has been widely used to manufacture hydrogel devices with desirable structures [8–13]. It remains challenging to achieve hydrogel stereoscopic construction with large surface area and size in air, which can give rise to uncertainty and distortion of the printed structures due to desiccation [14–16]. To address these shortcomings, approaches such as hygroscopic salts and binary water–organic mixed solvents have been introduced into the bulk hydrogels, restraining the water evaporation under ambient conditions by raising the enthalpy of vaporization of water [4, 17, 18]. Despite improved dehydration tolerance, the hydrogels constructed by these means are inevitably liable to alter in properties such as wettability, lubricity, mechanics, and even hydration. As a consequence, a fundamental challenge is how to prepare slippery hydrogels with outstanding dehydration tolerance together with mechanical performance and adaptation for processing.
In nature, numerous organisms, spanning bacteria, fungi, algae, yeast, tardigrades, insects, and some desert plants, demonstrate remarkable survival capabilities in extreme conditions such as subzero and desiccation [19–24]. Some evidence revealed that trehalose, functioning as a biological stress metabolite and interacting with water and proteins, can form a carbohydrate vitreous structure by hydrogen bonding on the surface of living cells, based on the 'glassy' hypothesis [25–29]. As a result, trehalose-induced glassy-like layer shows a low diffusion coefficient and weak molecular movement, which is beneficial to desiccation resistance, osmoprotection, and heat and cold tolerance [30, 31]. And thus, trehalose can also act as an effective water retention agent for hydrogels by introducing strong hydrogen bonding interactions to remain inherent properties in the atmosphere [32].
Given the biological structure of human skin for the 'water retention', herein we report a novel bioinspired strategy that introduces trehalose into the hydrogel network to form trehalose-induced hydrogen bond interactions. The interaction between trehalose and water can generate the surface layer with dehydration tolerance in atmosphere, which results in the flexible and slippery hydrogels. The mechanical properties and lubrication properties of trehalose-modified slippery hydrogel can also be regulated by the content of trehalose. Combining vat photopolymerization 3D printing and trehalose-modified hydrogels can achieve the miscellaneous stereoscopic hydrogels with desirable resolution, complicated geometries, and tailorable microarchitectures at the macroscopic level due to overcoming the desiccation induced shrinkage and collapse deformation in manufacturing process. As a proof-of-concept demonstration, a high-precision hydrogel vascular phantom was created to imitate guidewire intervention. The approach is also applicable to diverse types of conventional hydrogel systems to create 3D hydrogel architectures. It is believed that the proposed technique provides universal means and new insights for fabricating structured slippery hydrogels with extreme tolerance, high precision, and intricate geometries.
2. Materials and methods
2.1. Materials
Acrylamide (AAm, 98%) was purchased from Tianjin Kemiou Chemical Reagent Co, Ltd. D-(+)-Trehalose dihydrate (99%), phosphate buffered saline (PBS) buffer (pH = 7.4), poly(ethylene glycol)diacrylate (PEGDA, MW: 700), and 2-acrylamido-2-methylpropane sulfonic acid (AMPS, 98%) were purchased from Shanghai Macklin Biochemical Co, Ltd. Tartrazine (95%) was obtained from Shanghai Aladdin Biochemical Technology Co, Ltd. 4-Methoxyphenol (MEHQ, 99.14%) was purchased from Bide Pharmatech Co, Ltd. N, N'-methylenebis-acrylamide (MBAA, 99%) and lithium phenyl-2,4,6-trimethylbenzoylphosphinate (LAP, 95%) were purchased from Sigma-Aldrich. 1-Vinylimidazole (NVP, 99%) was purchased from Energy Chemical. Acrylic acid (99%) was obtained from TCI Development Co, Ltd. Hyaluronic acid methacryloyl (HAMA) and gelatin methacryloyl (GelMA) were purchased from Engineering For Life Company (Suzhou, China). Sterile simulated body fluid (SBF, 1.5×) was purchased from Yuanye Biotechnology Co, Ltd. Simulated blood was purchased from Solarbio Science & Technology Co, Ltd. Medical guidewire (Radifocus GA35153M, Terumo, Japan) was purchased from Qi Sheng Medical Equipment Co, Ltd.
