The recent publication of the summary for policy makers by Working Group I of the Intergovernmental Panel on Climate Change (IPCC) [1] has injected a renewed sense of urgency to address climate change. It is therefore timely to review the notion of preventing 'dangerous anthropogenic interference with the climate system' as put forward in the United Nations Framework Convention on Climate Change (UNFCCC). The article by Danny Harvey in this issue [2] offers a fresh perspective by rephrasing the concept of 'dangerous interference' as a problem of risk assessment. As Harvey points out, identification of 'dangerous interference' does not require us to know with certainty that future climate change will be dangerous—an impossible task given that our knowledge about future climate change includes uncertainty. Rather, it requires the assertion that interference would lead to a significant probability of dangerous climate change beyond some risk tolerance, and therefore would pose an unacceptable risk.
In his article [2], Harvey puts this idea into operation by presenting a back-of-the-envelope calculation to identify allowable CO2 concentrations under uncertainty about climate sensitivity to anthropogenic forcing and the location of a temperature threshold beyond which dangerous climate change will occur. Conditional on his assumptions, Harvey delivers an interesting result. With the current atmospheric CO2 concentration exceeding 380 ppm, a forcing contribution from other greenhouse gases adding an approximate 100–110 ppm CO2 equivalent on top of it, and a global dimming effect of aerosols that roughly compensates for this contribution (albeit still subject to considerable uncertainty) ([1], figures SPM-1 and 2), we are on the verge of or even committed to dangerous interference with the climate system if we (1) set the risk tolerance for experiencing dangerous climate change to 1% and (2) allocate at least 5% probability to the belief that climate sensitivity is 4.5 °C or higher. In the language of the IPCC, the latter would mean that such a high climate sensitivity is anything but extremely unlikely ([1], footnote 6 and p 9), a view that is shared by many in the scientific community. Even if the risk tolerance is increased to 10%, the maximum allowable CO2 equivalent concentration remains below 460 ppm ([2], figure 7(c)). We are bound to reach that concentration in the near future, as it can be surpassed both by addition of new greenhouse gases and by a reduction of global dimming.
Given the potential significance of this result, let us take a step back, and investigate its underlying assumptions. The concept of 'dangerous anthropogenic interference' is inextricably linked to the idea of a threshold beyond which climate change can be labeled dangerous. This idea enters Harvey's analysis in the form of a probability distribution for the—as he calls it—'harm threshold' measured in terms of global mean temperature increase since preindustrial time. It might be due to the presumption of such a threshold that climate science and society at large have had a difficult relationship with the concept of 'dangerous anthropogenic interference' (Dessai et al [3]). Nevertheless, I want to argue here, as many have done before (see Oppenheimer and Petsonk [4] for an overview), that this concept is not ill-defined. First of all, it is clear that 'dangerous interference' and the stipulation of a 'harm threshold' carry a value judgment, and therefore cannot be decided upon purely by science. This does not prevent science, however, from providing information and conceptual frameworks to facilitate such judgment (Schellnhuber et al [5]). Secondly, it is certainly true that our interference with the climate system emanates from local and national action, and that the consequences of such interference will first and foremost be felt on the local to regional scale. However, this does not need to conflict with the assessment of a global 'harm threshold'. The nexus of climate policy is inevitably global since our interference with the climate system is determined by the sum total of greenhouse gas emissions. In addition, we are living in a highly interconnected world, and it would be foolish not to take global patterns of drivers and impacts of climate change into account. Finally, some may find the assumption of a harm threshold at odds with a cost–benefit approach, since the latter implies trading off avoided climate damage with the costs of mitigation measures. However, even if such a trade-off is made, harm thresholds will occur if damage rises sharply beyond some critical amount of climate change. A look into the climate history will convince us that this is not a far-fetched idea. Climate changes in the past have often exhibited highly non-linear behaviour (see figure 1). Although the paleoclimatic record does not provide a perfect analogue to the current situation, it offers little comfort that abrupt climate change in response to our massive and rapid increase of atmospheric CO2 concentrations might not happen in the future. Consequently, cost–benefit analyses accounting for the prospect of dangerous climate change have surfaced in recent years (e.g. Keller et al [6]). In addition, society has been prepared to set thresholds, e.g., to limit exposure to contaminants, even in situations where no clear jump in damage could be identified. And often such identification of thresholds was aided by cost–benefit analysis (e.g. Gurian et al [7]).
