Abstract
Temperature increases due to climate change are expected to cause substantial reductions in global wheat yields. However, uncertainty remains regarding the potential role for irrigation as an adaptation strategy to offset heat impacts. Here we utilize over 7000 observations spanning eleven Kansas field-trial locations, 180 varieties, and 29 years to show that irrigation significantly reduces the negative impact of warming temperatures on winter wheat yields. Dryland wheat yields are estimated to decrease about eight percent for every one-degree Celsius increase in temperature, yet irrigation completely offsets this negative impact in our sample. As in previous studies, we find that important interactions exist between heat stress and precipitation for dryland production. Here, uniquely, we observe both dryland and irrigated trials side-by-side at the same locations and find that precipitation does not provide the same reduction in heat stress as irrigation. This is likely to be because the timing, intensity, and volume of water applications influence wheat yields, so the ability to irrigate—rather than relying on rainfall alone—has a stronger influence on heat stress. We find evidence of extensive differences of water-deficit stress impacts across varieties. This provides some evidence of the potential for adapting to hotter and drier climate conditions using optimal variety selection. Overall, our results highlight the critical role of water management for future global food security. Water scarcity not only reduces crop yields through water-deficit stress, but also amplifies the negative effects of warming temperatures.
Export citation and abstract BibTeX RIS

Original content from this work may be used under the terms of the Creative Commons Attribution 3.0 licence.
Any further distribution of this work must maintain attribution to the author(s) and the title of the work, journal citation and DOI.
Recent reports prepared for the Intergovernmental Panel on Climate Change predict a per-decade rise of 0.3 °C–0.6 °C in average global air temperature [1]. This is likely to be accompanied by increased heat wave frequency and decreased precipitation in certain geographical regions [2]. Temperature increases due to climate change are expected to cause substantial reductions in global wheat production [3].
One potential adaption path in response to climatic change could include targeting existing wheat varieties that exhibit improved heat-stress resilience, or developing new varieties with improved resilience through breeding efforts [4]. An alternative strategy would be to augment rainfed production with irrigation. Irrigation potentially reduces heat stress [5] by offsetting the additional evapotranspiration demand due to higher temperatures [6] and by cooling the canopy temperature [7]. These mechanisms imply that irrigation could reduce the impact of increased temperatures even if heat and water-deficit stresses have additive effects [2]. There remains much uncertainty about the magnitude of heat impacts on crop yields when irrigation is used as an adaptation strategy [7, 8]. This is an important knowledge gap, as the potential interaction of heat and water-deficit stress is a crucial consideration for accurately predicting the effects of climate change.
We follow previous work utilizing statistical regression models to estimate the impact of extreme heat—typically defined as ambient air temperatures above some threshold in the neighborhood of 30 °C—on observed crop yields [9–11], with the key innovation that we generalize the model to account for the combination of both dryland and irrigated production data for 180 varieties across 11 locations. We focus on wheat, which is the second most important crop globally in terms of caloric consumption [12]. A large source of uncertainty regarding wheat yields under climate change is the yield response to warming temperatures [13]. Statistical models that relate wheat yields to changes in temperature find negative impacts of warming [11, 14], and could enhance the accuracy of crop simulation models through inclusion of temperature and water stress relationships [15, 16].
The literature on the interactive effects of heat and water-deficit stress on crop yields is growing rapidly given the importance for understanding the likely impacts of climate change and developing optimal adaptation plans. Previous studies have quantified the interactive effect using crop simulation models [17], pairwise correlations between crop yields and climate extremes [18], or by estimating regression models linking crop yields to temperature variables under irrigated production systems [9, 19, 20]. There remains a need for additional research on heat stress impacts that incorporates the influence of irrigation and moisture stress on yields. Here, we extend previous literature by using field-level side-by-side comparisons of dryland and irrigated wheat yields. Importantly, we observe weather at each field location and thus do not have to rely on interpolated weather that could induce attenuation bias due to measurement error [21]. Experimental field plots in the same location also allow for a control, by measuring the effect of temperature and precipitation on dryland and irrigated yields, holding all other determinants of wheat yields constant. The unique nature of the data allows for accurate, unbiased estimates of the interaction of heat and water stress on crop yields.