2.2. Synthesis of hydrogel photosensitive inks
Polyacrylamide (PAAm) hydrogel photosensitive ink: firstly, different masses of trehalose were dissolved in DI water to prepare trehalose aqueous solutions with concentrations of 10 wt%, 20 wt%, and 30 wt%, respectively. And then, 21.3 g of AAm, 0.05 g of MBAA, and 0.11 g of LAP were dissolved into 100.0 ml of trehalose aqueous solution and deionized (DI) water, respectively. The hydrogel photosensitive inks were then prepared by adding 1.0 ml of tartrazine solution at a concentration of 50.0 g l−1 and 3.0 ml of MEHQ solution at a concentration of 1.0 g l−1.
The preparation procedures of other hydrogel photosensitive inks are illustrated in the supporting information.
2.3. Construction of anti-dehydration structured slippery hydrogel
A commercial digital light processing 3D printer (CREALITY, Shenzhen, China) with SF-01 light source (SHENGFENG TECH Co, Ltd, Shenzhen, China) is adopted to build an independently developed vat photopolymerization 3D printer. The above-prepared hydrogel photosensitive ink was poured into the self-built vat photopolymerization printer tank, and the 3D digital model was then sliced into stereolithography files by open-source slicing and control software (Creation Workshop V5.0) for the 3D printing process. During printing, ultraviolet source was employed to trigger radical polymerization to obtain 3D objects. The 3D printing parameters were set as follows: the thickness of the single layer was fixed at 100 μm, the photo-curing time was set to 10 s, and the experimental platform temperature was controlled at room temperature.
2.4. Structural and morphological characterization
Attenuated total reflection-Fourier transform infrared spectroscopy (FT-IR, Nicolet iS50, Thermo Fisher Scientific, USA) was utilized to identify the characteristic functional groups of hydrogels. X-ray photoelectron spectroscopy (XPS, ESCALAB 250Xi, Thermo Fisher Scientific, USA) with an Al Kα radiation source was employed to characterize the structural features of hydrogels. And all spectra were calibrated with the binding energy of the C1s peak at 284.8 eV. The microstructure of hydrogels after dehydration was characterized by scanning electron microscopy (SEM, FEI Quanta 650 FEG) under an accelerating voltage of 30 kV. Before SEM appearance observation, all hydrogels were lyophilized for 48 h, and then a thin layer of gold was sputtered on the surface by ion sputtering device (GVC-1000). Laser scanning confocal microscopy (Olympus FV1200, Japan) was used to observe the construction precision and collapse deformation of anti-dehydration slippery structured hydrogels after printing.
2.5. Characterization of mechanical properties
The mechanical properties of hydrogels were carried out with a universal material testing machine (EZ-Test, SHIMADZU, Japan) with a 500 N load cell. Prior to testing, the hydrogel tensile specimen was cut into rectangular specimens with a length of 40 mm, a width of 8 mm, and a thickness of 1 mm, and the specimen was stretched at a tensile speed of 100 mm min−1 until the sample fracture. Breaking strain (b, %) is defined as deformation relative to the initial length of the undeformed specimen. Fracture strength (σb, MPa) is the maximum tensile strength obtained by dividing the maximum bearing capacity by the cross-sectional area of the undeformed specimen. Young's modulus of hydrogel is defined as the slope of the stress–strain curve in the linear elastic region between 5% and 15% strain. Besides, the toughness of the hydrogel (Γ, MJ m−3) is the integral area of the region included in the stress–strain curve:
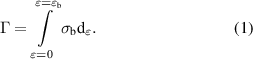
Among them, σb and b are the corresponding stresses and strains when the hydrogel fractures, respectively.
2.6. Characterization of dehydration and dimensional shrinkage properties
The water evaporation rate (V) of hydrogels at different time intervals is defined as the ratio of the mass of evaporable water to the initial water content of the hydrogel, which is calculated as:

where m0 and mt are the water content of the hydrogel in its initial state and the mass of the evaporated water at different evaporation times, respectively. Three parallel hydrogel samples were used to test the relative evaporation rate, and the final data are presented as means ± SD (n = 3).
The size shrinkage of a hydrogel can be calculated according to the following expression:

Among them, TA and TB are the thickness of the hydrogel after desiccating and before desiccating, respectively. At least three parallel tests were performed for each hydrogel, and the final data was represented by the mean ± SD (n = 3).
