Abstract
Screening of high-Z (W) impurities from the confined plasma by the temperature gradient at the plasma periphery of fusion-grade H-mode plasmas has been demonstrated in the JET-ILW (ITER-like wall) tokamak. Through careful optimisation of the hybrid-scenario, deuterium plasmas with sufficient heating power ( 32 MW), high enough ion temperature gradients at the H-mode pedestal top can be achieved for the collisional, neo-classical convection of the W impurities to be directed outwards, expelling them from the confined plasma. Measurements of the W impurity fluxes between and during edge-localised modes (ELMs) based on fast bolometry measurements show that in such plasmas there is a net efflux (loss) between ELMs but that ELMs often allow some W back into the confined plasma. Provided steady, high-power heating is maintained, this mechanism allows such plasmas to sustain high performance, with an average D–D neutron rate of
s−1 over a period of ∼3 s, after an initial overshoot (equivalent to a D–T fusion power of ∼9.4 MW), without an uncontrolled rise in W impurity radiation, giving added confidence that impurity screening by the pedestal may also occur in ITER, as has previously been predicted (Dux et al 2017 Nucl. Mater. Energy 12 28–35).
Export citation and abstract BibTeX RIS

Original content from this work may be used under the terms of the Creative Commons Attribution 4.0 license. Any further distribution of this work must maintain attribution to the author(s) and the title of the work, journal citation and DOI.
1. Introduction
The JET-ILW tokamak [1] has an all-metal (Be/W) first-wall, as is to be used in the ITER device [2], both to overcome the problem of tritium retention in graphite plasma facing materials (PFMs) and to handle the high stationary heat loads to the divertor targets [3]. A disadvantage with using high-Z (74) tungsten W as a target material is that W is not fully ionized, even in the plasma core at temperatures required for a burning D–T plasma (10 keV) [4], as currently achieved in JET-ILW and expected in ITER. Such partially ionized W ions are able to radiate strongly [5] (
more than other PFMs), hence cooling the core plasma and potentially leading to plasma disruptions. Furthermore, calculations show that for a W concentration of
, ignition of a fusion reactor is not possible [6].
Predictions for ITER have suggested that W contamination of the confined plasma can be avoided if the ion temperature at the top of the edge transport barrier (ETB), which forms the H-mode 'pedestal', is sufficiently high [7]. This so-called 'temperature-gradient screening' occurs when the ion temperature gradient is sufficiently strong compared to the ion density gradient
[8, 9], causing outward impurity convection to expel the impurities from the confined plasma.
Recent experiments on JET-ILW have demonstrated impurity screening by the temperature gradient at the pedestal for the first time in carefully optimised 'hybrid'-scenario H-mode deuterium (D) plasmas with sufficiently high, sustained heating power ( MW) [10]. Such pulses can achieve a D–D neutron rate
n s−1, which is an average over the period 8.5–11.5 s, after an initial overshoot (equivalent to a fusion power of (
MW for a 50:50 D–T fuel mix) [11], without an uncontrolled rise in W impurity radiation, giving confidence that this impurity mitigation mechanism may occur in ITER.
Screening of W impurities from the core plasma also occurs in ITER-baseline scenario plasmas in JET-ILW, which operate at higher plasma current and safety factor q than hybrid scenario plasmas. In baseline plasmas, outward neo-classical (NC) convection localises the W impurities just inside the pedestal top, where they can be efficiently flushed by edge-localised modes (ELMs) [12]. Maintaining good confinement with control of W radiation requires moderate D2 gas puffing and ELM pacing pellets to avoid disruptions induced by excess edge radiation [13]. ITER-baseline plasmas run at low or zero rates of D2 gas fuelling, exhibiting small, high-frequency ELMs, also exhibit temperature-gradient screening at the pedestal top, which is enhanced by a hot, low collisionality pedestal and with Ne impurity seeding the level of W radiation can be controlled [14].
Here, we show how the initial D2 gas fuelling during the early phase of hybrid-scenario pulses can be optimised to produce a hot, low-collisionality, strongly rotating plasma at the pedestal top, which screens the W impurities from the confined plasma. Recent, improved understanding of collisional, NC impurity transport [15–17] based on interpretation of drift-kinetic calculation results from the NEO code [18–20], has shown the temperature gradient screening to be enhanced at low collisionality and by strong toroidal flow (Mach numbers , where
is the toroidal rotation rate,
is the ion thermal velocity,
and
for the main
ions)—conditions favoured by the initially low gas fuelling rate.
Data from analysis of fast, bolometric total radiation measurements [21] is used to measure changes in the relative W content of the core plasma occurring between and during ELMs, thereby quantifying the W fluences (i.e. time-integrated fluxes) due to impurity transport across the pedestal between and during ELMs. These measurements clearly show that the optimised, hot pedestal reduces and, on-average, reverses the flux of W crossing the pedestal between ELMs. These results are consistent with the presence of impurity screening from the confined plasma in these plasmas, in contrast to the case in ITER-baseline plasmas in JET-ILW, which rely on impurity flushing by ELMs to control the impurity radiation [12].
A less desirable consequence of the resulting low W density in the outer, 'mantle' region of the optimised plasma is that the ELMs no longer expel or 'flush' W but on-average, allow the W to re-enter the plasma from the surrounding scrape-off-layer (SOL). Such a reversal of ELM flushing of impurities, resulting from hollowing of the W impurity density profile across the pedestal by NC temperature-gradient screening, is predicted in [22] by means of modelling using a full-orbit particle extension to the non-linear MHD code JOREK [23].
The remainder of the paper is structured as follows: in section 2, parameters of the two hybrid scenario pulses are presented and key features of this operational scenario discussed. Details of how the startup phase of the pulse is optimised to achieve a hot pedestal are presented in section 2.1 and general features of the total radiation distribution in these pulses are discussed in section 2.2. The main results of experimental analysis and interpretive modelling are presented in the rather extensive section 3, which are first previewed in its introduction. Finally in section 4, the available evidence for peripheral W screening is summarised in section 4.1 and implications for ITER operation discussed in section 4.2.
2. Hybrid-scenario pulses
Two different ELMy H-mode scenarios are under preparation for high-power D–T operation in both JET-ILW [24] and ITER: the ITER-baseline scenario operating at a normalised plasma pressure ∼ 1.8–2
5
and low edge safety factor
6
and the hybrid scenario with a lower ratio of plasma current
to toroidal field
and hence higher
∼ 2–3 and high
[25]. Hence, hybrid pulses operate with central q0 at or just above 1, while baseline pulses have
and hence exhibit saw-teeth instabilities of the plasma core. Another key difference in present experiments, is that the high
of baseline pulses favours a higher density at the H-mode pedestal top
[26] and hence a flatter, less peaked density profile than typical of hybrid pulses, in which the low-density pedestal is hotter and less collisional.
2.1. Startup optimisation
The evolution of two high-power ∼ 32–35 MW hybrid pulses, with
(q95 ∼ 4.8–5.0) is compared in figure 1. The initial
ramp and timing of the heating power is tailored to produce a broad, flat q profile with
, with the aim of avoiding deleterious MHD instabilities and allowing access to high
[29].