Here, we produce the first estimate of the mitigating influence of irrigation on heat-stress using a large panel of yield data at the field scale where dryland and irrigated plots are grown side-by-side at the same location. Our data contain over 7000 observations spanning 11 Kansas field-trial locations, 180 varieties, and 29 years to show that irrigation significantly reduces the impact of warming temperatures on winter wheat yields. These data are from the Kansas Performance Tests with Winter Wheat Varieties, for which management practices vary by location and year. Production methods are considered 'best management practices' for each location-year and are designed to eliminate all yield-reducing features such as nutrient deficiencies or toxicities, damage from insect pests and disease, and competition from weeds. We estimate a pooled regression of irrigation coupled with dryland data, and interact a dummy variable for irrigation with a measure of extreme heat exposure, while controlling for the alternative effects of irrigation on the other weather variables in the model. It is important that we estimate the effect of irrigation rather than only precipitation—as in previous studies [11, 22]—because irrigation is a potential mechanism for adapting to climate change. The difference between irrigation and dryland wheat production is important because under irrigation the producer has control over when and how much water is applied, whereas under dryland production the producer has control over neither.
Data and methods
Weather data were taken from Kansas Weather Library. Daily ambient air temperature observations correspond to each field trial location [11]. A sinusoidal distribution was fit between daily minimum and maximum temperatures to estimate intraday exposures [9, 11]. These exposures were then summed for each month during the wheat growing season, September through May (harvest typically occurs during June). We also collect daily precipitation, which along with the temperature exposures, are summed to a cumulative measure for the Fall (September–November), Winter (December–February), and Spring (March–May) seasons. Wheat yield data are from Kansas Performance Tests with Winter Wheat Varieties for the years 1985 to 2013. Varieties in the same location-year were sown at the same time. The majority of observations correspond to dryland (non-irrigated, rainfed) winter wheat which are the same data as in [11]. We supplement these data with observations from irrigated field trials, which are only available for a subset of location-year combinations, i.e. not all locations conduct irrigated trials and irrigated trials are not conducted in every year for some locations. Irrigation in Kansas for wheat production is typically applied during the fall to aid germination and early crop development before dormancy. Irrigation may also be applied in the Spring, especially during the boot stage (May–June) when the crop reaches peak water use. All yield data are in bushels per acre.
The data vary temporally across growing seasons and cross-sectionally across field trial locations, seed varieties, and production type (i.e. dryland vs. irrigated). We account for time invariant factors that vary across locations, such as soil quality, with location fixed effects. We also include seed variety fixed effects as the mean yields vary across this dimension. These variety fixed effects directly control for changes in technology over time, as newer varieties are typically associated with higher yields. We also include variety-by-irrigation fixed effects, which in practice are incorporated by interacting dummy variables for the varieties with a dummy variable for irrigation. A quadratic time trend is included to measure changes in the experimental design of the field trials over time, which could result from changes in best management practices (e.g. more timely fertilizer applications).
The starting point for the regression model is the specification based on piecewise linear temperature effects as in [11]:
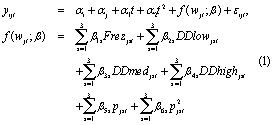
where yijt is log yield for variety i at location j in trial year t, αi and αj capture fixed effects across locations and varieties, α1t + α2t2 captures the trend component, and f (wjt; β) captures the (potentially nonlinear) effects of location-specific weather wjt on yields. The weather effects are modelled following the piecewise linear degree day approach [9], where Frezjst measures exposure in days to freezing temperatures, DDlowjst measures degree days between zero and the lower threshold, DDmedjst measures degree days between the lower and upper threshold, and DDhghjst measures degree days above the upper threshold. We use the same optimal knots for the piecewise linear components as [11], and multicollinearity of the degree day variables was not a major concern. The highest pairwise correlations among the fall, winter, and spring variables were 0.90, 0.93, and 0.70. We estimated a restricted version of our preferred model in which we used a single degree-days-above-zero variable in place of the low, medium, and high variables for the fall and winter. The highest pairwise correlation among all degree day variables under this new specification was 0.70, and the warming effects were similar to those reported here. The variable pjst measures cumulative precipitation. Note that weather variables are measured seasonally (s = 1,2,3 for Fall, Winter, and Spring).
We next consider several extensions of this model to account for pooling both dryland and irrigated observations. Measures of fit for these extensions are discussed in the results section. The final preferred model is given by
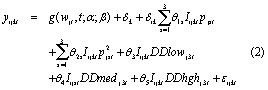
where yijkt is now log yield for variety i at location j under production type k (dryland vs. irrigated) in trial year t, and is the same specification as in equation (1) above. We extend the model by including the following: (i) irrigation fixed effects, (ii) variety-by-irrigation fixed effects, (iii) precipitation effects that differ by irrigation versus dryland, and (iv) Spring temperature effects that differ by irrigation versus dryland. The key to allowing for separate weather effects is through the dummy variable Iijkt, which takes on a value of 1 if variety i at location j in year t was grown under irrigated conditions, and zero otherwise. By interacting this dummy variable with the quadratic precipitation effects and the Spring temperature effects (ignoring freeze), we allow the effects on yields to differ across dryland versus irrigated production and test hypotheses of the form θ = 0 to evaluate the credibility of these extensions. We also present a series of robustness checks to evaluate whether further extensions of the model are warranted.