2.7. Rheological characterization
Dynamic shear rheology properties of the hydrogels were assessed on a rotational RS6000 rheometer (HAAKE, Germany), and the tests were performed at 25 °C. A hydrogel sample was set under a 35 mm diameter parallel plate with a sample gap of 1.0–1.5 mm. Storage modulus (G') and loss modulus (G'') of the anti-dehydration slippery hydrogels were measured on frequency sweep in a range of 0.1–100 Hz at a fixed stress of 2000 Pa. Amplitude sweep was performed from 1 Pa to 20 000 Pa stress at a fixed frequency of 1 Hz to measure dynamic G' and G''. Dynamic G' and G'' of time sweep were performed under a stress of 2000 Pa. The temperature dependence of the dynamic G' and G'' was measured at a fixed frequency of 1 Hz and a fixed stress amplitude of 2000 Pa in a temperature range of −10 °C–80 °C. Loss factor (tan δ) of the anti-dehydration slippery hydrogel was defined as G''/G'.
2.8. Tribological characterization
A ball-on-disk reciprocating tribometer (CSM, Switzerland) was utilized to evaluate the frictional properties of the anti-dehydration slippery hydrogels. A hydrogel slice with a diameter of 35 mm is fixed on tribometer, and DI water, SBF, simulated blood, and PBS buffer as lubricants are dropped onto the surface of the hydrogel. A glass ball with a radius of 3 mm is used as the friction pair. In the sliding friction test, the sliding stroke is 5 mm, the applied loads are 0.2 N, 0.5 N, 0.7 N, 1.0 N and 1.5 N, and the sliding frequencies are 0.2 Hz, 0.5 Hz, 1.0 Hz, 1.5 Hz and 2.0 Hz. After 180 sliding cycles, a stable friction test curve and average coefficient of friction (COF) are ultimately obtained.
3. Results and discussion
3.1. Design and construction of desiccation-tolerant slippery hydrogel
As shown in figure 1(a), human skin can effectively lock in dampness to prevent excessive evaporation by the stratum corneum, and thus possessing favorable dehydration tolerance. Under water-scarce conditions, trehalose is capable of forming vitrified structure on the cell surface to skillfully protect the biomolecular structure without being destroyed. To this end, we introduced trehalose into the hydrogel to construct the anti-dehydration surface layer by hydrogen bonding network. Specifically, the strong covalent-like hydrogen bonding interactions formed by multitudinous hydroxyl groups on the trehalose molecule and numerous polar groups on the long polymer chains can confer superior ductility and desiccation-tolerant of slippery hydrogel (figure 1(b)). As detailed in figure 1(c), AAm, MBAA, LAP, tartrazine, and MEHQ were dissolved into trehalose aqueous solutions with different concentrations to concoct hydrogel photosensitive inks, and then vat photopolymerization 3D printing was utilized to construct sophisticated and high-precision hydrogel architectures. As illustrated in figure 1(d), some structural hydrogels with high-precision, shape fidelity, and alloplasmic structure, like prosthetic nose, liver phantom, and blood vessel network, can be readily built through vat photopolymerization 3D printing of anti-dehydration hydrogel.
Figure 1. Design and construction of desiccation-tolerant slippery hydrogel with sophisticated structures. (a) Human skin structure. (b) Anti-dehydration mechanism of slippery hydrogel with skin layer. (c) Hydrogel photosensitive ink constituents for vat photopolymerization 3D printing. (d) Volumetric soft tissue phantoms constructed by harnessing anti-dehydration slippery hydrogel: prosthetic nose, liver phantom, and biomimetic vascular network.
Download figure:
Standard image High-resolution image3.2. Structural characterization of anti-dehydration slippery hydrogel
The FT-IR spectra of PAAm, trehalose, PAAm/trehalose-10 wt%, PAAm/trehalose-20 wt%, and PAAm/trehalose-30 wt% hydrogels are shown in figures 2(a) and (b). It was seen that the stretching vibration peak of -NH2 in the AAm molecule appears at 3170 cm−1, while the characteristic peak at 1660 cm−1 is ascribed to the stretching vibration of C=O [33]. Furthermore, the characteristic peaks at 1572 cm−1 and 1350 cm−1 are assigned to the bending vibration of N–H and the stretching vibration of C–N, respectively [33, 34]. Compared with PAAm and trehalose, the characteristic peak of trehalose-modified PAAm hydrogel at 989 cm−1 belongs to the C–C stretching vibrations of trehalose molecules, while the characteristic peaks at 1080 cm−1 and 1150 cm−1 correspond to the asymmetric stretching vibrations of C–O–C bridging band and non-bridging modes on trehalose molecules [35]. Meanwhile, the characteristic peak at 1040 cm−1 is related to the C–OH bending vibration of trehalose molecules [35]. Moreover, trehalose-modified PAAm hydrogels cause shifts in the N–H and C=O peaks, which may have formed intermolecular hydrogen bonds between trehalose and PAAm chains.
