ABSTRACT
We present a study of 107 Cybele asteroids based on the archival database "Asteroid Catalog Using AKARI (AcuA)" taken by the Infrared Astronomical Satellite. The database provides diameters D > 10 km, geometric albedos, and taxonomic information (75%) of the Cybeles. We find taxonomic diversity (mainly C-, D-, and P-type) in the population of 78 small Cybeles with diameters 10 km <D < 80 km. Their cumulative power-law size distribution index shows a shallow value of 0.86 ± 0.03. By contrast, 29 large Cybeles with D > 80 km are mostly classified as C- or P-types (90%), with a power-law index of 2.39 ± 0.18. The total mass of Cybele asteroids is estimated to be ∼10−5 MEarth. We also discuss the origin and formation process of the Cybele asteroid family.
Export citation and abstract BibTeX RIS
1. INTRODUCTION
The Cybele asteroid family resides at the right beyond the edge of the main belt, which has a semimajor axis of 3.27 AU < a ⩽ 3.70 AU (between the 2:1 and 5:3 resonances with Jupiter), inclination i ⩽ 25°, and eccentricity e ⩽ 0.3 (Zellner et al. 1985). The asteroids in the outer main belt, including Hildas at an average semimajor axis AU and Jovian Trojans at
AU, were believed to be formed in situ from a primordial disk (Bell et al. 1989). However, the recent dynamical work (the Nice model) proposed that they originate from trans-Neptunian objects (TNOs; Levison et al. 2009). The implications are that a large number of the outer main belt objects preserve TNOs like primitive and icy natures. Actually, they are primarily classified as C-, D-, and P7-type asteroids in the Tholen taxonomy system with low geometric albedos (Bell et al. 1989; Gradie et al. 1989; Tholen 1989; Tholen & Barucci 1989).
The taxonomic fraction of the Cybeles, Hildas, and Trojans has been statistically revealed by various types of surveys8 (Gil-Hutton & Brunini 2008; Gil-Hutton & Licandro 2010; Roig et al. 2008). Note that a fraction of C-type asteroids of the Cybeles are relatively larger (28%; Gil-Hutton & Licandro 2010) than those of the Hildas (16%; Gil-Hutton & Brunini 2008) and Trojans (⩽10%; Roig et al. 2008). This indicates that objects with nearly neutral reflectance spectra are more dominant by decreasing a down to the outer edge of the main belt, suggesting that the Cybeles are located at the unique transition zone between the inner and outer solar systems. At a ∼ 3.2 AU, main belt comets (MBCs) reside as active C-type asteroids that show sublimation of water ice (Hsieh et al. 2004, 2009, 2010; Hsieh & Jewitt 2006; Jewitt 2012). Some active MBCs are possibly products of shattering collisions from the Themis and Beagle families (Nesvorný et al. 2008; Haghighipour 2009, 2010), which perhaps involve icy parent bodies in the outer main belt (Jewitt et al. 2009). Those precursor objects are expected to possess ice-rich interiors (Trojans: Jones et al. 1990; Luu et al. 1994; Dumas et al. 1998; Emery & Brown 2003; Yang & Jewitt 2007; Fornasier et al. 2007). A broad absorption band centered at 3.1 μm has been detected on the 100 km scale asteroids (24) Themis and (65) Cybele, but whether or not this originates from surface water ice is disputable (Campins et al. 2010; Rivkin & Emery 2010; Licandro et al. 2011; Beck et al. 2011). If real, they could be intrinsically buried ice excavated by recent impacts with small, 10 m scale projectiles (Jewitt & Guilbert-Lepoutre 2012). On the other hand, massive collisional activities between 10 and 100 km scale objects are responsible for shaping the so-called families (Davis et al. 2002, and references therein). The Cybele family is also presumed to have experienced shattered events in the past only because numerous small D-type asteroids are found in common with the potential collisional families: the Hildas and Trojans (Lagerkvist et al. 2005). The latter two have been distinctively observed (spectroscopy, optical, and infrared imaging), and their physical parameters of sizes, geometric albedos, and taxonomy have been statistically measured (Jewitt & Luu 1990; Fitzsimmons et al. 1994; Dahlgren & Lagerkvist 1995; Dahlgren et al. 1997; Dahlgren 1998; Jewitt et al. 2000; Yoshida & Nakamura 2005; Szabó et al. 2007; Fernández et al. 2009; Ryan & Woodward 2011; Grav et al. 2011, 2012), as well as dynamical models (de Elía & Brunini 2007; Brož & Vokrouhlický 2008; Levison et al. 2009; Brož et al. 2011). As for the Cybele family, their taxonomic features have been revealed with a large number of samples (Lagerkvist et al. 2005; Gil-Hutton & Licandro 2010), whereas there are only a few thermal infrared studies on the representative object (65) Cybele (Müller & Blommaert 2004; Licandro et al. 2011). To further discuss the collisional history, infrared information of the population is necessary.
As stated above, physical properties of size, albedo, and taxonomy offer a creative window into the asteroid family background. Despite this, those of the Cybele asteroids have been little known. In this paper, we present infrared observational results (diameters and geometric albedos) and taxonomic characters of the Cybeles extracted from the archival database "Asteroid Catalog Using AKARI (AcuA)" (Usui et al. 2011). We study their orbital properties, size distribution, and diameter/albedo–taxonomy relations. Based on these results, we discuss the origin and formation of the Cybele asteroid family.
2. ARCHIVAL DATABASE: AcuA
Midinfrared (mid-IR) imaging data studied here were obtained as part of the AKARI Infrared Astronomical Satellite All-Sky Survey project (Murakami et al. 2007; Ishihara et al. 2010). The asteroid database is of already published as AcuA (Usui et al. 2011).
Asteroid data were taken by the Infrared Camera (IRC) in its survey mode (Onaka et al. 2007). Detectors of the IRC mid-IR two channels consist of infrared sensor arrays of 256 × 256 pixels (Si:As/CRC-744 Raytheon product) with an effective pixel scale of 94–10
4 pixel−1, giving a field of view of approximately 10' × 10' (Ishihara et al. 2010). Identified asteroids totaled 5120 and were recorded through two filters in the mid-IR, one obtained with the S9W (9 μm band) and another with L18W (18 μm band). Radiometric diameters (D) and geometric albedos in the visible wavelength (pv) of asteroids were calculated based on the concept of the Standard Thermal Model (Lebofsky et al. 1986; Lebofsky & Spencer 1989). The results are summarized in the archival database AcuA9 (Usui et al. 2011). The infrared emissivity
= 0.9 and thermal infrared phase coefficient β = 0.01 mag deg−1 were assumed (Lebofsky & Spencer 1989). The geometry was determined by the heliocentric distance and the "AKARI-centric" distance and phase angle. The fitted values of the beaming parameter for each individual filter, η = 0.87 for S9W and 0.77 for L18W, were utilized to reduce systematic errors. The absolute magnitude Hv (in visual) and the phase slope parameter G were taken from the Lowell Observatory,10 and the uncertainties were assigned as 0.05 mag and 0.02, respectively. Combined with the observed fluxes, typical uncertainties in diameter of 4.7% and those in geometric albedo of 10.1% were computed. The detail procedures of the data reduction, the analysis, and the catalog list are described in Usui et al. (2011).