Is it appropriate to offer a deep time perspective on climate change such as presented in figure 1 in a discussion of 'dangerous anthropogenic interference'? Yes, because the magnitude of the anthropogenic perturbation of the carbon cycle forces us to go back far into the past, if we want to look for clues of what might happen in the future. Certainly, some of the climate changes reflected in figure 1 are a result of volcanism and continental drift, in particular the opening and closing of sea passages. However, recent data indicate that the carbon cycle was a major player in the transition from the Eocene hothouse to the modern-day icehouse world (e.g. Moran et al [11], Zachos et al [12]). The studies by Zachos et al [12] and Pearson and Palmer [13] found that carbon dioxide concentrations in the atmosphere decreased from well above 1000 ppm during the Eocene to below or around 300 ppm during the Mio-, Plio- and Pleistocene. On the basis of their data, it is likely that present-day levels of atmospheric carbon dioxide have not occurred for the last 23 million years. Moreover, projections of the growing anthropogenic perturbation of the carbon cycle in the 21st century, including scenarios that aim at stabilizing atmospheric CO2 concentration at twice its preindustrial value, carry us to carbon dioxide levels that were last seen during the Oligocene, where major restructuring of the climate system occurred.
But what about time scales? Certainly, climate policy cannot be concerned with climate changes that unravel over millions of years. However, the slowest processes in the climate system, i.e., heat penetration into the deep ocean and changes in ice sheet volume, operate on time scales of thousands of years, with deglaciation potentially occurring much faster within hundreds of years. Hence, if the driver is sufficiently fast, rapid climate change can occur. This is evidenced in the paleoclimatic record shown in figure 1 by the event called Paleocene–Eocene Thermal Maximum (PETM) 55 million years ago. During the PETM, global temperatures rose by 5–10 °C to presumably the hottest conditions during the Cenozoic era in a matter of several thousand years (Zachos et al [14]) due to a large perturbation of the carbon cycle (Zachos et al [15]) of hitherto unknown cause (Pagani et al [16]). A millennial time scale is still far beyond the time horizon of current socio-economic activity, but this is just the time scale for the system to equilibrate (bar the removal of carbon dioxide from the atmosphere–ocean-biosphere reservoir which proceeds much more slowly [15]). Significant changes will be felt much earlier. And when it comes to assessing 'dangerous anthropogenic interference with the climate system' that has the potential to change the face of the planet for a hundred thousand or more years to come, an extension of our time horizon to several hundred years seems to be appropriate.
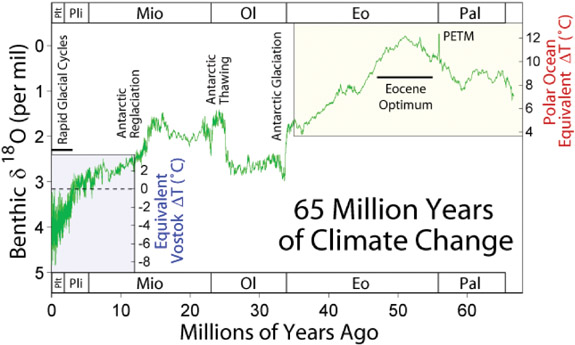
Figure 1. Any harm threshold here? Shown are δ18O stable isotope ratios (18O:16O relative to standard mean ocean water) in benthic foraminifera for the last 65 million years from Zachos et al [8]. The stable isotope ratio of the oxygen contained in the calcium carbonate of the foram shells depends on the water temperature in which they calcified (the warmer the water, the smaller δ18O). A complication arises from the fact that it also depends on the δ18O of the surrounding sea water, which is affected by latitude, evaporation and rainfall, and the presence or absence of large ice sheets. Therefore, these measurements can only be tied uniquely to past ocean temperatures for the early Cenozoic hothouse (Paleo- and Eocene) where no ice sheets existed, and for the most recent period by observing that the oxygen isotope measurements by Lisiecki and Raymo [9] are tightly correlated to temperature changes identified in the Vostok ice core (Petit et al [10]). Present day is indicated as 0. The large shifts of isotope ratio during the Oligocene also reflect changes in ice sheet volume. The figure was prepared by Robert A Rohde from published and publicly available data, and is distributed under the GNU free documentation license at www.globalwarmingart.com/wiki/Image:65_Myr_Climate_Change_Rev_png.