Figure 2. Structure characterization of PAAm and trehalose-modified PAAm hydrogels. (a) and (b) FT-IR spectra of PAAm, trehalose, PAAm/trehalose-10 wt%, PAAm/trehalose-20 wt%, and PAAm/trehalose-30 wt% hydrogels. (c) XPS survey spectra of PAAm, PAAm/trehalose-10 wt%, PAAm/trehalose-20 wt%, and PAAm/trehalose-30 wt% hydrogels. High-resolution (d) C 1s, (e) O 1s, and (f) N 1s spectra of PAAm, PAAm/trehalose-10 wt%, PAAm/trehalose-20 wt%, and PAAm/trehalose-30 wt% hydrogels.
Download figure:
Standard image High-resolution imageAs shown in figures 2(c)–(f), XPS spectra are further utilized to confirm the structural properties of the anti-dehydration hydrogels. For the high-resolution C 1s spectra of PAAm hydrogel, three peaks appear at 284.6 eV, 285.3 eV, and 288.1 eV, which can be attributed to C–C, C–N, and C=O bonds on the AAm molecule, respectively [34]. For the C 1s spectra of the PAAm/trehalose-10 wt% hydrogel in figure 2(d), a new peak appears at 286.3 eV, which can be attributed to the C–O bond of trehalose molecules in the anti-dehydration hydrogels [33]. As shown in figure 2(d), the peak intensity of C–O bonds is further enhanced with the increase of trehalose content in the hydrogel network. From the high-resolution O 1s spectra (figure 2(e)), it can be found that new C–OH and C–O–C peaks appear after the introduction of trehalose into PAAm hydrogels, and they move toward low binding energy with the increase of trehalose content. Meanwhile, the introduction of trehalose into PAAm hydrogels also causes the -NH2 peak to shift toward low binding energy (figure 2(f)). This result indicates that a strong hydrogen bond interaction is formed between trehalose molecules and PAAm polymer chains in the anti-dehydration hydrogel network.
3.3. Anti-dehydration performance of PAAm and trehalose-modified PAAm hydrogels
Figure 3(a) shows the desiccation performance of PAAm and trehalose-modified PAAm hydrogels in atmosphere. PAAm hydrogel loses water extremely quickly, resulting in the large contraction and breakdown of PAAm hydrogel network. On the contrary, the water in trehalose-modified PAAm hydrogels gradually volatilizes over time. Meanwhile, increasing the content of trehalose can efficaciously decelerate the desiccation behavior of PAAm hydrogels. Trehalose-rich PAAm hydrogel becomes opaque during water evaporation due to the difference in refractive index between the surface glassy layer and internal hydrogel matrix. These results show that trehalose-modified PAAm hydrogels retard the dehydration features of hydrogels due to their strong hydrogen bonding interaction. Furthermore, the water evaporation rate of the hydrogels was estimated by laying aside PAAm and trehalose-modified PAAm hydrogels at room temperature in the atmosphere, as illustrated in figure 3(b). PAAm hydrogel loses about 95% of their water content after 24 h. In contrast, trehalose-copious PAAm hydrogels exhibit a much lower water evaporation rate. After 24 h exposure in air, in particular, the water evaporation of PAAm/trehalose-10 wt%, PAAm/trehalose-20 wt%, and PAAm/trehalose-30 wt% hydrogels are 87%, 77%, and 63% of their initial water content, respectively. As displayed in figure 3(c), the dimension shrinkage rate of PAAm hydrogel is 77% after dehydration, while the dimension shrinkage rate of trehalose-altered PAAm hydrogels diminishes from 72% to 49% with the enlargement of trehalose contents. It further indicates that trehalose-transformed PAAm hydrogel possesses superlative desiccation-tolerant and low-level size shrinkage.
Figure 3. Anti-dehydration performance of PAAm and trehalose-modified PAAm hydrogels. (a) Desiccation circumstances of PAAm and trehalose-modified PAAm hydrogels under different intervals. (b) Water evaporation rate and (c) dimension shrinkage rate of PAAm and trehalose-modified PAAm hydrogels.