A total of 105 Cybele asteroids were observed by AKARI (into the AcuA). The circumstances are given in Table 1. The corresponding detectable size was D ⩾ 15 km (Usui et al. 2011). Previously, IRAS (Neugebauer et al. 1984) measured 70 Cybele asteroids (Tedesco et al. 2002). The AcuA includes 68 Cybele asteroids in the IRAS data. Using objects common to both surveys, we examined their consistency. Both of the data sets agree quite well, showing the significant correlation coefficient 0.9803 for the diameter and 0.8487 for the geometric albedo (Figure 1). Another two Cybele asteroids ((4014) Heizman and (21078) 1991 RR16) were only observed by IRAS. Then, we added them to this work. Finally, we studied 107 Cybele asteroids. The diameters and geometric albedos are listed in Table 2.
Figure 1. Comparison of diameters and geometric albedos from AKARI with those from IRAS (Tedesco et al. 2002) for 68 Cybele asteroids common to both surveys. The equivalences of the two measurements are shown by diagonal lines. Their significant correlations indicate that the mid-IR data are robust.
Download figure:
Standard image High-resolution imageTable 1. Observation Log for Cybele Asteroids from the AKARI Survey
Number | Name | ProvDesa | Hvb | UT Datec | Rd | Δe | αf | Flux Density | Bandg |
---|---|---|---|---|---|---|---|---|---|
(mag) | (AU) | (AU) | (deg) | (Jy) | |||||
65 | Cybele | 6.62 | 2006 Dec 26 17:04 | 3.414 | 3.279 | 16.75 | 5.038 ± 0.325 | S9W | |
65 | Cybele | 6.62 | 2006 Dec 27 04:39 | 3.414 | 3.272 | 16.75 | 5.061 ± 0.326 | S9W | |
65 | Cybele | 6.62 | 2007 Jun 21 04:23 | 3.246 | 3.086 | 18.23 | 7.928 ± 0.501 | S9W | |
65 | Cybele | 6.62 | 2007 Jun 21 06:02 | 3.246 | 3.087 | 18.23 | 7.914 ± 0.500 | S9W | |
65 | Cybele | 6.62 | 2007 Jun 21 14:19 | 3.246 | 3.092 | 18.23 | 41.488 ± 2.780 | L18W | |
65 | Cybele | 6.62 | 2007 Jun 21 15:59 | 3.246 | 3.093 | 18.23 | 42.159 ± 2.825 | L18W | |
76 | Freia | 7.90 | 2006 Jun 28 20:20 | 3.504 | 3.363 | 16.87 | 10.629 ± 0.714 | L18W | |
76 | Freia | 7.90 | 2006 Jun 28 21:59 | 3.504 | 3.362 | 16.87 | 10.802 ± 0.726 | L18W | |
76 | Freia | 7.90 | 2006 Jun 29 06:15 | 3.503 | 3.357 | 16.88 | 1.637 ± 0.107 | S9W | |
76 | Freia | 7.90 | 2006 Jun 29 07:54 | 3.503 | 3.356 | 16.88 | 1.619 ± 0.105 | S9W | |
76 | Freia | 7.90 | 2006 Dec 23 07:10 | 3.234 | 3.084 | 17.70 | 2.295 ± 0.145 | S9W | |
76 | Freia | 7.90 | 2006 Dec 23 08:49 | 3.234 | 3.085 | 17.70 | 2.254 ± 0.142 | S9W | |
76 | Freia | 7.90 | 2006 Dec 23 17:06 | 3.234 | 3.089 | 17.70 | 12.845 ± 0.861 | L18W | |
76 | Freia | 7.90 | 2006 Dec 23 18:45 | 3.233 | 3.090 | 17.70 | 12.883 ± 0.863 | L18W | |
76 | Freia | 7.90 | 2006 Dec 24 01:22 | 3.233 | 3.094 | 17.70 | 12.483 ± 0.836 | L18W | |
76 | Freia | 7.90 | 2006 Dec 24 03:02 | 3.233 | 3.095 | 17.70 | 12.422 ± 0.832 | L18W | |
76 | Freia | 7.90 | 2006 Dec 24 06:20 | 3.233 | 3.097 | 17.70 | 12.151 ± 0.814 | L18W |
Notes. Slope parameters G = 0.01, 0.08, and 0.15 were used for (65) Cybele, (107) Camilla, and other Cybele asteroids, respectively (from the Lowell Observatory). aAsteroid provisional designation. bAbsolute visual magnitude from the Lowell Observatory. cInterpolated time from Murakami et al. (2007). dHeliocentric distance. eAKARI-centric distance. fAKARI-centric phase angle. gFilter type. S9W=9 μm band and L18W=18 μm band.
Only a portion of this table is shown here to demonstrate its form and content. Machine-readable and Virtual Observatory (VO) versions of the full table are available.
