ABSTRACT
As an application of our recent observational error model, we present the astrometric masses of 26 main-belt asteroids. We also present an integrated ephemeris of 300 large asteroids, which was used in the mass determination algorithm to model significant perturbations from the rest of the main belt. After combining our mass estimates with those of other authors, we study the bulk porosities of over 50 main-belt asteroids and observe that asteroids as large as 300 km in diameter may be loose aggregates. This finding may place specific constraints on models of main-belt collisional evolution. Additionally, we observe that C-group asteroids tend to have significantly higher macroporosity than S-group asteroids.
Export citation and abstract BibTeX RIS
1. INTRODUCTION
The technique of astrometric mass determination, in which the deflection of a small body's trajectory allows us to deduce the mass of a larger perturbing body, may be entering a particularly fruitful period, as near-Earth asteroid (NEA) surveys coincidentally produce a flood of high-precision observations of main-belt asteroids.
The mass of an asteroid, when combined with its volume and mineralogy, allows us to derive its bulk density and porosity. Indeed, the direct determination of asteroid physical properties has acquired a heightened sense of urgency in recent years, as it has become clear that any attempt to deflect a threatening NEA must be informed by knowledge of the body's density, composition, and structure. So few asteroid masses are currently known that their gravitational perturbations remain the single greatest source of error in planetary ephemerides (Standish 2000) and while indirect methods of mass calculation, such as assuming a given density based on taxonomic class, have proven extremely useful in dynamical modeling, such assumptions must be calibrated against direct observation.
New surveys, such as PanSTARRS, promise to routinely provide observations with uncertainties on the order of 0.1 arcsec (Jedicke & The Pan-Starrs Collaboration 2004), thus permitting highly sensitive mass estimates, but many mass determination encounters are fixed in the past or rely upon long observational baselines. Thus, while new and precise observations may be helpful, we must also make the best possible use of contemporaneous and historical observations.
Unfortunately, the current convention in least-squares orbit (and mass) determination is to assume that all observations from a given era are of equivalent precision, regardless of source, and that any errors in these observations are uncorrelated. As we will see, these assumptions make it difficult to simultaneously utilize both heritage observations and more precise sightings from the new surveys; and while such simplifications may have been necessary when computational resources were more limited, they actually penalize the accuracy and precision of current work.
In this paper, we will utilize a new statistical error model of asteroid observations, providing realistic, site-specific estimates of observational uncertainties and error correlations in given eras. This error model will help us deduce the masses of 26 main-belt asteroids and create an integrated ephemeris of 300 of the largest asteroids. We will then discuss what conclusions can be drawn from the resulting densities and porosities.
2. CALCULATIONS—OVERVIEW
Astrometric mass determination is a modification of conventional least-squares orbit determination, in which the mass of the perturbing body is added as a seventh solve-for parameter. Ideally, the process is applied to relatively close encounters between a large subject asteroid perturbing a small test asteroid, where precise observations of the test asteroid exist before, during, and after the encounter.
Since the mass determination calculations are lengthy, it is first necessary to conduct a survey for encounters likely to result in significant deflection of the test asteroid. Furthermore, since the mass determination process is based upon perturbations in the trajectory of a test asteroid, it is absolutely essential to employ an accurate force model that accounts for all other known perturbations upon that test asteroid. Since newly calculated asteroid masses and orbits improve the accuracy of the force model, the processes of mass determination and force model refinement are intertwined.
We refer to our previous paper (Baer & Chesley 2008) for a detailed explanation of the selection of candidate encounters, and the iterative calculation of asteroid masses and ephemerides. What follows is a brief summary.
2.1. The Mass Determination Algorithm
The conventional least-squares orbit determination algorithm relies upon finding the minimum of the cost function:
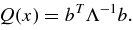
In this equation, x is the state vector, b = b(x) is a vector containing the "observed − computed" residuals for each observation, and Λ is the observational covariance matrix, with
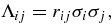
where σi is the square root of the covariance (i.e., the uncertainty) of the ith observation and rij is the correlation between the ith and jth observations. We would find the minimum by seeking stationary points of Q(x):
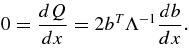
If we define , then the equation to be solved reduces to
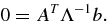
If we assume a total of N data points, where N = 2 (number of optical observations) + (number of radar delay observations) + (number of radar Doppler observations), then b is an N × 1 vector and A is an N × 6 matrix.