Download figure:
Standard image High-resolution imageFigure 4(a) shows the SEM image of a PAAm hydrogel, which can be seen collapsing after its lyophilization because lack of water supports the polymer network, leading to the loose and porous network structure. For trehalose-modified PAAm hydrogels, as illustrated in figures 4(b)–(d), the hydrogel network structure exhibits a partial porous microstructure after lyophilization. And with the increase of trehalose content in the hydrogel network, this loose porous structure becomes fewer and fewer. This substantiates that trehalose can prevent the hydrogel from dehydration during lyophilization, thereby preserving the network structure of the hydrogel from collapse.
Figure 4. SEM images of (a) PAAm, (b) PAAm/trehalose-10 wt%, (c) PAAm/trehalose-20 wt%, and (d) PAAm/trehalose-30 wt% hydrogels after lyophilization.
Download figure:
Standard image High-resolution image3.4. Mechanical properties of anti-dehydration slippery hydrogel
Figure 5(a) illustrates the tensile properties of PAAm/trehalose-30 wt% hydrogel under different tensile strains. The tensile stress–strain curves of PAAm and trehalose-modified PAAm hydrogels at room temperature are displayed in figure 5(b). Compared with pure PAAm hydrogel, the fracture strength and malleability of trehalose-embellished slippery hydrogels are remarkably advanced. As shown in figures 5(c) and (d), the fracture strain of PAAm, PAAm/trehalose-10 wt%, PAAm/trehalose-20 wt%, and PAAm/trehalose-30 wt% hydrogels is (349.1 ± 36.6)%, (412.5 ± 27.3)%, (438.7 ± 51.1)%, and (517.7 ± 11.7)%, respectively, while the tensile strength is (15.5 ± 2.4) kPa and (18.1 ± 3.1) kPa, (24.4 ± 0.5) kPa, and (36.2 ± 5.2) kPa, respectively. Moreover, the fracture toughness and elastic modulus of slippery hydrogel increase from (37.1 ± 7.6) kJ m−3 and (8.2 ± 0.6) kPa to (109.4 ± 9.8) kJ m−3 and (18.9 ± 1.9) kPa, respectively. It was found that the strength, malleability, toughness, and elastic modulus of trehalose-garnished slippery hydrogels rise substantially with the increase of trehalose content. Besides, trehalose-modified slippery hydrogels exhibit admirable flexibility even after 24 h of exposure to air (figure S1). This result shows that trehalose features a tremendously obvious enhancement effect on the fracture toughness and elastic modulus of hydrogels, which may be due to the introduction of trehalose that can form hydrogen bond interactions at multiple bonding points to accomplish supplemental energy dissipation.
Figure 5. Mechanical performance of the anti-dehydration slippery hydrogel. (a) Ductility of the hydrogel under different tensile strains. (b) Tensile stress–strain curves. (c) Ultimate strain and fracture strength. (d) Toughness and Young's modulus.
Download figure:
Standard image High-resolution imageAs shown in figure 6(a), the rotational rheological measurements of the anti-dehydration hydrogels were further carried out to reveal variations in their dynamic mechanical properties and internal network structure. Figure 6(b) shows the storage modulus (G') and loss modulus (G'') of PAAm and trehalose-modified PAAm hydrogels as a function of temperature. Compared with PAAm hydrogel, increasing trehalose is beneficial to decline the transition temperature of PAAm hydrogels (figure S2), which is due to the introduction of trehalose also having certain frost resistance properties. It is worth noting that the introduction of trehalose-induced hydrogen bond interaction leads to temperature-independent behavior of slippery hydrogels, suggesting that trehalose can ameliorate its dehydration properties. Furthermore, the loss factor (tan δ) reflects the overall energy dissipation during dynamic shearing deformation [36]. As illustrated in figure 6(c), tan δ gradually decreases with the increasing temperature variable, which indicates the transition from viscous to elastic behavior in slippery hydrogels [37]. This is because higher temperatures weaken the dynamic hydrogen bond network, resulting in slightly diminishing the physical crosslinking density. As depicted in figure 6(d), time-dependent G' and G'' of the anti-dehydration slippery hydrogels are also performed to characterize dynamic mechanical properties. Aside from the time sweep test, the amplitude sweep test is also performed to investigate the non-linear viscoelastic behavior of the anti-dehydration hydrogels (figure 6(e)). As the shear stress continues to increase, however, the G' of the hydrogel begins to decrease, while G'' shows an increasing tendency. This manifests that the viscous deformation moderately intensifies with the rise of shear stress, which ultimately leads to the destruction of the internal network structure of the hydrogel. The frequency dependence of G' and G'' for the anti-dehydration hydrogels with variable trehalose is shown in figure 6(f). For all the hydrogels, G' is much larger than G'' over the whole range of frequencies measured, indicating a solid-like and elastic nature of the anti-dehydration hydrogels [38]. Actually, both G' and G'' enhances with increasing trehalose content in the anti-hydration slippery hydrogels, indicating that the hydrogen bonding interactions between trehalose molecule and the polar groups on polymer side chains may cause the contraction of polymer segments. In brief, the introduction of hydrogen bonding network based on trehalose not only significantly improves the physical crosslinking density and viscoelastic modulus of the hydrogel, but also dramatically ameliorates their energy dissipation efficiency.