Download table as: Machine-readable (MRT)Virtual Observatory (VOT)Typeset image
Table 2. Physical and Orbital Properties of 107 Cybele Asteroids
Number | Name | ProvDesa | NIDb | Dc | pvd | ae | ef | ig | Taxonomyh |
---|---|---|---|---|---|---|---|---|---|
(km) | (AU) | (deg) | |||||||
65 | Cybele | 6 | 301 ± 5 | 0.044 ± 0.002 | 3.432 | 0.108 | 3.563 | P | |
76 | Freia | 11 | 168 ± 2 | 0.043 ± 0.001 | 3.415 | 0.163 | 2.117 | P | |
87 | Sylvia | 7 | 263 ± 4 | 0.043 ± 0.002 | 3.485 | 0.081 | 10.858 | P | |
107 | Camilla | 5 | 200 ± 4 | 0.065 ± 0.003 | 3.479 | 0.076 | 10.051 | P | |
121 | Hermione | 8 | 194 ± 3 | 0.058 ± 0.002 | 3.445 | 0.136 | 7.598 | C | |
168 | Sibylla | 10 | 146 ± 2 | 0.055 ± 0.002 | 3.378 | 0.068 | 4.635 | C | |
225 | Henrietta | 8 | 108 ± 2 | 0.051 ± 0.002 | 3.389 | 0.265 | 20.873 | C | |
229 | Adelinda | 12 | 109 ± 1 | 0.034 ± 0.001 | 3.417 | 0.141 | 2.081 | C | |
260 | Huberta | 7 | 95 ± 1 | 0.054 ± 0.002 | 3.444 | 0.117 | 6.417 | P | |
319 | Leona | 10 | 66 ± 1 | 0.051 ± 0.002 | 3.407 | 0.221 | 10.565 | P | |
401 | Ottilia | 1895 BT | 6 | 94 ± 1 | 0.046 ± 0.002 | 3.345 | 0.039 | 5.971 | |
414 | Liriope | 1896 CN | 7 | 77 ± 1 | 0.047 ± 0.002 | 3.503 | 0.069 | 9.544 | C |
420 | Bertholda | 1896 CY | 5 | 142 ± 3 | 0.042 ± 0.002 | 3.429 | 0.037 | 6.637 | P |
466 | Tisiphone | 1901 FX | 7 | 102 ± 1 | 0.082 ± 0.003 | 3.358 | 0.089 | 19.104 | C |
483 | Seppina | 1902 HU | 12 | 70 ± 1 | 0.172 ± 0.004 | 3.425 | 0.055 | 18.743 | S |
522 | Helga | 1904 NC | 11 | 101 ± 1 | 0.039 ± 0.001 | 3.627 | 0.076 | 4.437 | P |
528 | Rezia | 1904 NS | 9 | 85 ± 1 | 0.057 ± 0.002 | 3.400 | 0.019 | 12.675 | |
536 | Merapi | 1904 OF | 5 | 146 ± 3 | 0.049 ± 0.002 | 3.504 | 0.082 | 19.378 | P |
566 | Stereoskopia | 1905 QO | 9 | 177 ± 2 | 0.035 ± 0.001 | 3.384 | 0.112 | 4.898 | C |
570 | Kythera | 1905 QX | 5 | 99 ± 2 | 0.055 ± 0.002 | 3.421 | 0.122 | 1.785 | D |
643 | Scheherezade | 1907 ZZ | 6 | 71 ± 1 | 0.046 ± 0.002 | 3.354 | 0.064 | 13.767 | P |
713 | Luscinia | 1911 LS | 8 | 97 ± 1 | 0.048 ± 0.002 | 3.391 | 0.166 | 10.356 | C |
721 | Tabora | 1911 MZ | 7 | 82 ± 1 | 0.053 ± 0.002 | 3.553 | 0.110 | 8.348 | D |
733 | Mocia | 1912 PF | 7 | 97 ± 1 | 0.045 ± 0.002 | 3.397 | 0.063 | 20.265 | C |
790 | Pretoria | 1912 NW | 2 | 145 ± 5 | 0.053 ± 0.004 | 3.409 | 0.151 | 20.525 | P |
909 | Ulla | 1919 FA | 11 | 113 ± 1 | 0.037 ± 0.001 | 3.546 | 0.093 | 18.800 | P |
940 | Kordula | 1920 HT | 5 | 88 ± 2 | 0.035 ± 0.002 | 3.361 | 0.178 | 6.213 | C |
1004 | Belopolskya | 1923 OS | 6 | 80 ± 1 | 0.028 ± 0.001 | 3.402 | 0.084 | 2.979 | P |
1028 | Lydina | 1923 PG | 8 | 97 ± 1 | 0.032 ± 0.001 | 3.410 | 0.113 | 9.363 | C |
1091 | Spiraea | 1928 DT | 6 | 41 ± 1 | 0.063 ± 0.003 | 3.423 | 0.061 | 1.156 | |
1154 | Astronomia | 1927 CB | 7 | 64 ± 1 | 0.028 ± 0.001 | 3.385 | 0.074 | 4.559 | P |
1167 | Dubiago | 1930 PB | 11 | 76 ± 1 | 0.036 ± 0.001 | 3.412 | 0.077 | 5.721 | D |
1177 | Gonnessia | 1930 WA | 11 | 94 ± 1 | 0.040 ± 0.001 | 3.347 | 0.030 | 15.069 | P |
1266 | Tone | 1927 BD | 8 | 89 ± 1 | 0.039 ± 0.001 | 3.358 | 0.047 | 17.184 | D |
1280 | Baillauda | 1933 QB | 11 | 54 ± 1 | 0.045 ± 0.001 | 3.411 | 0.052 | 6.461 | P |
1295 | Deflotte | 1933 WD | 4 | 46 ± 1 | 0.049 ± 0.003 | 3.397 | 0.116 | 2.875 | |
1328 | Devota | 1925 UA | 9 | 56 ± 1 | 0.043 ± 0.002 | 3.509 | 0.139 | 5.758 | D |
1390 | Abastumani | 1935 TA | 4 | 98 ± 2 | 0.033 ± 0.002 | 3.432 | 0.028 | 19.989 | P |
1467 | Mashona | 1938 OE | 7 | 95 ± 1 | 0.074 ± 0.002 | 3.381 | 0.134 | 21.966 | C |
1556 | Wingolfia | 1942 AA | 2 | 34 ± 2 | 0.093 ± 0.012 | 3.422 | 0.111 | 15.753 | P |
1574 | Meyer | 1949 FD | 4 | 61 ± 1 | 0.036 ± 0.002 | 3.533 | 0.038 | 14.517 | D |
1579 | Herrick | 1948 SB | 8 | 48 ± 1 | 0.041 ± 0.001 | 3.428 | 0.131 | 8.782 | C |
1796 | Riga | 1966 KB | 5 | 86 ± 2 | 0.028 ± 0.001 | 3.346 | 0.062 | 22.673 | C |
1841 | Masaryk | 1971 UO1 | 7 | 44 ± 1 | 0.045 ± 0.002 | 3.420 | 0.098 | 2.621 | P |
2196 | Ellicott | 1965 BC | 6 | 58 ± 1 | 0.043 ± 0.002 | 3.441 | 0.052 | 10.303 | C |
2208 | Pushkin | 1977 QL3 | 6 | 44 ± 1 | 0.038 ± 0.002 | 3.515 | 0.039 | 5.387 | D |