The solution of this equation is

Conventionally, it is assumed that σi is 3 arcsec for optical observations prior to 1890, 2 arcsec for observations from 1890 to 1950, and 1 arcsec thereafter. The additional assumption that observations are uncorrelated, i.e.,
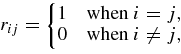
leads to Λ being a diagonal matrix, thus simplifying the solution. The validity and consequences of these assumptions will be addressed at length in Section 2.2.
In modifying this algorithm for astrometric mass determination, the mass of the perturbing subject body is added as a seventh solve-for parameter in the state vector x; otherwise, the principles are identical.
As implemented here, the least-squares algorithm begins with the best available estimates of the test asteroid's epoch state vector and the subject asteroid's mass; the algorithm iteratively refines these parameters, with all observations of the test asteroid included. Once convergence has been achieved, observations differing from the best-fit predictions by greater than a specified threshold are rejected in the next iteration (although they can be reconsidered in subsequent iterations); and the process continues until convergence has again been achieved.
2.2. The Observational Error Model
In our previous paper (Baer & Chesley 2008), we presented astrometric masses for 21 main-belt asteroids; we also created the BC405 integrated asteroid ephemeris, which served to model the perturbations from other large asteroids in the orbit and mass determination process. While these previous results were significant, they were nonetheless subject to two flawed assumptions made in the least-squares algorithm.
- 1.We assume that all observatories in a given era have the same observational uncertainty.
- 2.We assume that the errors in observations are uncorrelated.
The first assumption is clearly unrealistic. Factors such as aperture size, detector technology, astrometric reduction methodology, and observer experience are clearly relevant in determining the level of observational precision and accuracy.
Combining the astrometric products of diverse instruments also becomes problematic. For instance, Pan-STARRS is expected to yield observational uncertainties of 0.1 arcsec (Jedicke & The Pan-Starrs Collaboration 2004), almost an order of magnitude more precise than current surveys; but if all observations are weighted with a uniform uncertainty of 1 arcsec, the least-squares algorithm will produce a solution whose errors are equally distributed. It is therefore highly likely that the apparent residuals in Pan-STARRS observations will greatly exceed the design specifications, casting doubt on the system's performance, and nullifying the usefulness of these highly precise observations.
Moreover, Carpino et al. (2003) have demonstrated that closely spaced observations of the same asteroid made by the same observatory are significantly correlated; ignoring this correlation has the effect of inaccurately weighting such observations in the least-squares covariance matrix.
In short, subject to these assumptions, the least-squares algorithm will converge to a suboptimal orbit and mass.
Addressing these deficiencies required the development of time-based error models for all MPC-contributing observatories, including realistic values for the biases and rms errors of each observatory, and a model for the correlation of observational errors.
Carpino et al. (2003) outlined a design for such an observational error model. In Baer et al. (2011), we built upon this work, extending it to all available optical observations of the numbered asteroids and validating its performance against conventional methods.
The error model is essentially a look-up table, intended to provide a statistical summary of each MPC-contributing observatory's performance, and accounting for any factors that might reasonably be expected to impact that performance. The table is divided into "bins" covering a minimum period of 30 days, differentiated from one another by observatory, date, apparent magnitude, detector technology, and the location of an observation relative to the galactic plane. Knowing these characteristics, the corresponding bin lists the mean R.A. and decl. rms errors and biases for that observatory and provides the parameters for a time-based exponential model of the error correlation between two observations, each for use in the least-squares algorithm.
In Baer et al. (2011), we demonstrated that this error model yields orbits that describe the trajectory of an asteroid both more accurately and more precisely than conventional practice. In this paper, we apply this observational error model to derive the most accurate possible asteroid masses.
3. MASS DETERMINATIONS
As noted above, isolating the weak signatures of asteroid perturbations requires an accurate force model. Planetary perturbations were modeled using the masses and orbits from the JPL DE405 ephemeris. Perturbations from the 300 most significant asteroids (the same asteroids used in the development of the DE405 ephemeris) were modeled using our BC405 asteroid ephemeris.