Figure 6. Rheological properties of the anti-dehydration slippery hydrogel. (a) Schematic illustration of rheological testing of the hydrogels. (b) Storage modulus (G') and loss modulus (G'') of the hydrogels varied with temperature. (c) Tan δ of slippery hydrogels as a function of temperature. (d) G' and G'' of slippery hydrogels creep over time at room temperature. (e) Amplitude sweeps test of slippery hydrogel under stress from 1 Pa to 20 000 Pa. (f) Dynamic G' and G'' of slippery hydrogels as a function of frequency.
Download figure:
Standard image High-resolution image3.5. Lubrication performance of anti-dehydration slippery hydrogel
As shown in figure 7(a), a ball-disk sliding contact friction testing machine is exerted to evaluate the tribological properties of the anti-dehydration slippery hydrogels. The effect of trehalose content on the lubricating properties of slippery hydrogel is shown in figures 7(b) and (c). It can be found that anti-dehydration slippery hydrogel with higher trehalose content possesses a relatively low friction coefficient. This is because trehalose, which is highly absorbent, can effectively absorb water molecules to improve hydration on the surface of the hydrogel and reduce its friction coefficient. As illustrated in figures 7(d) and (e), the COF of slippery hydrogel gradually rises from 0.0541 to 0.3367 when the applied load expands from 0.2 N to 1.5 N. This difference in lubrication properties is basically due to the effect of the applied load on the contact deformation and viscoelastic resistance of hydrogel. Henceforth, the influence of sliding frequency on the lubrication properties of slippery hydrogel is shown in figures 7(f) and (g), which displays that a large sliding frequency is not conducive to achieving a low COF for slippery hydrogel. This is attributed to the fact that less lubricant penetrates the friction interface at a larger sliding frequency and that the hydration lubricant layer weakens during reciprocating friction. The influence of water lubricants, involving PBS buffer, DI water, simulated blood, and SBF, was systematically investigated. As shown in figures 7(h) and (i), the friction coefficients of slippery hydrogel under SBF, PBS, simulated blood, and DI water are 0.0569, 0.0566, 0.0623, and 0.0732, respectively. By comparison, slippery hydrogel in PBS and SBF water lubricated medium exhibited better lubricating properties. To this end, this structured hydrogel with moistening features and lubricating properties enhanced by hydrogen bond network exhibits broad application prospects in the field of biolubrication.
Figure 7. Lubrication performance of the anti-dehydration slippery hydrogel. (a) Schematic illustration of friction test of slippery hydrogel. (b) and (c) Effect of trehalose content on the lubricating properties of hydrogels. (d) and (e) Effect of applied load on the lubricating properties of hydrogels. (f) and (g) Effect of sliding frequency on the lubricating properties of hydrogels. (h) and (i) Effect of water lubricant on the lubricating properties of hydrogels.