2266 | Tchaikovsky | 1974 VK | 7 | 44 ± 1 | 0.045 ± 0.002 | 3.394 | 0.185 | 13.243 | D |
2311 | El Leoncito | 1974 TA1 | 6 | 52 ± 1 | 0.042 ± 0.002 | 3.632 | 0.043 | 6.620 | D |
2634 | James Bradley | 1982 DL | 5 | 36 ± 1 | 0.115 ± 0.006 | 3.451 | 0.054 | 6.404 | P |
2697 | Albina | 1969 TC3 | 9 | 53 ± 1 | 0.053 ± 0.002 | 3.560 | 0.082 | 3.580 | P |
2702 | Batrakov | 1978 SZ2 | 5 | 26 ± 1 | 0.065 ± 0.004 | 3.441 | 0.090 | 1.576 | |
2891 | McGetchin | 1980 MD | 9 | 37 ± 1 | 0.043 ± 0.002 | 3.360 | 0.129 | 9.331 | D |
2932 | Kempchinsky | 1980 TK4 | 1 | 23 ± 2 | 0.079 ± 0.013 | 3.621 | 0.113 | 2.279 | |
2976 | Lautaro | 1974 HR | 6 | 45 ± 1 | 0.048 ± 0.002 | 3.343 | 0.144 | 9.807 | |
3024 | Hainan | 1981 UW9 | 8 | 40 ± 1 | 0.058 ± 0.002 | 3.421 | 0.123 | 14.775 | |
3092 | Herodotus | 6550 P-L | 7 | 34 ± 1 | 0.064 ± 0.003 | 3.535 | 0.122 | 10.941 | |
3095 | Omarkhayyam | 1980 RT2 | 4 | 32 ± 1 | 0.055 ± 0.004 | 3.499 | 0.069 | 2.987 | D |
3141 | Buchar | 1984 RH | 5 | 40 ± 1 | 0.069 ± 0.003 | 3.399 | 0.077 | 11.001 | D |
3273 | Drukar | 1975 TS2 | 4 | 34 ± 1 | 0.045 ± 0.003 | 3.405 | 0.032 | 14.056 | |
3368 | Duncombe | 1985 QT | 4 | 35 ± 1 | 0.044 ± 0.003 | 3.394 | 0.091 | 19.152 | |
3396 | Muazzez | A915 TE | 2 | 36 ± 2 | 0.053 ± 0.007 | 3.384 | 0.187 | 8.352 | D |
3622 | Ilinsky | 1981 SX7 | 1 | 21 ± 2 | 0.107 ± 0.016 | 3.389 | 0.036 | 4.948 | D |
3675 | Kemstach | 1982 YP1 | 1 | 17 ± 1 | 0.234 ± 0.041 | 3.361 | 0.094 | 10.859 | S |
3727 | Maxhell | 1981 PQ | 8 | 31 ± 1 | 0.052 ± 0.002 | 3.320 | 0.146 | 5.310 | D |
3845 | Neyachenko | 1979 SA10 | 1 | 25 ± 2 | 0.059 ± 0.008 | 3.430 | 0.164 | 5.960 | P |
4003 | Schumann | 1964 ED | 5 | 35 ± 1 | 0.072 ± 0.004 | 3.422 | 0.097 | 5.018 | D |
4169 | Celsius | 1980 FO3 | 5 | 38 ± 1 | 0.057 ± 0.003 | 3.390 | 0.173 | 10.052 | D |
4236 | Lidov | 1979 FV1 | 2 | 30 ± 2 | 0.061 ± 0.009 | 3.445 | 0.030 | 7.294 | |
4973 | Showa | 1990 FT | 4 | 31 ± 1 | 0.057 ± 0.004 | 3.428 | 0.077 | 18.896 | D |
5301 | Novobranets | 1974 SD3 | 3 | 25 ± 1 | 0.091 ± 0.010 | 3.362 | 0.100 | 10.027 | P |
5362 | 1978 CH | 2 | 22 ± 2 | 0.093 ± 0.014 | 3.388 | 0.019 | 6.153 | D | |
5495 | Rumyantsev | 1972 RY3 | 3 | 26 ± 1 | 0.108 ± 0.012 | 3.430 | 0.045 | 9.203 | D |
5780 | Lafontaine | 1990 EJ2 | 2 | 24 ± 2 | 0.061 ± 0.009 | 3.350 | 0.123 | 8.685 | D |
5833 | Peterson | 1991 PQ | 6 | 34 ± 1 | 0.080 ± 0.004 | 3.491 | 0.029 | 19.325 | P |
5914 | Kathywhaler | 1990 WK | 4 | 39 ± 1 | 0.056 ± 0.004 | 3.554 | 0.089 | 10.357 | D |
6039 | Parmenides | 1989 RS | 1 | 25 ± 2 | 0.088 ± 0.012 | 3.410 | 0.059 | 13.110 | D |
6057 | Robbia | 5182 T-3 | 3 | 30 ± 1 | 0.073 ± 0.006 | 3.321 | 0.103 | 17.861 | P |
6103 | 1993 HV | 8 | 32 ± 1 | 0.033 ± 0.001 | 3.416 | 0.205 | 14.194 | ||
6574 | Gvishiani | 1976 QE1 | 6 | 30 ± 1 | 0.048 ± 0.002 | 3.400 | 0.191 | 17.710 | D |
6924 | Fukui | 1993 TP | 4 | 33 ± 1 | 0.055 ± 0.004 | 3.389 | 0.099 | 12.212 | P |
7466 | 1989 VC2 | 3 | 22 ± 1 | 0.059 ± 0.006 | 3.350 | 0.140 | 6.410 | ||
7501 | Farra | 1996 VD3 | 1 | 21 ± 1 | 0.052 ± 0.007 | 3.330 | 0.110 | 1.549 | |
7574 | 1989 WO1 | 3 | 24 ± 1 | 0.099 ± 0.011 | 3.441 | 0.173 | 11.109 | ||
8482 | 1988 RA11 | 1 | 16 ± 1 | 0.038 ± 0.006 | 3.336 | 0.082 | 3.106 | C | |
8917 | 1996 EU2 | 7 | 40 ± 1 | 0.035 ± 0.001 | 3.413 | 0.112 | 15.464 | ||
9402 | 1994 UN1 | 4 | 22 ± 1 | 0.045 ± 0.003 | 3.353 | 0.115 | 9.953 | ||
9552 | 1985 UY | 1 | 18 ± 2 | 0.065 ± 0.013 | 3.441 | 0.086 | 9.962 | D | |
11188 | 1998 KD50 | 2 | 16 ± 2 | 0.066 ± 0.013 | 3.380 | 0.082 | 11.427 | ||
12003 | Hideosugai | 1996 FM5 | 6 | 23 ± 1 | 0.045 ± 0.003 | 3.357 | 0.095 | 9.864 | C |
13096 | Tigris | 1993 BE5 | 1 | 15 ± 2 | 0.097 ± 0.028 | 3.651 | 0.025 | 2.265 | |
13832 | 1999 XR13 | 5 | 42 ± 1 | 0.065 ± 0.004 | 3.373 | 0.115 | 16.149 | D | |
17161 | 1999 LQ13 | 6 | 19 ± 1 | 0.047 ± 0.004 | 3.346 | 0.165 | 6.637 | C | |
18150 | Lopez-Moreno | 2000 OC60 | 1 | 22 ± 2 | 0.044 ± 0.008 | 3.444 | 0.096 | 12.771 | P |
20718 | 1999 XZ97 | 1 | 24 ± 2 | 0.052 ± 0.007 | 3.444 | 0.111 | 11.728 | C | |
23025 | 1999 WR9 | 4 | 21 ± 1 | 0.038 ± 0.004 | 3.439 | 0.089 | 6.887 | C | |