We began by using the observational error model to recalculate both the orbits of the 300 asteroids in the BC405 ephemeris and the masses of as many asteroids from our earlier survey as possible. The newly revised asteroid orbits and masses were used to recreate the ephemeris, which was then used to refine the masses; and this iterative process was repeated a total of 11 times, until each ephemeris state vector component and subject asteroid mass converged to within 1σ.
While the BC405 ephemeris was developed solely to assist the mass determinations, we nonetheless believe it could prove valuable in many high-precision dynamical applications requiring an accurate force model for the minor planets. The state vector components of the 300 asteroids at the central epoch of the ephemeris (Julian Date 2,453,775.0), and their calculated or assumed masses, are available to other researchers via FTP.4
In calculating the mass (and the uncertainty in that mass) of a specific asteroid, we conducted successive runs using least-squares rejection thresholds of 5σ, 3σ, and 1.5σ; the final reported mass and uncertainty were obtained as weighted averages of these three sets of values. Measured masses were considered valid if they yielded a density of between 0.5 and 8.0 g cm−3, and if the significance (defined as the ratio of the measured mass to its uncertainty) exceeded two.
In previous papers, we voiced concern about the reliability of the mass uncertainty produced by the least-squares algorithm; by definition, it would understate the effects of unmodeled perturbations, including uncertainties in the masses of key planetary and asteroidal perturbers. To assess the validity of these uncertainties, we conducted a variational analysis, repeating the 5σ mass determination runs with slightly different values for the masses of seven key perturbing bodies: Jupiter, Saturn, 1 Ceres, 2 Pallas, 3 Juno, 4 Vesta, and 10 Hygeia. In each case, the perturber's mass was increased by the magnitude of its published uncertainty; and the resulting mass of the subject asteroid was compared to the nominal value to determine if the least-squares uncertainty was realistic, and if the mass itself was reliable.
For instance, the nominal mass uncertainty resulting from the 4/17 encounter was 3.6 × 10−13 M☉. However, as Table 1 demonstrates, slight changes in the masses of significant perturbers caused changes in the mass of 4 Vesta on the order of 3.0 × 10−12 M☉; therefore, this value was adopted as the uncertainty. In the cases of 10/357 and 52/306, we obtained seemingly valid masses of 6.6 ± 1.7 × 10−11 M☉ and 2.50 ± 0.22 × 10−11 M☉, respectively. In each case, however, the variational analysis yielded such a wide range of values that we elected to discard these results as unreliable.
Table 1. Variant Mass Determinations Obtained by 1σ Variations in Masses of Largest Perturbers or by Initializing the Least-squares Algorithm at One-half of the Expected Mass
Subject | Test | Jupiter | Saturn | 1 Ceres | 2 Pallas | 3 Juno | 4 Vesta | 10 Hygeia | ![]() |
---|---|---|---|---|---|---|---|---|---|
Asteroid | Asteroid | Variant | Variant | Variant | Variant | Variant | Variant | Variant | Variant |
4 | 17 | 137.9 | 133.8 | 132.7 | 134.0 | 133.9 | 134.3 | 133.6 | |
5 | 17 | 1.12 | 1.26 | 1.25 | 3.02 | 1.50 | 1.20 | 0.0384 | |
10 | 357 | 90.2 | 70.3 | 105.7 | 93.7 | 97.2 | 94.9 | 67.2 | |
11 | 17 | 2.67 | 2.50 | 2.01 | 2.53 | 3.03 | 2.54 | 2.78 | 1.56 |
31 | 3176 | 28.9 | 29.4 | 29.7 | 26.7 | 29.0 | 25.4 | 29.1 | 27.9 |
52 | 306 | 26.9 | 26.7 | 23.7 | 18.8 | 43.5 | 12.5 | 15.6 | 28.7 |
Note. All masses are in units of 10−12 M☉.
Download table as: ASCIITypeset image
By contrast, variational analysis demonstrated that the mass resulting from the 31/3176 encounter is relatively consistent within the range of least-squares uncertainty, regardless of changes to perturbing masses. As will be seen in Section 4, this mass leads to a bulk density that is inconsistent with its taxonomically derived grain density. Nevertheless, we have no objective dynamical reason to discard it; so we have elected to report this result, although it must obviously be viewed with caution.
In several instances, we wanted to ensure that the mass produced by the least-squares algorithm was not dependent upon the initial assumed value. Therefore, we conducted additional mass determination runs, in which the initial assumed mass was one-half of the estimated value.