Download figure:
Standard image High-resolution image3.6. High-precision structural construction of anti-dehydration slippery hydrogel
Hydrogels have multifarious limitations in terms of structural design and manufacturing accuracy, such as the destruction of print quality, fidelity, and precision because of their dehydration characteristics in atmosphere [39]. As shown in figure 8(a), a tower model was applied to investigate the dehydration, printing accuracy, and structural integrity of trehalose-converted PAAm hydrogel in compared with pure PAAm ones for a long-term printing at room temperature. As revealed in figure 8(b), the structured hydrogel constructed by PAAm hydrogel has collapsed and deformed due to its elementary dehydration, resulting in inferior manufacturing precision. The hydrogel structure constructed by trehalose-modified PAAm hydrogels feature sophisticated printing accuracy, and the manufacturing precision is higher and higher with the increase of trehalose content (figures 8(c)–(e)). Optical microscopic images also further verify that high-precision structured hydrogels are composed of layers of structures stacked on top of each other. Meanwhile, trehalose-modified slippery hydrogels can also manufacture high-precision microneedle arrays, and the high trehalose content will be conducive to improving the accuracy of hydrogel microneedles (figure S3). This high-precision shaping fundamental may be related to two inimitable protective mechanisms of trehalose: (1) hydrogen bonding interaction between trehalose molecules and PAAm polymer chains (water replacement) and (2) the capture of water molecules closes to hydrogel surfaces (water layer) [40, 41]. Consequently, this tactic can tackle the issues of dehydration deformation and printing accuracy of large-size hydrogel structures for a long-term manufacturing process in atmosphere.
Figure 8. High-precision construction of the anti-dehydration slippery hydrogel by harnessing vat photopolymerization 3D printing. (a) 3D model of tower. Photographs of tower and corresponding optical microscopic images fabricated with (b) PAAm, (c) PAAm/trehalose-10 wt%, (d) PAAm/trehalose-20 wt%, and (e) PAAm/trehalose-30 wt% hydrogels, respectively.
Download figure:
Standard image High-resolution imageDue to their slippery properties similar to natural vascular tissue, hydrogels are extensively used to engineer vascular network phantom [42]. Nevertheless, achieving hydrogel based fine geometry design and interconnected hollow channel is extremely challenging. Vat photopolymerization 3D printing provides the opportunity to construct the multi-bifurcation structures and interconnected microscale vascular networks. As illustrated in figures 9(a)–(d), several types of sophisticated vascular networks were constructed with anti-dehydration slippery hydrogels, including portal vein vascular network (figure 9(a)), arterial vascular network (figure 9(b)), arteria hepatica vascular network (figure 9(c)), and pulmonary artery vascular network (figure 9(d)). It can be seen that these slippery hydrogel vascular networks feature the characteristics of highly bifurcated network structure, independent and narrow vasculature networks. As a paradigm, slippery hydrogel vascular replicates served as training platforms to imitate endovascular interventions (figure 9(e)). The guidewire is manually rotated at the intersection of slippery hydrogel vascular so that it enters the exact branch of the vascular network. Manually rotate the guidewire at the intersection of slippery hydrogel vascular so that it enters the exact branch of the vascular network. When the guidewire enters the vascular network for a distance, it can be seen that the guidewire can be freely traction to the end in multiple vascular channels. Meanwhile, in vitro biocompatibility evaluation of PAAm and trehalose-modified PAAm hydrogels showed excellent cell proliferative activity, and hydrogels with high trehalose content are more conducive to cell proliferation (figure S4). It indicates that the as-prepared hydrogel vascular network not only possesses a smooth inner wall, but also exhibits the exceptional lubrication and biocompatibility, showing the potential in slippery hydrogel based biomimetic vascular phantoms for vascular interventional therapy.
Figure 9. Design and fabrication of slippery hydrogel multiscale vascular network. (a) Portal vein, (b) arterial, (c) arteria hepatica, and (d) pulmonary artery vascular networks. (e) Demonstration of guidewire navigation within a slippery hydrogel vascular network.