Figure 1. Comparison of the evolution of hybrid-scenario pulses #96501 and #97781 showing: (a) the total input power ; (b) the requested D2 gas fuelling rate waveform
(does not include delays due to finite valve opening and gas transmission times or valve hysteresis effects); (c) the line-average density
; (d) the ion temperature at the density pedestal top
measured by CXRS [27] (solid) and the central ion temperature
measured by high-resolution spectroscopy of Ni+26 ions [28] (dashed); (e) the radiated power
and radiated power fraction
(dashed) from the confined plasma; (f) the ELM behaviour from a visible Be II line intensity measured viewing the outer divertor target; (g) the D–D neutron rate
; and (h) the major radius of the outer strike point
.
Download figure:
Standard image High-resolution imageIn the 2.2 MA pulse #96501, an initially high level of D2 gas fuelling e s−1 induces an early transition to ELMy H-mode at ∼7.3 s, while the ion temperature at the top of the density pedestal
remains
1.3 keV. In the optimised 2.3 MA pulse #97781, this initial gas puff is delayed by ∼1 s, thereby delaying the density rise and the onset of the ELMs.
During the main heating phase in pulse #96501, divertor heat loads were mitigated using 4 Hz sweeping of the outer strike point radius, which was located close to the divertor cryopump. As part of the optimisation of pulse #97781, the sweeping frequency was increased to 20 Hz, with the strike point slightly further from the cryopump. This may have affected the ELM behaviour because the ELMs appear to be preferentially triggered during a specific phase of the sweep cycle and the outer strike-point location can affect the pumping behaviour.
A striking consequence of this delayed gas fuelling is to increase both and the central
particularly early in the pulse, just after the low-gas phase, when
keV. The concomitant increase in
with
is partly a result of a degree 'stiffness' of the core heat transport, i.e. that the ion heat diffusivity χi
increases above the threshold temperature gradient
required to destabilise ion-scale turbulence [30].
In the non-optimised pulse #96501, the radiated power quickly increases to ∼10 MW by 7.9 s, representing a fraction
of the heating power. Thereafter,
remains quite constant until 9.5 s when there is a temporary drop in the neutral beam injection (NBI) power, after which it increases to almost 50%. The significance of this observation is discussed later in section 3.4.
In contrast, in pulse #97781 with the optimised gas-fuelling, the onset of the ELMs is delayed by ∼0.7 s. During the initial ELM-free H-mode phase when is high,
is reduced by half, with
. After the onset of the ELMs,
gradually increases, rising to the same level as in pulse #96501 by 9.5 s and then decreasing slightly after the W impurities accumulate in the plasma core.
As a consequence of the higher core ion temperature , which primarily increases the thermal fusion rate coefficient
, the D–D neutron rate
is more than twice as high in the optimised pulse than the non-optimised pulse, the fraction of thermal neutrons increasing from
to
of the total rate.
2.2. Total radiation distribution
Distributions of the total radiated emissivity at 11 s determined from tomographic inversions [31] of bolometric measurements are shown in figure 2 for the same two hybrid pulses. The radiation from the confined plasma is distinguished by two features: in a central region near the magnetic axis and another located around the outer plasma mid-plane in the outer third of the plasma radius, which we refer to here as the 'mantle' region
7
. Note that the radiation from the mantle is much weaker in the optimised than the non-optimised pulse, while the emissivity from the core region is similar.
Figure 2. Comparison of the total radiated emissivity distributions from tomographic inversions of bolometric measurements for the two hybrid pulses #96501 (left) and #97781 (right) at 11 s, where outline of the first wall is shown in yellow and the separatrix contour in red.
Download figure:
Standard image High-resolution imageThere is also pronounced emission from the high-field-side (HFS) divertor region, reminiscent of the HFS high-density (HFSHD) region observed in several devices operated at high radiation fractions [32], which may play an important role in the ELM induced transport of impurities across the separatrix.
Analysis of such radiation distributions in high-power baseline pulses in JET-ILW [33] has shown that the radiated power is dominated by W, with these impurities typically emitting 80% of the total radiated power. The outwardly asymmetric poloidal emissivity distribution results from centrifugal forces on the heavy W ions due to the strong toroidal rotation driven by the tangentially oriented NBI heating [34]. The conclusion that W dominates the total radiation is supported by an integrated analysis of
, soft x-ray and total radiation measurements [35].
3. Cross-pedestal W fluxes
In this section, the main results of the experimental analysis and interpretive modelling are presented.
In section 3.1, the method of analysis used to determine the cross-pedestal W fluences (time integrated fluxes) from fast bolometric total radiation measurements, both during and between ELMs, is explained and then the results of this analysis are summarised in section 3.2. The W accumulation behaviour later in the steady, ELMy H-mode phase of these pulses is then discussed in section 3.3.
The 'ELM-flushing' data quantifies the W fluences entering/leaving the confined plasma across the pedestal region, in a manner which is independent of extensive modelling and shows that the 'normal' situation of W impurities entering the plasma between ELMs and being flushed out again by the ELMs is reversed in the later phase of the optimised pulse.
In order to better understand these observations, the collisional, NC convective fluxes of the W impurities across the pedestal and mantle regions are then discussed section 3.4, firstly introducing the necessary theoretical expressions in section 3.4.1 and then evaluating simplified proxies for the magnitude and strength of the NC convection in section 3.4.2. These are based on averaged values of the parameter (to which the NC convection velocity is proportional when the impurities are in the collisional PS regime).
Because of an uncertainty in the reliability of the charge-exchange recombination spectroscopic (CXRS) measurements across the pedestal gradient region, which are made on impurities rather than the main
ions, the proxy for the NC convection
and also the related parameter ηi
(which, in the PS regime, has to exceed ∼2 for an outward NC impurity flux, i.e. for
) are also calculated under the limiting assumption that
across the pedestal region as the parameters
and
.
The behaviour of these proxies for the NC convection, both averaged over the pedestal gradient region and also its maximum value across the mantle region
shows: (a) in both pulses the NC impurity convection is always outward across the mantle region, i.e. there is temperature gradient screening (b) using the measured Ti
across the pedestal gradient region
, consistent with strong inward convection. However, under the assumption that
across this region, this parameter reverses sign, i.e.
, consistent with impurity screening across the pedestal.
Evidence for hysteresis of the NC impurity screening, which appears as a consequence of a temporary reduction of NBI heating power in the non-optimised pulse, is presented in section 3.4.3, which shows that once the hot pedestal conditions are lost, impurities enter the plasma causing an irreversible change, from which the pulse does not recover.
The means to easily calculate this proxy for the NC convection across the pedestal and the mantle regions as a function of time from the Thompson scattering (TS) and CXRS profile measurements allows the inter-ELM dynamics of the NC screening to be resolved, as presented in section 3.4.4.
Calculations of the NC W transport across the mantle region performed using NEO are presented section 3.5, which highlight the importance of enhancement of the magnitude of W screening across the mantle region by the stronger toroidal rotation at the pedestal top in the optimised pulse.