While the paleoclimatic record in figure 1 can inform us that 'dangerous interference with the climate system' may be in store for a species that evolved during icehouse conditions, it can not yet point us to specific harm thresholds in the climate system. Our knowledge about the climate in the past is still too sparse, and the analogy to present-day conditions too limited. In order to get a better idea about harm thresholds as the global mean temperature continues to increase, we need to turn to model projections of future climate change and associated impacts, as well as our own normative assessment of what might be labeled dangerous and what not. Given the imperfection of state-of-the-art model projections, e.g., in terms of extreme event statistics (although some have become available, see Tebaldi et al [17]), agreement on the regional scale (although improving, see [1], p 12 and figure SPM-6), and ability to model abrupt climate change, and the foreseeable disagreement between societal groups on what might be dangerous, this will certainly be an exercise in guestimating and consensus finding on some sort of uncertainty measure for the location of thresholds to dangerous climate change. In his article, Harvey offers his own take on the problem by presenting two different harm-threshold probability distributions: a stringent variant with median at 1.5 °C and 95% quantile at 2.7 °C of global mean temperature increase since preindustrial time, and a more lenient variant with median at 2.5 °C and 95% quantile at 3.8 °C. Whether or not one believes the temperature values attached to the list of impacts that Harvey offers in support of his harm assessment, there is still a value judgment about the 'dangerousness' of these impacts to be made. As Harvey points out himself, this is a question that can be informed, but not answered by science. Consequently, the allowable CO2 concentrations to prevent dangerous anthropogenic interference with the climate system presented in Harvey's article reflect a value judgment. So it is for you, the reader, and society at large to decide whether or not these findings are significant. If your judgment about the onset of dangerous climate change lies somewhere in the range of Harvey's harm-threshold probability distributions, his results will carry meaning for you. I, for my part, can certainly answer this question in the affirmative.
Once we accept this range of harm-threshold probabilities, the natural question emerges whether Harvey's result indicating that we are on the verge of dangerous anthropogenic interference with the climate system is inevitable. Is there an easy way out by adjusting the methodological framework that would present us considerably larger allowances of carbon in the atmosphere? It does not seem so. The virtue of Harvey's back-of-the-envelope calculation is that it includes the dominant factors in a simple, but fairly robust manner, which makes it hard to significantly alter the outcome by changing the details. Harvey has considered a wide range of medians and 95% quantiles for the probability distribution of climate sensitivity, but what if we changed the shape of both the climate sensitivity and harm-threshold probability density function (PDF)? After all, there is no particular reason why they should follow a lognormal distribution as Harvey assumed. The answer to this question is harbored by equation (1) of his article. The smaller the overlap between the climate sensitivity PDF and the harm-threshold CDF, the greater the carbon allowance available without committing dangerous interference with the climate system will be. And in this respect, Harvey's assumption of lognormality for both distributions goes some way in minimizing the overlap across possible shapes for fixed median and 95% quantiles.
So what about peaking concentrations that can reach higher carbon dioxide levels because the part of the equilibrium warming they would entail will never be realized due to the time lag of the temperature response? Harvey has included this—as he calls it—climate-disequilibrium credit in his analysis, and thus his dire assessment of the proximity of dangerous interference extends to the case of transient climate change. True, he has not considered uncertainty in the heat uptake of the ocean that dominates the time lag of the temperature response along with climate sensitivity. But assuming a larger heat uptake and a slower temperature response within the confidence bounds allowed by the 20th century temperature and ocean heat content records is unlikely to change the carbon allowance by more than a few tens of ppm. Thus, Harvey's findings seem to stand firm once we underwrite the value judgment that the probability of dangerous climate change lies somewhere in the range of the harm-threshold probability distributions put forward in his article. The new sense of urgency to address climate change mirrors this judgment.