Download figure:
Standard image High-resolution image3.7. Versatility of trehalose for miscellaneous hydrogel systems
To confirm the universality of trehalose for preparing desiccation tolerant hydrogel systems, we extend the means to trehalose-modified P(AAm-co-AA) hydrogel, P(AAm-co-AMPS) hydrogel, and P(AAm-co-NVP) hydrogel systems. As shown in figure 10(a), the Wolpertinger was used as the printing model (figure 10(c)) to verify the water loss resistance and printing accuracy of P(AAm-co-AA) and trehalose-modified P(AAm-co-AA) hydrogels. After 20 h exposure to a room temperature atmosphere, the water evaporation of P(AAm-co-AA) and trehalose-modified P(AAm-co-AA) hydrogels is 88% and 69% of their initial water content, respectively (figure 10(b)). Furthermore, as shown in figures 10(d) and (e), the water evaporation of P(AAm-co-AMPS) and trehalose-modified P(AAm-co-AMPS) hydrogels is 100% and 82% of their initial water content, respectively, and then utilizing Great Wild Goose Pagoda as the printing model to investigate the structural precision of the hydrogel in air after printing. As illustrated in figures 10(g) and (h), compared with P(AAm-co-NVP) hydrogel, trehalose-modified P(AAm-co-NVP) hydrogel can significantly improve their resistance to dehydration, and the desiccation and shrinkage of the hydrogel structure after printing were observed using the Tristar Piles Bronze Mask (figure 10(i)) as the printing model. Intuitively compared with pure hydrogels, 3D hydrogel structures constructed by trehalose-modified P(AAm-co-AA), P(AAm-co-AMPS), and P(AAm-co-NVP) hydrogels feature high printing accuracy and shape fidelity. The structural hydrogel is constructed by trehalose-modified slippery hydrogel, which still possesses acceptable environmental preserve performance. Meanwhile, the strategy is capable of extending to degradable hydrogel systems, such as PEGDA-co-GelMA and PEGDA-co-HAMA hydrogels, to achieve high-precision manufacturing of complicated architectures (figure S5). Accordingly, the improvement strategy based on trehalose-induced hydrogen bond network exhibits favorable universality, which provides a novel approach for high-precision manufacturing and resistance to dehydration of complex structured hydrogels.
Figure 10. Manufacturing capability of trehalose-modified slippery hydrogels in dissimilar hydrogel systems. (a) and (b) Photosensitive ink components and dehydration properties of trehalose-modified and unmodified P(AAm-co-AA) hydrogels. (c) Photographs of the Wolpertinger printed with trehalose-modified and unmodified P(AAm-co-AA) hydrogels. (d) and (e) Photosensitive ink components and desiccation properties of trehalose-modified and unmodified P(AAm-co-AMPS) hydrogels. (f) Photographs of the Great Wild Goose Pagoda printed with trehalose-modified and unmodified P(AAm-co-AMPS) hydrogels. (g) and (h) Photosensitive ink components and dehydration properties of trehalose-modified and unmodified P(AAm-co-NVP) hydrogels. (i) Photographs of the Tristar Piles Bronze Mask printed with trehalose-modified and unmodified P(AAm-co-NVP) hydrogels.
Download figure:
Standard image High-resolution image4. Conclusions
In conclusion, we develop a novel bioinspired strategy for the construction of a spontaneously formed 'skin' on the slippery hydrogels in atmosphere by incorporating biological stress metabolites trehalose into the hydrogel matrix. The introduction of trehalose into the hydrogel network can serve as the network-strengthened agent and anti-dehydration agent by harnessing the hydrogen bond interactions formed between trehalose, water, and polymer chains. As a result, the mechanical and lubrication performance of trehalose-modified slippery hydrogels improves significantly with the increase of trehalose contents. Furthermore, as introducing trehalose into the photosensitive hydrogel ink, a variety of slippery structured hydrogels with high precision, shape fidelity, and complicated architectures are easily manufactured at the macroscopic scale by taking the advantage of desiccation tolerance, which effectively avoids structural collapse and shrinkage deformation in atmosphere. This convenient and versatile strategy is also extensively applicable to various commonly utilized hydrogels system for high-precision additive manufacturing. Most significantly, this protocol can be used to prepare the slippery hydrogel-based vascular phantom for in vitro guidewire intervention simulation. It is believed that the proposed method paves the way toward manufacturing the large-scale structural hydrogels with dehydration tolerance in atmosphere, broadening their applications in complex environments.
Acknowledgments
The authors are grateful for the financial support from the National Key Research and Development Program of China (2022YFB4600101), the National Natural Science Foundation of China (52175201, 52005484, and 52205228), the Research Program of Science and Technology Department of Gansu Province (21YF5FA139 and 22JR5RA107), the Shandong Provincial Natural Science Foundation (ZR2023OE090), the Major Program (ZYFZFX-2) and the Cooperation Foundation for Young Scholars (HZJJ23-02) of the Lanzhou Institute of Chemical Physics, CAS, the Western Light Project, CAS (xbzg-zdsys-202007), the Taishan Scholars Program, and the Oasis Scholar of Shihezi University.
Conflict of interest
The authors declare that they have no known competing financial interests or personal relationships that could have appeared to influence the work reported in this paper.
Supplementary data (0.9 MB PDF)