The kinetic profiles from the TS and CXRS measurements, which are used as input to these calculations, as well as those of the derived Mach number and collisionality, are presented in section 3.5.1, which also discusses in more detail the possible reasons why the CXRS measurements of Ti
and
may be unreliable in the pedestal gradient region.
The resulting transport coefficients of the W impurities from NEO calculations, which are performed both with and without toroidal rotation, are then presented in section 3.5.2, showing that both the NC diffusion and convection are strongly enhanced across the mantle region by the toroidal rotation, particularly in the optimise pulse.
As an experimental confirmation of these calculations, the resulting total emissivity profiles of the W impurities, which dominate the total radiated power in high-power JET-ILW pulses, are calculated as described in section 3.5.3 and compared with bolometric measurements in section 3.5.4.
Calculations using NEO of the NC W transport for a case where the input profiles are forced to give across the pedestal gradient region have not been performed because of the lack of direct measurements to support this hypothesis. Instead, the fact that the simplified proxy
is consistent with temperature gradient screening across the pedestal gradient region is considered as far as any proof of this conjecture can be taken here.
3.1. ELM W 'flushing' analysis
The fact that the total emissivity is dominated by W in such pulses can be used to measure changes in the relative W content
of the confined plasma due to ELMs and inter-ELM cross-pedestal particle transport, as described in [12]. This method [36] also relies on the fact that the cooling factor of W is relatively constant at
W m3 over temperature range prevailing over the mantle region, i.e.
keV [37].
This, and the fact that the radiated power is proportional to ne
, i.e. , where
is the W density, can be availed of to determine relative changes in the W content using the relation
, where the 'flushing' signal
, where
is the total radiation from the mantle region and
the line-averaged density measured along an interferometer chord through the mantle region [12]. Examples of the flushing signal
and the derived, average W concentration over the mantle region
, which is typically ∼2–3 × 10−4, are shown figure 3(a).
Figure 3. Evolution of hybrid-scenario pulses #96501 (left) and #97781 (right) showing: (a) the flushing signal (blue) and the mean W concentration over the 'mantle' region
(red); (b) relative changes in the W content of the confined plasma
due to ELMs (
) and during the inter-ELM periods (
) and the net change over the ELM cycle (
); (c) the net rate of change of the W content
(black) and that due to ELMs (red), the inter-ELM transport (blue); (d) the FSA W density
as a function of normalized radius
; (e) the parameter
calculated using the measured Ti
profile averaged over the pedestal region
(
) and its maximum value over the mantle region
(
) (for comparison
, calculated assuming
is also shown (
)); and (f) the toroidal rotation at the density pedestal top
(
) and the pedestal temperatures
(
) and
(
).
Download figure:
Standard image High-resolution image3.2. ELM and inter-ELM W fluences
Relative changes in the flushing signal over the intra-ELM and inter-ELM periods, which are
(1 ms) and
(10–100 ms) in duration respectively, can be used to quantify relative changes in the W content
, due to the W fluences (i.e. the time-integrated fluxes) caused by the ELMs and transport across the pedestal between the ELMs respectively. Examples of such
data are shown in figure 3(b), which also shows the net change over each ELM cycle. This data can also be used to determine rates of change of the W content
due to these processes, which are shown in figure 3(c) to be
(1–3 s−1).
Average values of these relative changes in W content in the mantle region are stated in table 1 for the two pulses over time periods both during the initial phase of 7.5–9.0 s and later during the steady ELMy phase 9.5–12.0 s, after some impurities have migrated to the core. The data plotted in figure 3(b) from the post-accumulation phase is also plotted vs the relative ELM density losses in figures 4 and 5.
Figure 4. The relative W content flushed by the ELMs (a) and the relative inter-ELM influx
(b) vs the relative ELM density losses
for pulse #96501 during the post-accumulation phase over the time period 9.5–11.5 s, where the histograms show the amplitude distribution on both axes, with average and standard deviations indicated by the error bars.
Download figure:
Standard image High-resolution imageFigure 5. As figure 4 for pulse #97781.
Download figure:
Standard image High-resolution imageTable 1. Relative changes W content per ELM () and inter-ELM period (
) for the two hybrid pulses shown in figure 3 averaged over the stated time periods.
Pulse |
![]() |
![]() | ||
---|---|---|---|---|
# | (%) | (%) | ||
![]() | 7.5–9.5 s | 9.5–11.5 s | 7.5–9.5 s | 9.5–11.5 s |
96501 |
![]() |
![]() |
![]() |
![]() |
97781 |
![]() |
![]() |
![]() |
![]() |
It can be seen from figure 3(b) that in the non-optimised pulse #96501, the ELMs typically flush out ∼7% of the W from the mantle region, while a similar fraction then 'leaks' back into the confined plasma between the ELMs due to cross-pedestal transport. This is the typical situation when inward NC convection peaks the W impurity density in the pedestal region [22]. The net changes per-ELM cycle are consequently much smaller, which is consistent with the relatively steady W concentration and radiated power.
In contrast, in the optimised pulse #97781 with the higher , the typical magnitude of the changes in
due both to the ELMs and inter-ELM transport are much smaller (
1%). However, on-average the situation is reversed, i.e. the inter-ELM transport expels W from the confined plasma, while the ELMs allow some W back into the plasma from the SOL.
This change in behaviour to a regime where the W impurity content is controlled by ELM flushing to a regime with peripheral impurity screening is illustrated clearly in figure 6. These observations are consistent with a hollow W impurity density in the pedestal region [22], due to the presence of NC temperature gradient screening across the pedestal.
Figure 6. A comparison of the relative changes in the W content of the plasma due to ELM flushing vs the change due to the inter-ELM influx
between the non-optimised pulse #96501 and the optimised pulse #97781 for the time period 9.5–11.5 s, showing the change from ELM flushing to peripheral screening behaviour.
Download figure:
Standard image High-resolution image3.3. W accumulation behaviour
The density profile of the heavy impurities nZ
is largely governed by the ratio of collisional, NC convection and diffusion [38]. If the impurities are in the collisional, Pfirsh–Schlüter (PS) regime, the stationary impurity density profile is related to that of the bulk ions ni
according to
, i.e. a peaked density profile causes impurities to accumulate in the plasma core, while a peaked temperature opposes this, 'screening' out the impurities from the core.
For the W impurities, which typically have a mean charge in the core plasma of , if these accumulate, the impurity density peaking is extreme. The resulting radiation reduces the central electron temperature
, thereby modifying the current profile, quickly resulting in MHD instabilities and often plasma disruptions. For this reason, minority ion-cyclotron-resonance heating is used to inhibit full accumulation of the heavy impurities by modifying both the background plasma profiles and transport in the core [39, 40].
Because W dominates the total emissivity, its approximate flux-surface-averaged (FSA) density can be calculated from , as shown for the two hybrid pulses in figure 3(d). During these pulses, there a gradual peaking of the ion density profile
with respect to the Ti
profile, which, combined with profile changes caused by MHD islands [41], triggers reversal of the NC convection, causing the W to accumulate in the plasma core after ∼9.3 s. However, the central W concentration remains low enough not to cause intolerable Te
profile hollowing. Note that in the optimised pulse #97781, the W content is about half that in the non-optimised pulse #96501, with about a factor
less in the outer mantle region of the plasma.