References
[1] IPCC 2007 Climate Change 2007: The Physical Science Basis - Summary for Policymakers. Contribution of Working Group I to the Fourth Assessment Report of the Intergovernmental Panel on Climate Change (Cambridge: Cambridge University Press) in preparation (available for download at http://www.ipcc.ch)
[2] Harvey L D D 2007 Allowable CO2 concentrations under the United Nations Framework Convention on Climate Change as a function of the climate sensitivity probability distribution function Environ. Res. Lett.2 014001
[3] Dessai S, Adger W N, Hulme M, Turnpenny J, Köhler J and Warren R 2004 Defining and experiencing dangerous climate change Clim. Change64 11–25
[4] Oppenheimer M and Petsonk A 2005 Article 2 of the UNFCCC: historical origins, recent interpretations Clim. Change73 195–226
[5] Schellnhuber H J, Cramer W, Nakicenovic N, Wigley T and Yohe G (ed) 2006 Avoiding Dangerous Climate Change (Cambridge: Cambridge University Press) pp 392
[6] Keller K, Hall M, Kim S R, Bradford D F and Oppenheimer M 2005 Avoiding dangerous anthropogenic interference with the climate system Clim. Change73 227–38
[7] Gurian P L, Small M J, Lockwood J R and Schervish M J 2001 Benefit-cost estimation for alternative drinking water maximum contaminant levels Water Resources Res.37 2213–16
[8] Zachos J C, Pagani M, Sloan L, Thomas E and Billups K 2001 Trends, rhythms, and aberrations in global climate 65 Ma to present Science292 686–93
[9] Lisiecki L E and Raymo M E 2005 A Pliocene–Pleistocene stack of 57 globally distributed benthic δ18O records Paleoceanography20 PA1003
[10] Petit J R, Jouzel J, Raynaud D, Barkov N I, Barnola J M, Basile I, Bender M, Chappellaz J, Davis J, Delaygue G, Delmotte M, Kotlyakov V M, Legrand M, Lipenkov V, Lorius C, Pépin L, Ritz C, Saltzman E and Stievenard M 1999 Climate and Atmospheric History of the Past 420 000 years from the Vostok Ice Core, Antarctica Nature399 429–36
[11] Morgan K and the ACEX expedition team 2006 The Cenozic paleoenvironment of the Arctic Ocean Nature441 601–5
[12] Pagani M, Zachos J C, Freeman K H, Tipple B and Bohaty S 2005 Marked decline in atmospheric carbon dioxide concentrations during the paleogene Science309 600–3
[13] Pearson P N and Palmer M R 2000 Atmospheric carbon dioxide concentrations over the past 60 million years Nature406 695–9
[14] Zachos J C, Wara M W, Bohaty S, Delaney M L, Petrizzo M R, Brill A, Bralower, T J and Premoli-Silva I 2003 A transient rise in tropical sea surface temperature during the Paleocene–Eocene Thermal Maximum Science302 1551–4
[15] Zachos J C, Röhl U, Schellenberg S A, Sluijs A, Hodell D A, Kelly D C, Thomas E, Nicolo M, Raffi I, Lourens L J, McCarren H and Kroon D 2005 Rapid acidification of the ocean during the Paleocene–Eocene Thermal Maximum Science308 1611–5
[16] Pagani M, Caldeira K, Archer D, Zachos J C 2006 An ancient carbon mystery Science314 1556–7
[17] Tebaldi C, Hayhoe K, Arblaster J M and Meehl G A 2006 Going to the extremes: an intercomparison of model-simulated historical and future changes in extreme events Clim. Change79 185–211
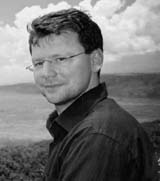
Elmar Kriegler received a diploma (MS equivalent) degree in physics from the Albert-Ludwigs-University of Freiburg, Germany, in 1998, and a PhD degree in physics from the University of Potsdam, Germany, in 2005. He worked for seven years as a graduate/post-doctoral researcher at the Potsdam Institute of Climate Impact Research on topics relating to the integrated assessment of climate change. He is now a visiting researcher at Carnegie Mellon University in Pittsburgh, USA, where he works—with support by a Marie-Curie Outgoing International Fellowship from the European Union—on the evaluation of climate policies under large uncertainty about climate change.