In the case of the 5/17 encounter, we obtained a nominal mass for 5 Astraea of 1.09 ± 0.13 × 10−12 M☉. However, this mass is quite sensitive to variations in the mass of 3 Juno; and when the initial assumed mass was reduced by half, the least-squares algorithm produced a far lower value. Therefore, we do not believe this mass can be considered reliable.
The 11/17 encounter had previously been analyzed by Viateau & Rapaport (1997b) and Viateau & Rapaport (2001), and we obtained a nominal mass for 11 Parthenope of 2.72 ± 0.30 × 10−12 M☉, which is consistent with their results. However, variational analysis demonstrated that our derived mass is sensitive both to variations in the masses of 1 Ceres and 3 Juno and to the value of the initial assumed mass. So while this mass is physically reasonable, we feel the observed variations cast doubt upon its reliability; and we have elected not to report it.
Several of the obtained asteroid masses benefited from the availability of additional observations, beyond those in the MPC database. The SkyMorph Web site5 provides access to images from multiple sources, including the Digitized Sky Survey (Pravdo et al. 1998). We theorized that images from the SkyMorph archive might allow us to precover some test asteroids and establish their pre-encounter trajectories. In several cases, this helped to refine existing masses; in other cases (including 6 Hebe and 8 Flora), it allowed us to calculate new masses in cases where pre-encounter observations had previously not existed. These additional observations are available from the lead author's Web site.6
The resulting masses for 26 main-belt asteroids are listed in Table 2.
Table 2. Masses Measured Using the Observational Error Model
Subject | Test | Mass | Std Dev | Significance |
---|---|---|---|---|
Asteroid | Asteroid | (M☉) | (M☉) | |
1 | 91 | 4.796 × 10−10 | 2.7 × 10−12 | 175.1 |
1 | 348 | 4.719 × 10−10 | 1.2 × 10−12 | 392.7 |
1 | 5303 | 4.768 × 10−10 | 1.1 × 10−12 | 426.6 |
1 | 13801 | 4.796 × 10−10 | 1.8 × 10−12 | 264.9 |
1 | Average | 4.757 × 10−10 | 7.2 × 10−13 | 661.0 |
2 | 582 | 1.056 × 10−10 | 7.9 × 10−12 | 13.4 |
2 | 4971 | 9.10 × 10−11 | 1.2 × 10−11 | 7.8 |
2 | Average | 1.010 × 10−10 | 6.5 × 10−12 | 15.5 |
3 | 251 | 2.21 × 10−11 | 9.5 × 10−12 | 2.3 |
3 | 17715 | 1.77 × 10−11 | 7.4 × 10−12 | 2.4 |
3 | 61235 | 1.35 × 10−11 | 2.2 × 10−12 | 6.0 |
3 | Average | 1.44 × 10−11 | 2.3 × 10−12 | 6.3 |
4 | 17 | 1.335 × 10−10 | 1.5 × 10−12 | 89.0 |
4 | 67 | 1.329 × 10−10 | 1.9 × 10−12 | 68.9 |
4 | 77 | 1.333 × 10−10 | 3.8 × 10−12 | 34.8 |