3.4. Collisional, NC impurity flux
3.4.1. Theoretical expressions.
The flux of impurity ions denoted with the subscript I of charge state Z averaged over a flux surface is given by , where nI
is its density and VI
is the radial convective velocity. When the main ions are in the collisionless, 'banana' regime and the impurity ions are in the collisional, PS regime and uniformly distributed over a flux surface, i.e. centrifugal effects due to toroidal rotation are negligible (
), the impurity convection velocity
is given by equation 12 of [42]:

where the classical, collisional diffusion coefficient and τii
is the self collision time of the bulk ions
8
, the 'temperature screening' coefficient
[8] and the scale lengths are defined as, e.g.
, i.e. positive for a peaked profile. Hence, the density gradient drives the impurities inwards causing them to accumulate in the plasma core, while the Ti
gradient acts to inhibit this accumulation.
Note that a corollary of equation (1) is that the condition for temperature screening of the impurities () in the PS regime is for the parameter
to exceed the critical value
.
For convenience, we define the bracketed term as a proxy for the NC convection as , which is simple to calculate to give an approximate estimate of the strength of the impurity convection, such that
. Typically, the W ions are in charge states
in the mantle region [33], so this convection is very strong for the W ions.
In the case of strong toroidal rotation ((1)), e.g. as driven by tangential NBI heating, centrifugal effects localise the heavy impurities such as W (
) to the outboard low-field side (LFS) mid-plane of the plasma. These effects are treated for the case of the heavy impurities in the PS regime in [42], in which it is shown that with strong localisation, the impurity fluxes are enhanced by the factor
, where the inverse aspect ratio
, i.e. by a factor ∼5 for the peripheral region of JET-ILW.
For the core plasma parameters achieved in these high-power hybrid-scenario pulses in JET-ILW, i.e. relatively low density and high temperature, the impurity ions are also in the banana regime. Under these conditions, a more complete treatment of NC theory for arbitrary collisionality regimes is required.
A convenient analytic form for the NC transport coefficients is derived in [15], which provides an approximation to results of the drift-kinetic code NEO [18], encompassing scans over the relevant parameter space. A general form for the impurity flux components is given by equation 2 of [15]:

where the superscript c denotes the component due to classical (CL), PS and banana-plateau (BP) transport processes and is the convection velocity. Both diffusion
and convection
coefficients are positive, hence, for normal, peaked profiles (L > 1), the diffusive flux is outward and the convective flux inward. However, because
, the dominant, friction driven convection is inward [15].
The coefficients ,
,
in equation (2) are each dependent on the parameters
, where
is the collisionality parameter and
is the main ion collisionality. Note that the ions are in the banana or PS regimes when
and g > 1 (or
and
) respectively—at the periphery of JET-ILW plasmas,
.
Note that the convection coefficients HI
and KI
are both linearly proportional to the diffusion coefficient DI
in all collisionality regimes, so the total convection driven impurity flux (and hence its net radial convection velocity VI
) increases with DI
. However, the temperature screening parameter (where HI
and KI
are sums over each of the components, index c), is independent of the magnitude of DI
.
3.4.2. Estimates of strength and direction of NC convection.
To give an approximate estimate of the strength and direction of the NC impurity convection, the spatial average of the convection parameter , calculated over the pedestal region and its maximum value over the mantle region are shown in figure 3(e), i.e.
and
respectively. For calculation of
, Ti
data from only the edge charge-exchange-recombination spectrometer system [27] is used and we assume
, where the ne
profile is measured by the high-resolution Thomson scattering system [44].
To implement this analysis, profiles of the quantities ηi
and , which require combining fitted Ti
and ne
profile data, are first calculated by finding the closest CXRS data point to each of the TS pulses. Hence, the detailed behaviour of these quantities is approximate on timescales below 5 ms, i.e. half of the integration period of the CXRS measurements. Also, the values for
ms may be affected by the influence of the ELM on the CXRS data.
Because the pedestal width (2 cm) is of the order of the ion banana orbit width, i.e. the gyro-radius of the ions
in the poloidal magnetic field Bp
, it is appropriate to consider spatially averaged values of
(or ηi
) in the pedestal region. However, as the extent of the mantle region is
(10) larger, it is more appropriate to consider the maximum value of the screening parameters in this region. The corresponding values of
and
for the pedestal and mantle regions, averaged over time ranges before and after the W impurities accumulate in the plasma core at ∼9.5 s are stated in table 2.
Table 2. Time-averaged values over the specified time ranges of the spatially averaged values of
and
for the pedestal region and
values of
and ηi
over the mantle region, where the two values for the pedestal region are calculated using the measured Ti
and assuming
.
Pulse |
![]() |
![]() |
![]() | |||
---|---|---|---|---|---|---|
# |
![]() | 9.5–11.5 s | 7.5–9.5 s | 9.5–11.5 s | 7.5–9.5 s | 9.5–11.5 s |
96501 |
![]() |
![]() |
![]() |
![]() |
![]() |
![]() |
97781 |
![]() |
![]() |
![]() |
![]() |
![]() |
![]() |
— |
![]() |
![]() |
![]() | |||
# | 7.5–9.5 s | 9.5–11.5 s | 7.5–9.5 s | 9.5–11.5 s | 7.5–9.5 s | 9.5–11.5 s |
96501 |
![]() |
![]() |
![]() |
![]() |
![]() |
![]() |
97781 |
![]() |
![]() |
![]() |
![]() |
![]() |
![]() |
3.4.2.1. Mantle region.
Throughout both of the pulses, the maximum value of across the mantle region is maintained at
, i.e. the NC impurity convection is directed outwards, localising the W impurities to this region, where they can be efficiently flushed from the confined plasma by the ELMs.
Results of more accurate calculations of the NC convection in these pulses, performed numerically using the NEO code [18] presented in section 3.5, show that the strong enhancement due to the toroidal rotation in these pulses reveals marked differences in the NC convection between the two pulses, particularly in the mantle region, just inside the pedestal top.
3.4.2.2. Pedestal region.
Two sets of data for averaged over the pedestal region are shown in figure 3(e) to give an indication of the possible range of values:
calculated assuming
and
calculated using the Ti
profile from CXRS.
For the non-optimised pulse #96501, both estimates of are consistent with strong inward NC impurity convection across the pedestal between ELMs, i.e.
, albeit reduced in magnitude assuming
. For the optimised pulse #97781, the average value of
(–100), is also consistent with strong inward W convection across the pedestal. However, the estimate made assuming
of
in the initial phase and +50 after 9.5 s, implies screening of the W impurities by the pedestal temperature gradient. This arises due to a change, from the non-optimised to the optimised pulse, in ηi
(assuming here
) across the pedestal to a value exceeding the critical value
required for outward NC convection.