23782 | 1998 QE12 | 2 | 16 ± 1 | 0.069 ± 0.013 | 3.411 | 0.097 | 9.281 | C | |
26607 | 2000 FA33 | 2 | 20 ± 2 | 0.028 ± 0.005 | 3.392 | 0.052 | 16.442 | C | |
29538 | 1998 BN16 | 2 | 25 ± 2 | 0.076 ± 0.012 | 3.401 | 0.049 | 14.631 | ||
31822 | 1999 SY4 | 5 | 24 ± 1 | 0.055 ± 0.004 | 3.414 | 0.042 | 6.369 | ||
52706 | 1998 FO77 | 1 | 25 ± 2 | 0.020 ± 0.003 | 3.498 | 0.057 | 9.469 | ||
73983 | 1998 DS19 | 1 | 14 ± 1 | 0.060 ± 0.013 | 3.339 | 0.117 | 15.575 | ||
74487 | 1999 CE105 | 1 | 17 ± 2 | 0.082 ± 0.016 | 3.369 | 0.027 | 20.916 | ||
77735 | 2001 OJ76 | 1 | 15 ± 2 | 0.020 ± 0.005 | 3.527 | 0.089 | 12.276 | D | |
83923 | 2001 VR16 | 1 | 14 ± 2 | 0.048 ± 0.012 | 3.391 | 0.116 | 11.584 | ||
99167 | 2001 FX151 | 2 | 14 ± 1 | 0.055 ± 0.011 | 3.411 | 0.180 | 17.212 | P | |
4014i | Heizman | 1979 SG10 | ⋅⋅⋅ | 37 ± 6 | 0.021 ± 0.009 | 3.425 | 0.033 | 1.097 | P |
21078i | 1991 RR16 | ⋅⋅⋅ | 26 ± 2 | 0.022 ± 0.004 | 3.411 | 0.085 | 8.068 |
Notes. Diameters, geometric albedos, and taxonomic data are provided by Usui et al. (2011, AcuA). Orbital data are from the Lowell Observatory. aAsteroid provisional designation. bNumber of detections by AKARI (Usui et al. 2011). cDiameter. dGeometric albedo in optical. eSemimajor axis. fEccentricity. gInclination. hFrom Appendix 4 in Usui et al. (2011). (Sources: Tholen 1984; Bus 1999; Lazzaro et al. 2004; Carvano et al. 2010.) Here, Gil-Hutton & Licandro (2010) are added. iIRAS data from Tedesco et al. (2002).
3. RESULTS
3.1. Diameter and Geometric Albedo
Figure 2 shows a plot of diameter versus geometric albedo. We find that 98% of the population has low geometric albedos pv ⩽ 0.11 in the range of D = 10–300 km. A little spread of geometric albedos is confirmed by decreasing diameters. This is more readily displayed in Figure 3, where the histograms of the size-dependent populations are compared. At the top panel, we find that smaller diameters of Cybeles (10 km <D < 50 km) have a slightly wider range of a mean geometric albedo than those of the middle (
) and bottom (
) panels. Note that geometric albedos of a much smaller diameter range (D < 10 km) are arguable. Using Spitzer observations, the much smaller Hildas and Trojans show the very wide spread of geometric albedos from 0.01 up to 0.35 (Fernández et al. 2009; Ryan & Woodward 2011). Contrary to this, according to the WISE preliminary results, Spitzer suffers from observing selection biases (Mainzer et al. 2011; Masiero et al. 2011; Grav et al. 2011, 2012). AKARI had sensitivity that was sufficient enough to detect objects with diameters larger than 15 km around the Cybele region (Usui et al. 2011). In this work, although four Cybele asteroids are at the critical detection limit ((73983) 1998 DS19 with D = 13.64 ± 1.44 km, (99167) 2001 FX151 with D = 13.75 ± 1.18 km, (83923) 2001 VR16 with D = 14.48 ± 1.75 km, and (77735) 2001 OJ76 with D = 14.93 ± 1.83 km), those geometric albedos show modest values (0.02–0.06) with ∼20% of errors. Hence, their observing biases here should be negligible.
Figure 2. Scatter plot of the diameters and geometric albedos for 107 Cybele asteroids.
Download figure:
Standard image High-resolution imageFigure 3. Histograms of the size-dependent geometric albedos. From the top to bottom panels, the number of objects is 65, 27, and 15, respectively. These differences are real.
Download figure:
Standard image High-resolution imageThe left panel of Figure 4 presents the distribution of the diameters. Approximately 61% of the Cybele asteroids are in the diameter range 10 km <D < 50 km, 25% are 50 km <D < 100 km, and 14% are 100 km <D. Asteroid (65) Cybele has the largest diameter, D = 301 ± 5 km, in the family. The right panel of Figure 4 exhibits the case for the geometric albedos, which has an average and covers 105 members within the 2σ level of significance. Two of the largest albedos, pv = 0.234 ± 0.041 and 0.172 ± 0.004, come from S-type asteroids 3675 Kemstach and 483 Seppina, respectively (see Section 3.4).
Figure 4. Distributions of diameters (left) and geometric albedos (right).