4 | 197 | 1.273 × 10−10 | 1.0 × 10−12 | 121.9 |
4 | 5205 | 1.307 × 10−10 | 1.1 × 10−12 | 119.1 |
4 | 6027 | 1.370 × 10−10 | 5.6 × 10−12 | 24.3 |
4 | 8331 | 1.295 × 10−10 | 9.7 × 10−13 | 134.0 |
4 | Average | 1.300 × 10−10 | 5.3 × 10−13 | 243.9 |
6 | 1150 | 6.81 × 10−12 | 1.16 × 10−12 | 5.9 |
6 | 4497 | 4.19 × 10−12 | 9.82 × 10−13 | 4.3 |
6 | 5295 | 1.06 × 10−11 | 1.45 × 10−12 | 7.3 |
6 | Average | 6.40 × 10−12 | 6.67 × 10−13 | 9.6 |
7 | 1188 | 9.45 × 10−12 | 9.8 × 10−13 | 9.7 |
7 | 12301 | 1.70 × 10−11 | 5.0 × 10−12 | 3.4 |
7 | 1879 | 8.57 × 10−12 | 2.2 × 10−12 | 3.9 |
7 | 47544 | 1.24 × 10−11 | 3.6 × 10−12 | 3.5 |
7 | 52443 | 9.31 × 10−12 | 8.7 × 10−13 | 10.7 |
7 | 17186 | 5.34 × 10−12 | 7.5 × 10−13 | 7.2 |
7 | 2346 | 1.48 × 10−11 | 4.5 × 10−12 | 3.3 |
7 | 1651 | 8.85 × 10−12 | 3.3 × 10−12 | 2.7 |
7 | 3133 | 1.13 × 10−11 | 3.0 × 10−12 | 3.8 |
7 | 46529 | 1.58 × 10−11 | 3.9 × 10−12 | 4.0 |
7 | Average | 8.12 × 10−12 | 4.6 × 10−13 | 17.8 |
8 | 330 | 3.31 × 10−12 | 4.3 × 10−13 | 7.6 |
8 | 1577 | 3.64 × 10−12 | 1.6 × 10−12 | 2.3 |
8 | Average | 3.33 × 10−12 | 4.2 × 10−13 | 8.0 |
9 | 20 | 7.0 × 10−12 | 3.1 × 10−12 | 2.3 |
9 | 43 | 4.0 × 10−12 | 1.6 × 10−12 | 2.5 |
9 | 29818 | 7.3 × 10−12 | 1.8 × 10−12 | 4.1 |
9 | Average | 5.7 × 10−12 | 1.1 × 10−12 | 5.1 |
10 | 69 | 4.993 × 10−11 | 1.3 × 10−11 | 3.8 |
10 | 111 | 5.590 × 10−11 | 3.9 × 10−12 | 14.4 |
10 | 209 | 5.626 × 10−11 | 5.2 × 10−12 | 10.8 |
10 | 1259 | 7.644 × 10−11 | 1.0 × 10−11 | 7.6 |
10 | 3946 | 4.051 × 10−11 | 1.0 × 10−12 | 40.1 |
10 | 6006 | 4.776 × 10−11 | 9.8 × 10−12 | 4.9 |
10 | 6143 | 5.283 × 10−11 | 2.8 × 10−12 | 19.0 |
10 | 11215 | 4.131 × 10−11 | 1.7 × 10−12 | 24.3 |
10 | 13266 | 6.130 × 10−11 | 5.3 × 10−12 | 11.6 |
10 | 15487 | 5.806 × 10−11 | 1.3 × 10−11 | 4.6 |
10 | 24433 | 4.113 × 10−11 | 2.9 × 10−12 | 14.0 |
10 | 48499 | 4.425 × 10−11 | 4.4 × 10−12 | 10.0 |
10 | 54270 | 6.074 × 10−11 | 1.4 × 10−11 | 4.3 |
10 | 57493 | 6.943 × 10−11 | 1.1 × 10−11 | 6.4 |
10 | 75794 | 6.042 × 10−11 | 1.1 × 10−11 | 5.4 |
10 | Average | 4.358 × 10−11 | 7.4 × 10−13 | 58.7 |
13 | 14689 | 8.0 × 10−12 | 2.2 × 10−12 | 3.7 |
14 | 15186 | 2.34 × 10−12 | 1.0 × 10−12 | 2.3 |
14 | 64537 | 5.75 × 10−12 | 1.4 × 10−12 | 4.1 |
14 | Average | 3.49 × 10−12 | 8.2 × 10−13 | 4.2 |
15 | 14401 | 1.427 × 10−11 | 1.4 × 10−12 | 10.0 |
15 | 16693 | 1.554 × 10−11 | 2.2 × 10−12 | 7.1 |
15 | 45690 | 1.588 × 10−11 | 5.4 × 10−12 | 2.9 |
15 | 1284 | 1.639 × 10−11 | 1.1 × 10−12 | 15.3 |
15 | 50278 | 1.599 × 10−11 | 1.5 × 10−13 | 106.3 |
15 | 1313 | 1.303 × 10−11 | 2.9 × 10−12 | 4.5 |
15 | Average | 1.597 × 10−11 | 1.5 × 10−13 | 108.2 |