On devices with a main ion () CXRS system, e.g. DIII-D, it is often found that over the pedestal region the ion-temperature gradient
is closer to
than that measured on the impurity ions (i.e.
on DIII-D or
on JET-ILW) [45]. As there are no measurements of the main
ion temperature on JET-ILW, these estimates of
made assuming
are purely conjectural and offer an alternative explanation of the observations of (a): controlled W impurity radiation, particularly from the mantle region in the optimised pulse #97781; and (b) the apparent reversal of the flushing behaviour of the ELMs in this pulse after 9.5 s, when magnitude of
is larger; to that offered by the more comprehensive calculations of the W convection presented in section 3.5 below.
The maximum sustainable difference between temperatures of impurity ions and the main ion species due to collisional equipartition has been investigated in [46]. This steady-state analysis is appropriate for the case when the collisional equipartition time τZi
between the impurities and main ions is much shorter than the energy confinement time, i.e. , as is the case in the core plasma. However, in the pedestal region the local confinement time of the impurity ions is much shorter and may be comparable or shorter than τZi
, allowing larger temperature differences to be sustained at the plasma periphery than in the core.
As mentioned in section 2.1, the reduced early gas fuelling in pulse #97781 more than doubles the initial pedestal ion temperature at the pedestal top compared to that in pulse #96501, which remains higher throughout the pulse. As also shown in figure 3(f), the reduced early gas fuelling results in an even greater increase in the toroidal rotation rate at the pedestal top
early on in the pulse, initially by a factor ∼3, although this enhancement decreases later on when the fuelling rates are the same. Note that the pedestal top density
m−3 remains quite constant throughout the sustained phase of both pulses.
3.4.3. Hysteresis of impurity screening.
The evolution of the ELM frequency , the normalised density and temperature gradients and the parameters
across the pedestal in the non-optimised pulse #96501 and optimised pulse #97781 are shown in figure 7. After ∼9.5 s when the W accumulates in the plasma core, there is a difference in the behaviour of
between the two pulses, i.e. in pulse #96501
decreases, while in #97781
increases compared to that in the earlier phase, when it is similar in both pulses. The cause of these changes in
can be explained by the behaviour of the radiated power fraction
(see figure 1(e) and related discussion in section 2.1) in terms of the resulting changes in the loss power across the separatrix
.
Figure 7. Evolution during the two hybrid pulses #96501 (black) and #97781 (red) of: the ELM frequency (a), the normalised gradients
(b),
(c) and
(d) and the parameters
(e) and
(f) averaged over the pedestal region for the time period 7.5–12.5s. The threshold
is indicated by the dashed line.
Download figure:
Standard image High-resolution imageIn pulse #96501, just prior to the increase in at 9.5 s, there is a temporary drop in the NBI power by ∼5 MW for ∼0.3 s, after which there is a decrease in
and reduction in
, which can be explained by a reduction in
due to the increased radiated power and the power dependence of
for type-I ELMs. However, the behaviour of the optimised pulse #97781, in which the high-power heating is maintained, is quite different. Following the impurity accumulation at 9.5 s, the radiation fraction
decreases (due to the W impurities having a lower emissivity at the higher Te
prevalent in the core) and the consequent increase in
causes an increase in
.
The different ELM behaviour between the two cases is accompanied by changes to the normalised gradients across the pedestal, with slightly lower and both
and
slightly higher on average over this later phase. These changes combine to significantly increase the values of
and
, in the case of the latter causing the critical value
for outward NC convection to be exceeded in the optimised pulse. Note that the maximum values of both ηi
and ηe
across the pedestal are a factor
higher than the spatially averaged values.
If the temperature of the main ions across the pedestal were closer to Te
than to that of the Ne10+ impurity ions used for the CXRS measurements, then because
, it is possible that NC impurity screening across the pedestal gradient region is maintained throughout the later phase of the optimised pulse #97781 but is lost at the time of the NBI power drop in pulse #96501 due to a reduction of the pedestal Ti
gradient. This highlights the necessity of sustained, high-power heating to maintain W impurity temperature gradient screening at the plasma periphery that is initially set up by optimising the gas fuelling.
3.4.4. Inter-ELM dynamics of NC convection.
The density losses caused by the ELMs change the gradients and hence the profiles of the derived parameters and
in the pedestal and mantle regions, which then evolve during the subsequent inter-ELM period. The HRTS measurements of the Te
and ne
profiles are essentially instantaneous at the laser pulse times, which are separated by 50 ms and occur at random times relative to the ELMs,
. Similarly, the CXRS measurements of the Ti
profiles are available at an integration time of 10 ms at 100 Hz sample rate.
Hence, the kinetic profile data can be used to determine the inter-ELM evolution of the NC impurity screening across the pedestal and mantle regions. The inter-ELM evolution of the parameters and both
and
relative to the time of the ELM
are compared in figure 8 between the two hybrid pulses #96501 and #97781.
Figure 8. Inter-ELM evolution of the parameters (a), (b) and
(blue) and
(red) (c), (d) averaged over the mantle and pedestal regions for the two hybrid pulses #96501 and #97781 for the time period 9.5–11.5 s. Data are at the times of the TS laser pulses plotted relative to the time of the previous ELM
. The threshold
is indicated by the dashed line.
Download figure:
Standard image High-resolution image3.4.4.1. Mantle region.
The ELM density losses reduce the pedestal density and hence increases the both the absolute and normalised density gradients
and
across the mantle region just inside the pedestal top, resulting in a decrease of the parameter
to
. As
increases during the inter-ELM period,
decreases across the mantle and consequently
increases to
by the end of the ELM cycle. Hence, the NC impurity screening weakens after each ELM due to the stronger density gradient across the mantle and then strengthens again during the ELM cycle. Consequently, a higher ELM frequency might be expected on average to reduce the impurity screening across the mantle region.
3.4.4.2. Pedestal region.
The inter-ELM dynamics of for the pedestal region are the inverse of that for the mantle region, i.e. the decrease in
due to the ELMs decreases
across the pedestal and hence increases
just after the ELMs. The subsequent increase of
and hence decrease of
across the pedestal then causes
to decrease during the ELM cycle. As the values of
, the NC impurity convection across the pedestal is always expected to be strongly inward (
) in both pulses, consistent with the data in table 2, at least if it is the case that the edge CXRS measurements give the correct
ion temperature in the pedestal region.
3.5. Numerical calculations of the W transport
Toroidal rotation affects the transport coefficients defined in equation (2) by modifying the poloidal distribution of the particle densities, particularly of the heavy impurities such as W. This introduces a further dependence on the toroidal Mach number Mφ
as well as the parameters . Note that the analytic model of Fajardo et al [15] has recently been extended to incorporate these dependencies. This will be the subject of a forthcoming publication [16], which will be of use for future interpretive analysis and predictive modelling.
Results from calculations performed using the drift-kinetic solver NEO for ions on a flux surface just inside the pedestal top (
, where
) of a JET-ILW equilibrium are presented in figure 9, which shows the dependencies of the enhancement of the impurity diffusion coefficient due to toroidal rotation
and of the temperature screening parameter
on the collisionality parameter
for various values of the generalised impurity Mach number
.