Download figure:
Standard image High-resolution image3.2. Orbital Properties
Figure 5 depicts population graphs against various orbital elements a, i, e, and perihelion distance q. The a-graph (Figure 5(a)) has about 77% of the population in a mean range AU. As a increases on the far side of the
, numbers of each bin drop off down to several percent or less of the entire group (Δa = 0.05 AU). Figure 5(b) shows the i-graph with an average value
. We find the moderate uniformity. For the Trojans case, a possible bimodal feature of the i-graph separated at a gap of i ∼ 13° is debatable because this could be taken as a sign of their collisional origin (Degewij & van Houten 1979; Jewitt et al. 2000; Yoshida & Nakamura 2005). The Cybeles do not have this kind of tendency. No trend of the e-graph (Figure 5(c)) is readily apparent (
). The q-graph is pictured in Figure 5(d). Nearly 3% of the Cybele population goes inside the heliocentric distance of ∼2.7 AU, where MBCs show cometary activities (Hsieh et al. 2004). C-type asteroid (225) Henrietta (D ∼ 100 km) has a small q that comes inside at the limit to the snow line (<2.5 AU), estimated by the known distribution of MBCs (Sonnett et al. 2011). No active Cybele asteroids have yet been observed.
Figure 5. Population as a function of various orbital parameters of the Cybeles, (a) semimajor axis, (b) inclination, ( c ) eccentricity, and (d) perihelion distance.
Download figure:
Standard image High-resolution image3.3. Size Distribution
Figure 6 presents the cumulative size distribution (CSD) of the 107 Cybeles to examine the footprint of the collisional evolution. We assumed that the diameters of the Cybeles follow a power-law distribution with a slope index b. The function can be approximated to be the form N(> D) = C · D−b, where N is the cumulative number of asteroids larger than D and C is a constant. If the bodies are uniform in material (i.e., have the same strength per unit mass) and respond in a size-independent way, the equilibrium power-law index is found to be b = 2.5 (Dohnanyi 1969). The error bars for the CSDs were estimated from Poisson statistics. The D-bin size was set to be 10 km to cover an averaged diameter and its uncertainty of objects allocated. The data in Figure 6 visibly display two breaks at D ∼ 20 km and ∼80 km. Such breaks (kinks) in the size distribution are also found in the Trojans and the main belt asteroids (Jedicke & Metcalfe 1998; Durda et al. 1998; Fernández et al. 2009). Then, the Cybele data were adequately fitted by the following three CSD functions:
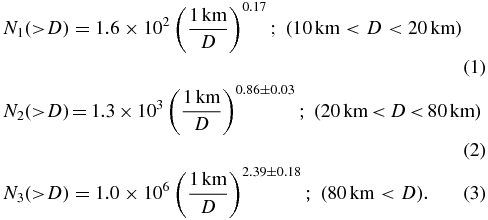
The flatter slope index value b = 0.17 is obtained by 12 small objects in the range of D = 10–20 km (straight line between two points in Figure 6). Sixty-six objects with diameters 20 km <D < 80 km show the index b = 0.86 ± 0.03, which is slightly steeper than that of the smaller objects. Twenty-nine objects with D > 80 km have the steepest power-law index b = 2.39 ± 0.18, which is near the equilibrium b = 2.5 distribution produced by collisional shattering (Dohnanyi 1969). The most straightforward explanation of the power-law indexes in Equations (1)–(3) is that the large Cybeles with D > 80 km are products from self-similar collision cascades of larger precursor bodies, while objects with 20 km <D < 80 km represent a primordial population. By contrast, the flatter index (b = 0.17) may have little physical significance because of the observational selection against small objects. The corresponding differential size distributions are
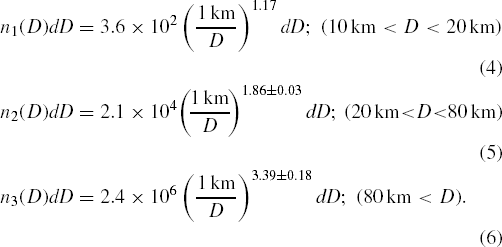
The total mass of Cybele MCybele can be estimated by integrating over the size distributions. As we noted above, the small size range of D < 15 km may suffer from selection biases. To avoid this (negligibly small ΔMCybele ∼ 3%, though), Equation (4) (D < 20 km) was not applied; instead, Equation (5) was extrapolated down to D = 0 km. We then obtained

where n2 and n3 are from Equations (5) and (6), Dc ∼ 77 km is a diameter at which the two CSDs are equal (Equation (2) = Equation (3)), Dmax ∼ 300 km is taken from the largest member (65) Cybele, and ρ = 1400 kg m−3 is the measured bulk density from the Cybele binary asteroid (121) Hermione (Marchis et al. 2009). We find MCybele ≈ 6.5 × 1019 kg ≈10−5 MEarth (MEarth = 6.0 × 1024 kg), equivalent to a 440 km diameter sphere body with the same density (an assumed precursor body). This is about 1.5 times as large as (65) Cybele. For comparison, the total mass of Hildas is 6.0 × 10−6MEarth (≈0.6 MCybele) for a sphere body with D ∼ 330 km (Ryan & Woodward 2011), and the total mass of the Trojans is 9 × 10−5MEarth (≈9 MCybele) for a sphere body with D ∼ 800 km (L4; Jewitt et al. 2000).
Figure 6. Cumulative size distribution of 107 Cybele asteroids. The derived power-law indexes are b = 0.17 (10 km <D < 20 km), 0.86 ± 0.03 (20 km <D < 80 km), and 2.39 ± 0.18 (D > 80 km).
Download figure:
Standard image High-resolution image3.4. Taxonomy
Figures 7 and 8 plot histograms of the diameters and geometric albedos for different taxonomic types. In this work, 80 Cybeles (75%) have their taxonomic information. As described in Appendix 4 of Usui et al. (2011), they are determined by compiling data provided by Tholen (1984), Bus (1999), Lazzaro et al. (2004), and Carvano et al. (2010). In this study, the SDSS-MOC result from Gil-Hutton & Licandro (2010) is taken together. The types are shown in Column 10 of Table 2.
Figure 7. Histograms of diameters for 80 Cybeles in the different taxonomic types (22 C-types, 27 D-types, 29 P-types, and two S-types). The C- and P-type Cybeles are dominant at larger sizes (D > 80 km). The taxonomic diversity of the smaller Cybeles is confirmed.
Download figure:
Standard image High-resolution imageFigure 8. Same as Figure 7, but for geometric albedos. The C-, D-, and P-type Cybeles present low values of pv ⩽ 0.11.