It is likely that the error terms εijkt are heteroskedastic and autocorrelated. We therefore cluster standard errors by year, which allows for errors to be heteroskedastic and spatially correlated within each year. This allows for a rich pattern of spatial correlation both within and across locations, varieties, and production type. This is an important consideration as standard errors in these types of observational studies are often much larger after accounting for spatial correlation.
Results
The raw data include both dryland and irrigated wheat yields for 180 varieties matched by location with daily minimum and maximum temperatures and precipitation tested on dryland and irrigated plots across 11 locations in Kansas from 1985 to 2013 (table S1 in the supplementary material available at stacks.iop.org/ERL/12/114027/mmedia, all tables and figures with a leading 'S' refer to the Supplementary Material). There are many more dryland observations (5713) than irrigated (1436), however there exists substantial yield and weather data variation within both trial types (figure 1, table S2, and figures S1–S3). An advantage of the data is the identical location for both dryland and irrigated plots, making direct comparisons that control for the same weather exposure possible. Throughout the analysis, the winter wheat growing period is divided into three-month seasons: Fall (September–November), Winter (December–February), and Spring (March–May).
Figure 1. Spatial and temporal variation of dryland and irrigated yields. We observe both irrigated and dryland yields across varieties at the location-year level, and construct boxplots for each year and trial type. The horizontal line across boxplots is the average yield. Each box is defined by the upper and lower quartile, with the median depicted as a horizontal line within the box. The endpoints for the whiskers are the upper and lower adjacent values, which are defined as the relevant quartile +/− three-halves of the interquartile range, and circles represent data points outside of the adjacent values. The bottom panel reports kernel density plots of both dryland and irrigated sample yields.
Download figure:
Standard image High-resolution imageWe pool dryland and irrigated data and estimate a regression model based on previous work that focused exclusively on dryland data [11], however we generalize the model to allow variety performance and weather effects to differ across dryland and irrigated production. Yields are expressed as a function of location and variety fixed effects, a quadratic trend specification is used to account for changes in management practices and/or experimental design over time, and a suite of weather variables across the Fall, Winter, and Spring seasons. Weather variables include quadratic functions of cumulative rainfall, total time exposure to temperatures below 0 °C, and a piecewise linear function based on degree days for the effect of positive temperatures. We utilize in- and out-of-sample prediction measures to guide our extension of this model to account for the differential effects of irrigation (table S3). Our preferred model includes an irrigation dummy variable, variety by irrigation fixed effects, separate precipitation effects for dryland and irrigated yields in each of the three seasons, and separate temperature effects in the Spring. These weather-irrigation interactions are supported by the data (table S4). The results are robust to several modifications of the model, discussed in more detail below.
Perhaps the most striking finding is that irrigation almost completely eliminates the negative effect of extreme heat (temperatures above 34 °C) in the Spring (figure 2), where the mitigating effect is statistically significant (P < 0.01, table S4). The confidence intervals for extreme heat impacts with irrigation are larger than for dryland, likely because we do not have information on the timing, intensity, or volume of irrigation at each location-year. It is worth noting that our inability to measure fine-scale multiple heat-stress occurrences—such as would materialize by high daytime temperatures followed by high nighttime temperatures instead of cooler nighttime temperatures that would aid plant recovery—likely contribute to this large standard error. This implies that we cannot rule out the possibility of scenarios under which irrigation fails to completely mitigate extreme heat exposure, even though it fully mitigates the effect in our sample on average. In addition, our focus is on ambient air temperatures while canopy and/or plant tissue temperatures are likely to be more informative for the physiological processes affected by heat stress [23]. Previous research has found differences in canopy temperatures under dryland versus irrigated conditions for a fixed level of ambient air temperature [24].
Figure 2. Nonlinear relation between positive Spring temperature and yields for both dryland and irrigated wheat. The graph displays changes in log yield if the crop is exposed for one day to a given 1 °C temperature interval. Dashed lines show 95% confidence intervals using standard errors clustered by year.