9
Figure 9. The dependence on the collisionality parameter of: (a) the ratio of the W diffusion coefficient calculated with rotation to that without
and (b) the temperature screening parameter
for a range of values of the generalised impurity Mach number
calculated using NEO for
at the
flux surface of a JET-ILW equilibrium.
Download figure:
Standard image High-resolution imageThe primary effect of increased rotation is to increase all three transport coefficients () over their values without rotation, increasingly so over the collisionality range
. However, the effect of rotation on the temperature screening parameter
is more complex, depending on whether the impurities are in the PS or BP regime. The magnitude of
increases, both at low collisionality (
), where
and
screens the impurities, and at high collisionality (
, not shown in figure 9), where
and
enhances the impurity pinch. In the intermediate collisionality regime, the value of g where
changes sign increases with
and is also dependent on the other parameters
and hence on the ion species and radial location within the plasma.
Hence, at low collisionality and high Mach number an operational window opens where the temperature screening of impurities is enhanced by increasing rotation, opposite to the impact of rotation at high collisionality [9]. Note that over the mantle and pedestal regions the screening parameter , except close to the separatrix where it decreases significantly. Hence, it is a valid assumption to assume this value of
when calculating the screening parameter
discussed in section 3.4 above.
3.5.1. Kinetic profile data.
An example of the raw and fitted kinetic profile data used for calculations of the NC W transport is shown in figure 10 for pulse #97781, averaged over a 1 s period of the post-accumulation phase from 10.5–11.5 s, where both the raw and fitted data are averaged separately, after performing separate fits to measurements at 50 ms intervals
10
. Both the raw ne
and Te
data from HRTS is measured at the laser repetition period of 50 ms and the CXRS Ti
and data is measured with an integration period of 10 ms. Profiles measured during or immediately following ELMs are not included in the set of averaged profiles.
Figure 10. Profiles of ne
(a); Te
and Ti
(b); (c) for the pulse #97781, where the fits (lines) and raw measurement data (points) from HRTS and the core and edge CXRS systems are both separately averaged over the 1 s time period 10.5–11.5 s.
Download figure:
Standard image High-resolution imageThe profiles are fitted using a 3rd-order polynomial over the core and an function [47] for the pedestal region, which is constrained to give zero value in the SOL. The requirement to fit the whole core profile with a rather stiff function is the reason why the fitted profiles depart from the measurements in some places. However, this does provide a reasonable interpolation between the measurements from the core and edge CXRS systems, the former giving higher values of Ti
and
than the edge system over the region where the measurements overlap. Note that only the edge CXRS data is used in the calculations of the NC convection parameter
presented earlier.
It is striking that, over the steep-density gradient region of the pedestal, the measured Ti
exceeds Te
, with a much weaker gradient and relatively high value ∼1 keV at the separatrix, where eV. Also, the
data is particularly not well represented by the
function in this region and the measured points are also non-monotonic.
There are several reasons why the CXRS measurements of Ti
and over the pedestal region are challenging and it is particularly difficult to determine their gradients accurately, this affecting the derived values of ηi
across this region:
- The rapidly decreasing
density outwards across the pedestal results in lower CX line intensities for the Ti and
measurements, making these less reliable and prone to contamination by small impurity lines towards and outside the separatrix.
- The off-mid-plane CXRS measurements (and to a lesser degree the HRTS measurements) require mapping to the magnetic axis by means of the magnetic equilibrium to be able to align them to calculate the profile of ηi
across the pedestal. This requires using a pressure-constrained EFIT equilibrium (EFTP) with well-defined separatrix location, which uses the separatrix temperature
eV as an additional constraint in the reconstruction.
- Fast EFIT equilibrium reconstructions or flux measurements show that there are small movements of the separatrix location by 1–2 cm during the ELM cycle due to changes in
during the ELM cycle, which imposes an intrinsic limitation on the accuracy of the mapping, which uses the EFTP equilibrium available with lower time resolution.
- As discussed earlier, on devices with a main ion (
) CXRS system, e.g. DIII-D, it is often found that over the pedestal region the ion-temperature Ti is closer to Te than that measured on the impurity ions (i.e.
on DIII-D or
on JET-ILW) [45]. Without main-ion CXRS measurements in the pedestal region, there is no way to determine whether this is also the case under these conditions on JET-ILW.
3.5.2. Calculation of the W transport coefficients.
Profiles of the parameters used to perform the NEO calculations are shown in figure 11 for the two pulses #96501 and #97781. As the temperature screening parameter across the full profile, in the case of the normalised temperature gradient
is shown for comparison with
because where the
impurity screening is expected.
Figure 11. Profiles of parameters input to NEO vs' normalised radius for the two pulses #96501 and #97781: (a), (b) the normalised gradients
(green) and
(black); (c), (d) ion collisionality
(blue) and Mach number of the
ions
(magenta), averaged over the time periods 7.5–8.5 s (solid) and 10.5–11.5 s (dashed).
Download figure:
Standard image High-resolution imageJust inside the top of the pedestal
11
, at the toroidal Mach number of the
ions
in pulse #96501, which corresponds to a Mach number
for the highly charged
ions, while in the optimised pulse #97781 these values are a factor
higher. The collisionality of the
ions is in the range
, i.e. in the collisionless banana regime.
Profiles of ηi
and values of the inverse screening parameter are shown in figure 12, so that regions where impurity screening is expected (where
) can be discerned, i.e. in particular across the mantle region (
) inside the pedestal top, whereas in the pedestal region strong, inward impurity convection is expected.
Figure 12. Profiles of the parameter ηi
(black) and the inverse of the impurity screening parameter vs' normalised radius
calculated using NEO for the input profiles shown in figure 11 for the two pulses #96501 (a) and #97781 (b), averaged over the time periods 7.5–8.5 s (solid) and 10.5–11.5 s (dashed).
Download figure:
Standard image High-resolution imageProfiles of the calculated W diffusion coefficient and of the NC convection velocity
for the W ions are shown in figure 13 for the pulses #96501 and #97781. Profiles calculated both with and without rotation show that the NBI-driven toroidal rotation enhances the impurity transport by
(10) above the level for a non-rotating plasma. Furthermore, the higher Mach number
early on in the optimised pulse #97781 enhances W transport coefficients, increasing
in the mantle region, by a factor
at
compared to that in pulse #96501. This enhancement also increases the outward W convection velocity
by a similar factor.
Figure 13. Profiles of the NC W convection velocity vs' normalised radius
calculated using NEO for the input profiles shown in figure 11 for the two pulses #96501 (a) and #97781 (b), averaged over the time periods 7.5–8.5 s (solid) and 10.5–11.5 s (dashed).
Download figure:
Standard image High-resolution imageThe resulting values of and
can be used to calculate the W density profile, both as a FSA quantity
and across the LFS major radius through the magnetic axis
, as described below. These are used to calculate the total corresponding emissivity profile of the W impurities
, which are then compared with the total emissivity
derived from bolometric measurements.
3.5.3. Calculation of the W density and emissivity profiles.
The density profile of the W impurities is calculated following the approach described in [48]. In steady-state conditions, in the core plasma there is an absence of impurity sources and sinks. Hence, simplifying the notation for the FSA, the continuity equation for the impurities implies that everywhere the W flux
.