Download figure:
Standard image High-resolution imageFigure 7 shows the histograms of the diameters for C-, D-, P-, and S-type asteroids. From 10 to 50 km, 50% of the objects are D-types. C- and P-types are 20% and 28%, respectively. For the size range 50–100 km, C-, D-, and P-types are similarly 32%, 28%, and 36%, respectively. Both C- and P-types are relatively uniformly distributed over the entire 10–100 km range. Any changes in the overall proportions are caused by the large number of small D-types. For D = 100–200 km, the statistics are small and do not allow any interpretation beyond C- and P-types being roughly equal. All of the largest objects (D > 200 km) are P-types.
Hereafter, we use the break at D ∼ 80 km of the CSD to relate to the power-law indexes (see Sections 3.3 and 4). For large diameters D > 80 km, we find that the C- and P-type Cybeles are dominant (90%). By contrast, for small diameters D < 80 km, numerous D- and two S-type Cybeles are also found. The small Cybeles are mainly classified into D-type (45%), P-type (32%), and C-type (19%). For the large Cybeles, the trend presented here is consistent with previous works, including the spectroscopy (Lagerkvist et al. 2005) and the SDSS-MOC results (Gil-Hutton & Licandro 2010). But, for the small ones, we find conflict between them. Although the D-type dominance was reported (>70%; Lagerkvist et al. 2005), the taxonomic variety was also found (Gil-Hutton & Licandro 2010). Our study obviously supports the latter. The difference may be mostly caused by sampling biases. The former only has 20 Cybeles, but, for the latter, we have more than 80 Cybeles. Accordingly, we can conclude that the small Cybeles essentially have the taxonomic diversity.
Figure 8 represents the histograms of the geometric albedos for various taxonomic asteroids. An average is = 0.047 ± 0.014 for C-type, 0.058 ± 0.021 for D-type, 0.053 ± 0.021 for P-type, and 0.203 ± 0.044 for S-type. The C-type Cybeles are comparatively close to the typical value of cometary nuclei pv ∼ 0.04 (Jewitt 2004). The D- and P-type Cybeles have nearly the same mean value. They are slightly larger than that of the C-type, but those three are well consistent with a 1σ level of confidence. The C-, D-, and P-type asteroids similarly show the widespread distribution shapes of pv = 0.02–0.11. The two S-type asteroids have higher albedos than the other types.
4. DISCUSSION
The small Cybeles (D < 80 km) show the taxonomic diversity. There are numerous D-types, plus moderate fractions of P- and C-types (Figure 7). The SDSS-MOC study also reports three taxonomic types for the small ones that are not overconcentrated on D-types (Gil-Hutton & Licandro 2010). The majority of D-type asteroids with small diameters were confirmed in both the Hildas and Trojans, which have been considered either primordial properties or, preferably, possible remnants of their collisional histories (Jewitt & Luu 1990; Fitzsimmons et al. 1994; Dahlgren & Lagerkvist 1995; Dahlgren et al. 1997). For the small Cybeles, however, three types of taxonomies (D-, P-, and C-types) are applicable to the hypothesis. Their physical connections are notable. First, the spectra of P-type asteroids are less red than the D-type asteroids. Lagerkvist et al. (2005) suggest that this might just result from neutralizing D-type spectra slopes by various factors, for example, heating by the Sun (Dahlgren et al. 1997) and space weathering (Moroz et al. 2004). Second, Fornasier et al. (2011) study the possible composition of the asteroids using the RELAB spectral database. They find that spectra of Carbonaceous Mighei (CM) meteorites are well fitted with low albedo P- and C-type asteroids that have been exposed to either heat or irradiation. This is a similar case found in the relation between P- and D-types. Third, the spectral gradients of C- and D-types are well consistent with those of cometary nuclei (Jewitt 2004). Compositionally, they are primordially equivalent in nature, and, besides, the difference is simply due to sublimation-driven evolution (Jewitt 2002; Hsieh et al. 2009). Altogether, the small D-, P-, and C-type Cybeles may be closely associated physically. Meanwhile, their power-law index b = 0.86 ± 0.03 has no consistency with Dohnanyi's value (b ∼ 2.5; Dohnanyi 1969). If we follow the rule, they are unlikely to be products after cascade collisions.
By contrast, the C- and P-type Cybele asteroids are dominant at large diameters. The CSD with D > 80 km consists of approximately 90% of those objects with the power-law index b = 2.39 ± 0.18 (Figure 6). This result implies that the large C- and P-type Cybeles could be collisionally produced fragments of larger bodies (b ∼ 2.5; Dohnanyi 1969). Here, for consistency with the published literature, we should note that the equilibrium index is statistically insignificant (Durda & Dermott 1997; Jedicke & Metcalfe 1998; Durda et al. 1998; Jewitt et al. 2000; Fernández et al. 2003, 2009; Yoshida & Nakamura 2005; Szabó et al. 2007; Wiegert et al. 2007; O'Brien & Greenberg 2003, 2005; O'Brien et al. 2007). The index is only applicable to self-similar collision cascades in which the impact strengths are independent of target size, but, in reality, the strength is strongly size dependent (Davis et al. 2002, and references therein). This fact leads us to expect that catastrophic collisional impacts can produce fragments with non-Dohnanyi indexes. Well-known examples include the Hildas and Trojans. Observationally, their power-law indexes apparently exhibit the wide range of b = 0.4–4.5. Nevertheless, their collisional origins are proposed. For the Hildas, Ryan & Woodward (2011) gave b ∼ 0.4 (5 km ⩽ D ⩽ 12 km) and ∼2.0 (12 km ⩽ D ⩽ 180 km). For the Trojans, Jewitt et al. (2000) found b ∼ 2.0 (4.4 km ⩽ D ⩽ 40 km) and ∼4.5 (D ⩾ 84 km). Also, Fernández et al. (2009) derived b ∼ 2.0 (1 km ⩽ D ⩽ 5 km), ∼0.8 (5 km ⩽ D ⩽ 35 km), and ∼3.8 (D ⩾ 35 km). As for Trojans with D < 10 km, Yoshida & Nakamura (2005) and Szabó et al. (2007) likewise obtained similar values b ∼ 1.9–2.2. Using simulation, Levison et al. (2009) also suggest that the two groups originate from collisional grindings (for example, Trojans: the b ∼ 1.8 for the D < 105 km and b ∼ 5.5 for the D > 105 km, respectively). In the studies above, the equilibrium index is partly responsible for the collisional histories; nonetheless, evidently it is not always essential. Under these circumstances, a comparison of the indexes between actual observation and simulation may be useful. Unfortunately, the Cybeles are unfocused using the recent Nice model (Levison et al. 2009). Therefore, the power-law index here is not a unique indicator of the past process.