Download figure:
Standard image High-resolution imageAn advantage of these data is that they permit estimation of a discrete switch from dryland to irrigation, holding weather fixed. Under average weather conditions, we find that irrigation is associated with a 24 percent increase in yields (P < 0.001). This effect increases to 57 percent under water-deficit stress conditions (P < 0.001). We define water-deficit stress as Springtime precipitation at the 5th percentile of its historical distribution, and water-deficit stress impact as the difference between dryland and irrigated production when precipitation is held at this level. To estimate these impacts we focus on the 54 varieties that have appeared in at least 10 separate irrigated field trials, as care must be taken when estimating stress impacts for varieties with limited observations [4]. We re-estimate the model with this sub-sample and allow the water-deficit stress impact to vary across varieties.
The results in figure 3 show evidence of extensive genetic by environmental (G × E) interactions as the water-deficit stress impacts range from a 95% decrease in yield for the least resilient variety compared to a 33% decrease for the most resilient variety (figure S4). This result is consistent with previous observations that some varieties have more water-deficit stress tolerance than others [25].
Figure 3. Water-deficit stress impacts versus ability to resist extreme heat, average yields, and variety release year. Water-deficit stress impact is defined by the difference between dryland and irrigated production when precipitation is held fixed at the 5th percentile of its historical distribution. We estimate a separate effect for 54 of the 180 varieties in the data that have appeared in at least 10 different irrigated field trials. The first graph plots these impacts against extreme heat effects, the second against average yields under normal weather conditions, and the third against the year in which the variety was publicly released. The heat effect is estimated using only the dryland data, and the variety specific heat effects are estimated by interacting variety dummy variables with the Spring extreme heat variable (degree days over 34 °C). Average yields are predicted under this same dryland model but with yield replacing log-yield as the dependent variable and without the heterogeneous extreme heat effects. The pairwise correlations (p-value) for each of the three relationships are 0.19 (0.139), 0.65 (0.000), and 0.71 (0.000).
Download figure:
Standard image High-resolution imageNext, we examine potential genetic linkages between heat stress, water stress, and yield potential and find evidence of G × E impacts for heat stress as well. We plot water-deficit stress impacts for each variety against the heat stress impacts, yield performance under normal weather conditions, and the year in which the variety was publicly released (figure 3). Variety-specific heat stress impacts are estimated using a separate model using only the dryland data, and we allow the effect of extreme Spring temperatures (degree days over 34 °C) to vary across varieties. Overall, we find no evidence linking water and heat stress impacts, but strong evidence suggesting positive relationships between water-deficit stress resistance with both yield potential and the year the variety was released (figure 3).
We estimate a separate effect of precipitation for both dryland and irrigated yields, and find that irrigation provides protection against low rainfall outcomes, as predicted yields are substantially higher under irrigation (figure S5). This advantage becomes smaller as precipitation increases, however it remains positive over most of the precipitation distribution. Note that there are very few rainfall observations above 400 mm, so care must be taken in interpreting yield difference in the right-hand tail.
Our results suggest that irrigation's reduction of heat stress has the potential to offset warming impacts for wheat. To further investigate this, we first replicate previous results for dryland wheat [11] by predicting yield impacts across a variety of uniform warming scenarios for the entire growing season and find a similar pattern of negative impacts as expected (figure 4). Next, we use the same warming scenarios to predict impacts for irrigated wheat. We find that irrigation completely offsets the warming impacts as the estimates are small and not statistically significant (figure 4). We also interact cumulative precipitation with extreme heat in the Spring and allow the effect of this interaction to differ across dryland and irrigated production. We find that additional Spring precipitation can partially offset the negative effect of warming for dryland wheat, however it is not as effective as irrigation (figure 5).
Figure 4. Predicted warming impacts for dryland and irrigated wheat yields under alternative uniform temperature changes across the entire Fall-Winter-Spring growing season. Impacts are reported as the percentage change in yield relative to historical climate. Each two-bar cluster shows estimates for dryland and irrigation using parameter estimates from the preferred model. Bars show 95% confidence intervals using standard errors clustered by year.
Download figure:
Standard image High-resolution imageFigure 5. Influence of rainfall outcomes on predicted warming impacts for dryland and irrigated wheat. We extend the preferred model to include an interaction between precipitation and extreme heat in the Spring, and allow the effect of this interaction to differ across dryland and irrigation. The impacts are reported at the sample average of precipitation, as well as ± 20 and 50 percent of the sample average. Bars show 95% confidence intervals using standard errors clustered by year.