12
Neglecting the contributions to the W transport from NC diffusion and the anomalous turbulent pinch, i.e. assuming and
(where
and
are the turbulent impurity diffusivity and convective pinch velocity), the W flux is given by
. Hence, the logarithmic W density gradient can be expressed as
, where the
. It is then straightforward to integrate this expression radially, taking the boundary condition as the value of
either at the magnetic axis or separatrix, to yield the W density profile across the LFS mid-plane, where the poloidal angle θ = 0:

For calculation of the FSA W density, it is necessary to know its dependence on the poloidal location around the flux surface, i.e. , where r is a suitable flux-surface label, e.g.
. The centrifugal force acting on the heavy impurities due to toroidal rotation concentrates their densities at the LFS of the plasma [34], with their density distribution given by equation 6 of [48]:
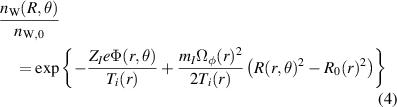
where is the calculated electrostatic potential and
. The FSA density
can then be calculated in the usual way
13
.
For calculation of the anomalous turbulent impurity diffusivity , this is assumed to be equal to that of the background
ions
,
14
where an expression for mixed Bohm/gyro-Bohm transport is used [49], as described in [50].
For comparison with data from tomographic reconstructions, e.g. as shown in figure 2, total emissivity coefficients for W impurities in coronal equilibrium from the ADAS atomic database [37] are used to calculate the total emissivity of the W ions,
. Note that because the electron temperature is a flux function
, either the FSA profile or LFS profile of
can be calculated using the appropriate density profile,
or
.
3.5.4. Comparison of total emissivity profiles.
Profiles of the measured total emissivity across the LFS mid-plane, through the magnetic axis () determined from tomographic reconstructions of the bolometer data are compared to the calculated emissivity profiles in figure 14 for the two pulses #96501 and #97781. The profiles are averaged over two 1 s time ranges during the early phase (7.5–8.5 s) and later (10.5–11.5 s), after the W has accumulated in the plasma core. Because only the relative emissivity profiles can be calculated without knowledge of the W influx, the calculated profiles are normalised to the measured values at R = 3.5 m.
Figure 14. Total emissivity profiles across the LFS mid-plane (@ ) vs major radius R determined from tomographic reconstructions of the bolometric data (blue) and calculated using the NC transport coefficients from NEO (red) for the two pulses #96501 (a) and #97781 (b) for the time periods 7.5–8.5 s (solid) and 10.5–11.5 s (dashed).
Download figure:
Standard image High-resolution imageFor pulse #96501, the shape of the profiles are reasonably well reproduced using the transport coefficients from NEO
15
. Outside the separatrix (
m),
is not calculated, while the measured profile may be broadened somewhat into the SOL due to the finite spatial resolution (
∼ 0.05–0.1 m) of the measurement and reconstruction.
In the case of pulse #97781, over the mantle region, the calculated profiles are hollow, consistent with the outward NC convection. The increasing gradient towards the periphery can be ascribed to a local overestimation of the NC convection
or more likely an underestimate of the anomalous diffusivity
. The accumulation of the W at the later time period is well reproduced by the calculation in this pulse. Note that, at the later time, whereas the emission from the core plasma is similar between the two pulses, the emissivity from the mantle region is almost a factor
higher in the non-optimised pulse #96501.
The profiles in figure 15 show that the radiation from the mantle region is far less significant in a FSA sense compared to that from the LFS mid-plane where the W is localised by the toroidal rotation. However, the larger volume of this region compared to the core (
) results in
of the radiated power being emitted from impurities in the mantle, while only
is emitted from the core. Note that both the calculated and measured
profiles are consistent with significant localisation of the W impurities close to the separatrix due to the outward NC convection.
Figure 15. FSA total emissivity profiles vs normalised radius
determined from tomographic reconstructions of the bolometric data (blue) and calculated using the NC transport coefficients from NEO (red) for the two pulses #96501 (a) and #97781 (b) for the time periods 7.5–8.5 s (solid) and 10.5–11.5 s (dashed).
Download figure:
Standard image High-resolution imageAlthough FSA data is not available outside of the separatrix (), the LFS profiles in figure 14 show that there is significant emission from impurities the SOL region. The 2D distributions in figure 2 show that the radiation from the pedestal foot and SOL is dominated by emission from the region above the HFS divertor leg. Strong emission from this region is frequently observed in high-power H-mode pulses in JET-ILW and has been attributed to the poloidal localisation of impurities to a so-called HFS high-density (HFSHD) region [51]. The significance of this will be discussed in section 4 below.
4. Summary and conclusions
4.1. Evidence for high-Z impurity screening
Reduced gas fuelling during the initial H-mode phase of the optimised hybrid pulse #97781 results in both significantly higher () ion temperature
and toroidal rotation at the pedestal top
, particularly early in the pulse, although the enhancement persists later into the steady ELMy H-mode phase. The effect of the strong toroidal rotation (
(0.4)) is to localise the heavy impurities to the LFS mid-plane, thereby enhancing the level of collisional NC transport by
(10).
During the initial phase, before the W impurities accumulate in the core, analysis of the fast bolometry signals reveals that in the optimised pulse, there is a reduction in changes to the relative W content of the plasma due both the ELM flushing and inter-ELM ingress of these impurities relative to those in the non-optimised pulse. This indicates that the influx of W across the pedestal is already reduced during this early phase.
Calculations using NEO of the NC transport of the W impurities using measured ELM-averaged kinetic profiles, reveals that there is certainly screening due to outward convection () in the mantle region, just inside the pedestal top in both of the pulses compared here. This screening, which occurs when
, is enhanced by the
stronger toroidal rotation at the pedestal top in the optimised compared to the non-optimised pulse.
Note that calculations with ASTRA [52] for Q = 10 ITER plasmas [53], consistent with evaluations of turbulent transport and intrinsic torque [54], predict reduced toroidal Mach number of the D+ ions Mϕ,I at the pedestal top of ∼0.11, 16 which is considerably lower than the values of ∼0.3 − 0.4 for the hybrid-scenario plasmas discussed here. As discussed in [16], the current expectation of low rotation in ITER implies that it will likely not significantly profit from rotation-enhanced W screening across the mantle region, although W screening is expected to occur across the pedestal gradient region [55].
As evident from the FSA emissivity profiles, the presence of less W in the mantle only partly explains why the ELMs flush less W from the confined plasma in the optimised pulse—the factor reduction in
is less than the decrease in the W flushing efficiency
and also of the corresponding inter-ELM influx. Although after the initial phase (t > 8 s), the gas fuelling is the same in both pulses, differences in their behaviour persist, i.e. the higher
and
in the optimised pulse (both enhancing impurity screening) and also in the radiation and ELM behaviour.