To proceed, we examined a collisional timescale between the asteroids in the Cybele region. Some 100 km scale asteroids may have survived relatively undamaged since the time of their creation because their collisional lifetimes are estimated to be the same order of ∼109 yr as the age of the solar system (4.6 × 109 yr; Dohnanyi 1969; Bell et al. 1989). Assuming that a precursor body of the Cybeles had experienced catastrophic impacts by projectiles of diameter Dp, the interval between collisions, τc, can be derived from (Davis et al. 2002)

where Dt is the diameter of the precursor body (target), Dp is the diameter of projectiles, PCybele is the collision probability per unit area in the Cybele region, and Np(⩾ Dp) is the cumulative number of projectiles larger than Dp. Substituting Dt = 440 km (see Section 3.3), Dp = 50–100 km (Dahlgren 1998), PCybele ⩽ 2.0 × 10−17 km−2 yr−1 (Dahlgren 1998; Dp ⩾ 50 km at AU in Figure 5), and Np(⩾ 50–100) ∼ 45–17 (Equations (2) and (3)), we find τc ⩾ 109–1010 yr. The result is longer than the age of the solar system. To assess this accuracy, we also computed individual τc of the Hildas and Trojans because they are thought to be collisionally shattered populations. Equation (8) is then applied to both groups. For the Hildas, we took Dt = 330 km, Np(⩾ 50–100) ∼ 37–9 from Ryan & Woodward (2011), and PHilda ∼ 1.6 × 10−17 km−2 yr−1 (Dahlgren 1998; Dp ⩾ 50 km). For the Trojans, we used Dt = 800 km, Np(⩾ 50–70) ∼ 256–130 (Equation (10)), Np(⩾ 80–100) ∼ 47–17 (Equation (11)) in Jewitt et al. (2000), and PTrojan ∼ 1.1 × 10−17 km−2 yr−1 (Dahlgren 1998; Dp ⩾ 50 km). Substitutions give τc ⩾ 1010 yr for the Hildas and τc ⩾ 108–109 yr for the Trojans, respectively. These estimates indicate that the Hildas may be survivors of a primordial population, while the Trojans have the potential to be products of past-shattering collisions. The former is apparently negative to the possible collisional origin (also see Brož & Vokrouhlický 2008); by contrast, the latter is positive. However, this contradiction is not allowed. Hence, these results estimated here could be insufficiently verifiable to be discussed. The collisional timescale of the Cybeles is also not an appropriate indicator. A future update for the collisional probability from Dahlgren (1998) may be warranted. The work includes the Cybeles, Hildas, Trojans, and main belt objects, but it does not consider possible interactions with the scattered TNOs into the outer main belt region (Levison et al. 2009). The effectiveness seems to create more frequent collisions (Brož et al. 2011). For this reason, the current collisional lifetime/environment may not have always been the same. To discuss in more detail, further progress into the understanding of the impact rate for the entire outer main belt will be helpful.
As a result, the large Cybeles (D > 80 km) could still be catastrophic collisional products because of the agreement with the Dohnanyi power-law index. Technically, the index can only apply to an equilibrium cascade. In other words, just because shattering collisions really occurred in the past does not always mean that the observed power-law index is consistent with the Dohnanyi one. So, what matters most is that we can simply relate an equilibrium-like observed index directly back to the possible collisional processes. There remains a gray zone between observation and Dohnanyi's model. To settle this argument, the next step is to couple observation with simulation approaches. That would be valuable to picture the origin and formation processes of the Cybele asteroid family. Until then, the argument is still open-ended.
5. SUMMARY
The Cybele asteroid family data, primarily from the AcuA, provide the following results:
- 1.The small Cybele asteroids with diameters 10 km <D < 80 km show taxonomic diversity (mainly C-, D-, and P-types). Their cumulative power-law size distribution index is 0.86 ± 0.03.
- 2.The large Cybeles with D > 80 km are mostly classified as C- or P-types (90%). The CSD has a power-law index of 2.39 ± 0.18.
- 3.The average geometric albedo of Cybele asteroids is
.
- 4.The distribution of inclinations of the Cybeles has a mean
. No bimodal trend is found.
- 5.The total mass of the Cybele asteroid family is about 6.5 × 1019 kg ≈ 10−5 MEarth, assuming a bulk density ρ = 1400 kg m−3.
This research is based on observations with AKARI Infrared Astronomical Satellite, a JAXA project with the participation of ESA. T.K. thanks Makoto Yoshikawa, David Jewitt, and Fumi Yoshida for comments. S.H. is supported by the Space Plasma Laboratory, ISAS, JAXA. T.O. is supported by Grants-in-Aid for Young Scientists (B) No. 21740153 and Scientific Research on Innovative Areas No. 21111005. M.I. is supported by the National Research Foundation of Korea. Finally, we are very grateful to the reviewer, Prof. Richard P. Binzel (MIT), for his constructive comments.
APPENDIX
We studied the physical and orbital properties of 107 Cybele asteroids. The objects, diameters, geometric albedos, orbital and taxonomic data are summarized in Table 2.
Footnotes
- 7
Here we note in the Tholen taxonomy that X-type is a temporary assignment for objects with a low slope and generally featureless visible spectra and whose albedos are unknown. There are three possible outcomes for assignment into a definitive taxonomic category: E-, M-, or P-type based on high, immediate, or low albedo values. Because albedos are available for the objects in this study, and because these albedos fall at low values, we are confident in breaking the spectral degeneracy and recognizing our observed objects to be P-types. For this reason, the P-type spectral classification is used throughout this paper.
- 8
The photometric data set is from the Moving Objects Catalog of the Sloan Digital Sky Survey (SDSS-MOC; Ivezić et al. 2001; Jurić et al. 2002). Trojan spectral data sets are taken by the Small Main-belt Asteroid Spectroscopic Survey (SMASS; Xu et al. 1995; Bus & Binzel 2002), the Small Solar System Objects Spectroscopic Survey (S3OS2; Lazzaro et al. 2004), Bendjoya et al. (2004), Fornasier et al. (2004), and Dotto et al. (2006).
- 9
Available at http://darts.jaxa.jp/ir/akari/catalogue/AcuA.html.
- 10
Available at ftp://ftp.lowell.edu/pub/elgb/astorb.html: Minor Planet Center.