Download figure:
Standard image High-resolution imageThe robustness of the warming impacts is considered by estimating several modifications of the regression model and/or data, as reported in the supplementary material. We consider (i) location by irrigation fixed effects (figure S6), (ii) irrigation interactions with Fall and Winter temperatures (figure S7), (iii) restricting the data to locations or location-year combinations with both dryland and irrigated trials (figures S8–S9), (iv) restricting the data to only irrigated trials (figure S10), and (v) removing potential outlier observations (figures S11–S13). Our key result that irrigation reduces heat stress remains robust across all specifications.
Discussion
Water-deficit and heat stress are considered to be the two most important environmental factors that influence crop production [8]. They can affect a large number of crop development processes, including physiological, biochemical, and molecular changes at both cellular and whole-plant levels that affect both yields and quality [22]. Until recently the mechanisms driving these outcomes had not been well understood [26]. Recent studies have identified important relationships between heat stress and the reproductive functioning of the plant, as well as the role of other climatic conditions such as vapor pressure deficit [6, 27, 28]. While both forms of stress have been studied extensively, they are usually studied separately as they affect plant metabolism in different ways [2, 8]. Water-deficit stress has been linked to reduced conductance and gas exchange while heat stress is associated with the biochemistry of photosynthesis and membrane function. The interaction of these stresses remains an open area of study as the molecular and metabolic responses of plants to a combination of water-deficit and heat stress are often considered unique and it can be difficult to separately identify their effects from observed plant responses as they often occur simultaneously [22].
Our estimate of a 6% wheat yield reduction for dryland production under a 1 °C warming scenario is consistent with both US and global aggregate impacts [29]. Our results suggest that irrigation may have the potential to eliminate heat stress in climate conditions and mega environments [30] similar to the central US. Approximately 20 percent of global wheat growing area is irrigated (FAO) and irrigation occurs predominately in developing nations such as India, China, Pakistan, Egypt, and Mexico [31]. Irrigated wheat production in these areas may be subject to hotter temperatures than those observed here, and irrigation may not completely offset heat stress at these higher levels. In addition, local humidity levels could also influence irrigation's ability to reduce heat stress in other wheat production areas.
Reduced irrigation from water scarcity is likely to have substantial impacts on global wheat production because of the effects of water-deficit stress, but also because of its implications for heat stress at a time when heat stress is expected to increase due to climate change. Our results suggest that water is likely to become more valuable as climate change occurs. Therefore, the use of irrigation as an adaptation mechanism has important implications for future water use, and further research is needed to better understand the role of irrigation as a potential adaptation mechanism and the implied management implications for scarce natural resources. Importantly, irrigation may not be feasible in every crop production region, and it is important to consider competing uses and the overall availability of water. We also note that widespread shifts in irrigated acreage can affect local climate, often by reducing observed temperatures [32, 33], which can be viewed as a complementary indirect effect relative to the one identified here.
Our results also demonstrate extensive varietal heterogeneity in yield responses to water-deficit and heat stress (i.e. G × E interactions), and that these responses are uncorrelated across varieties on average. This is consistent with previous research that shows that cultivars within crop species have different responses to heat and water-deficit stress [34]. However, we do observe a few varieties that exhibit high resistance to both, which could aid plant scientists identifying genetic markers associated with these phenotypic traits. However, it should be noted that we estimated heterogeneous heat stress impacts while assuming homogenous water-deficit stress impacts, and vice versa. There exists a need for more data to study these interactions in which tests are conducted with these stresses occurring both separately and together [8], or through the use of large scale statistical models that incorporate soil moisture data alongside temperature data [35]. We find strong evidence of a positive association between water-deficit stress resistance and both average yields and genetic innovations over time, suggesting that breeding programs have made significant progress in developing lines that stack both water-deficit stress resistance and yield potential.
In summary, our main findings are as follows. Dryland wheat yields are estimated to decrease about eight percent for every one-degree Celsius increase in temperature, yet irrigation completely offsets this negative impact. Our data also permit identification of water-stress impacts by variety, and we find evidence of extensive differences across varieties. As in previous studies, we find important interactions between heat stress and precipitation for dryland production. However, we find that precipitation does not provide the same reduction in heat stress as irrigation. Our results highlight the critical role of water management for future global food security as we find that water scarcity not only reduces crop yields through water-deficit stress, but also amplifies the negative effects of heat stress. A caveat to these results is that future increases in CO2 concentrations might limit plant transpiration, thereby reducing the plant's ability to cool itself and the efficacy of irrigation in reducing heat stress.
Acknowledgments
We are grateful to Krishna Jagadish, and Jane Lingenfelser for assistance with the field-trial production data. Contribution 18-151-J from the Kansas Agricultural Experiment Station.