After the W accumulates in the plasma core, in the non-optimised pulse, following a temporary drop in the NBI heating power, the radiated power fraction increases significantly. The resulting reduction in the loss power
across the pedestal causes both the ELM frequency
and also
to decrease, which consequently reduces the peripheral impurity screening. This results in a loss of performance in terms of energy confinement and neutron rate due to the higher radiated power and lower core temperature. This observation highlights the importance of sustained high-power heating in maintaining the temperature-gradient impurity screening at the plasma periphery.
The inter-ELM dynamics of the impurity screening caused by the ELM density losses exhibit opposite trends for the convection across the pedestal gradient region and the mantle. Just after the ELMs, temperature screening across the mantle is reduced by lower , while inward convection across the pedestal is reduced by a higher
, so these effects partially compensate from the point of view of the net influx.
ELM flushing results from the fast bolometry exhibit a reversal of the typical situation during the later phase of the optimised pulse, i.e. instead of the ELMs flushing W from the plasma, on average they slightly increase the W content of the plasma (by 1%), which is then expelled between the ELMs rather than entering the confined plasma. This observation, together with the fact that
remains controlled, indicates that during this later phase: (a) there is efficient impurity screening at the pedestal, resulting in a hollow impurity density profile across the pedestal; (b) this has the consequence that ELMs can transport impurities into the confined plasma rather than flush them out, as originally identified in simulations performed for ITER [7, 56].
Using the available ion temperature measurements from the edge CXRS system, it has not been possible to confirm the presence of NC temperature-gradient impurity screening across the steep-density gradient region of the pedestal by modelling of the NC transport. However, it is possible that, were the ion temperature gradient across the pedestal to be higher than that of the impurities, such screening may also be present across the steep gradient region—assuming that
across the pedestal does result in sufficiently high
for screening. Without a measurement of the
temperature, there is no way to be sure but the other results presented here are consistent with this conjecture.
The observation that ELMs can cause an ingress of impurities into the confined plasma is probably due to an interchange nature to this explosive instability [22], 17 rather than a reversal of the collisional NC transport during the ELMs. If the impurity content of the outer pedestal/SOL plasma is higher than that in the mantle region, just inside the pedestal top, the ELMs can cause a net increase in the impurity content of the confined plasma, a fact which has consequences for the operation of ITER.
During the recent JET-ILW DT campaign (DTE2) at the end of 2021, this hybrid scenario was used to run the best performing DT pulse #99950 with a record fusion energy of 45.8 MJ and power averaged over 5 s of 8.3 MW [58], albeit with slightly modified gas fuelling to account for the slower response of the tritium injection module and increased particle confinement in DT compared to D plasmas. Both this pulse and the D pulse #100822, with matched engineering parameters, exhibited similarly high edge Ti and reduced edge radiation as in the optimised D pulse #97781 discussed in detail here.
4.2. Significance for ITER operation
Since the effect of NC temperature gradient screening scales with impurity charge Z, it is stronger for W relative to turbulent impurity transport than for lower Z impurities and so easier to detect. Furthermore, this empirical confirmation of this phenomenon relies on bolometric measurements of rather than spectroscopy, which is very difficult for W. The concomitant observation that ELMs allow W back into the plasma, in optimised high-power hybrid-scenario pulses on JET-ILW is important for several reasons:
Firstly, scenarios that integrate core fusion performance, i.e. high fusion gain Q, and tolerable inter-ELM divertor power loads automatically require a high separatrix to pedestal density ratio , resulting in W pedestal screening [55]. However, with inter-ELM W screening, the requirements for ELM control and W efflux are orthogonal, which questions whether active triggering of the ELMs for their control can be solution to the ELM problem.
If W brought into the plasma by ELMs (as predicted in [22]), and only expelled between them, then increasing the ELM frequency will increase
, eventually causing an H/L transition [7]. Furthermore, a weak decrease of the ELM target heat flux is expected with increasing
. Hence, increasing
cannot give a consistent solution to the power flux and W contamination problem in the presence of W screening [56].
This conundrum does not occur in ITER-baseline scenario pulses, in which W is localised to the mantle and hence power and impurity exhaust go together [12], as was assumed when the original ELM control requirements for ITER were derived [59]. Hence, these new JET results justify that ELM suppression rather than mitigation by controlled ELM triggering is the preferred solution for ITER, since it can provide the solution to the power exhaust problem and, together with W pedestal screening, good W impurity exhaust. However, a disadvantage of this strategy for use in a future fusion reactor, is that fuelling of the confined plasma with extrinsic impurities, e.g. the noble gas Ar, as a means of mitigating the power exhaust problem would be ineffective.
Acknowledgments
This work has been carried out within the framework of the EUROfusion Consortium, funded by the European Union via the Euratom Research and Training Programme (Grant Agreement No. 101052200—EUROfusion) and from RCUK Energy Programme (Grant No. EP/W006839/1). Views and opinions expressed are however those of the author(s) only and do not necessarily reflect those of the European Union or the European Commission. Neither the European Union nor the European Commission can be held responsible for them. ITER is the Nuclear Facility INB No. 174. The views and opinions expressed herein do not necessarily reflect those of the ITER Organization. To obtain further information on the data and models underlying this paper please contact PublicationsManager@ukaea.uk.
Footnotes
- 5
The normalised pressure
, where βT is the ratio of volume-averaged thermal to magnetic pressure.
- 6
The safety factor is defined as
, where Φ and Ψ are the toroidal and poloidal magnetic fluxes within a surface enclosing the flux Ψ and q95 is the value at the 95% flux surface.
- 7
The mantle region is defined here as
, where the normalised radial coordinate
and
is the normalised toroidal flux.
- 8
The self-collision time of the hydrogenic main ions is defined as
, where mp is the proton mass and
is the Coulomb logarithm [43].
- 9
The generalised Mach number of the impurity ions is defined as
.
- 10
Note that the profiles must be plotted vs'
rather than
because the toroidal flux is not defined outside the LCFS.
- 11
The top of the density pedestal is located at
.
- 12
Note that it is not necessary to model the transport of each ionisation stage of the impurities separately because the relevant atomic rate coefficient used to calculate the total emissivity
is available for a 'bundled' ensemble of ions at the appropriate fractional abundances for the impurities in coronal equilibrium [37].
- 13
The flux-surface average of a quantity X is defined as
, where Bp is the poloidal component of the magnetic field and lp is the path length around the flux surface in a plane of constant toroidal angle.
- 14
Note that because the anomalous transport is expected to be due to electrostatic turbulence, the associated transport due to fluctuating
drifts is the same for all particle species, independent of charge and mass [38].
- 15
As mentioned previously, it is a good approximation in high-power JET-ILW pulses that radiation from W impurities dominate the total emissivity.
- 16
The most relevant rotation profiles for ITER Q = 10 plasmas shown in figure 1 of [53] are those for a case with Prandtl number Pr ≡ χϕ/χi = 0.3 and ratio of momentum to energy confinement times τϕ/τE,th = 2 with pedestal top rotation Ωϕ,ped ∼ 10 krad/s, corresponding to Mϕ,I ∼ 0.11 with Ti,ped ∼ 4.2 keV.
- 17
The interchange nature of ELMs has been demonstrated by means of fast beam-emission spectroscopy (BES) measurements of the edge density profile on MAST [57].