16 | 13206 | 1.167 × 10−11 | 4.5 × 10−13 | 25.8 |
16 | 94 | 9.83 × 10−12 | 1.7 × 10−12 | 5.8 |
16 | 39054 | 9.60 × 10−12 | 1.5 × 10−12 | 6.4 |
16 | Average | 1.140 × 10−11 | 4.2 × 10−13 | 27.2 |
17 | 11 | 7.21 × 10−13 | 2.5 × 10−14 | 29.0 |
19 | 3486 | 3.90 × 10−12 | 3.7 × 10−13 | 10.5 |
19 | 27799 | 9.10 × 10−12 | 1.6 × 10−12 | 5.8 |
19 | Average | 4.18 × 10−12 | 3.6 × 10−13 | 11.5 |
20 | 44 | 1.68 × 10−12 | 3.5 × 10−13 | 4.8 |
21 | 29523 | 1.31 × 10−12 | 4.4 × 10−13 | 2.9 |
29 | 987 | 7.73 × 10−12 | 3.2 × 10−13 | 24.1 |
29 | 48464 | 6.25 × 10−12 | 1.2 × 10−12 | 5.2 |
29 | Average | 7.63 × 10−12 | 3.1 × 10−13 | 24.6 |
31 | 3176 | 2.92 × 10−11 | 9.9 × 10−12 | 3.0 |
39 | 2416 | 2.83 × 10−12 | 7.3 × 10−13 | 3.9 |
52 | 124 | 1.139 × 10−11 | 7.9 × 10−13 | 14.5 |
65 | 526 | 5.30 × 10−12 | 9.6 × 10−13 | 5.5 |
88 | 7 | 9.40 × 10−12 | 6.0 × 10−13 | 15.6 |
88 | 7629 | 8.12 × 10−12 | 1.4 × 10−12 | 6.0 |
88 | Average | 9.19 × 10−12 | 5.5 × 10−13 | 16.7 |
511 | 203 | 1.58 × 10−11 | 2.6 × 10−12 | 6.1 |
511 | 532 | 1.75 × 10−11 | 1.3 × 10−12 | 13.9 |
511 | 1550 | 2.46 × 10−11 | 2.0 × 10−12 | 12.2 |
511 | Average | 1.896 × 10−11 | 9.9 × 10−13 | 19.2 |
704 | 10034 | 3.14 × 10−11 | 3.1 × 10−12 | 10.1 |
704 | 37 | 1.89 × 10−11 | 4.6 × 10−12 | 4.1 |
704 | 7461 | 1.97 × 10−11 | 5.9 × 10−12 | 3.4 |
704 | 1467 | 1.77 × 10−11 | 1.1 × 10−12 | 16.8 |
704 | 95 | 2.15 × 10−11 | 2.3 × 10−12 | 9.3 |
704 | Average | 1.950 × 10−11 | 8.9 × 10−13 | 21.9 |
804 | 1002 | 1.75 × 10−12 | 4.0 × 10−13 | 4.4 |
Download table as: ASCIITypeset image
4. INTERPRETATION OF MASSES
To place our results into context, in Table 3, we have listed our mass determinations alongside those of other authors, including astrometric, binary, and spacecraft-derived masses. The best available three-axis ellipsoid model of each asteroid is also listed, so the bulk density can be calculated.
Table 3. Recent Astrometric, Binary, and Spacecraft Asteroid Mass Determinations
Asteroid | Mass | Bulk Density | Assumed | Uncertainty in | Dimensions | Δ | |||||||
---|---|---|---|---|---|---|---|---|---|---|---|---|---|
Mass | Uncertainty | Bulk Density | Uncertainty | Grain Density | Bulk | Bulk | Taxonomic | (km) | Radius | Mass | |||
(in M☉) | (in M☉) | (g cm−3) | (g cm−3) | (g cm−3) | Porosity | Porosity | Class1 | a | b | c | (km) | Reference | |
1 Ceres | 4.700 × 10−10 | 3.0 × 10−11 | 2.07 | 0.14 | 2.12 | 0.02 | 0.00 | G | 9752 | 9752 | 9092 | 3.62 | Goffin (1991) |
4.796 × 10−10 | 8.5 × 10−12 | 2.11 | 0.06 | 2.12 | 0.00 | 0.00 | G | 9752 | 9752 | 9092 | 3.62 | Sitarski & Todorovic-Juchniewicz (1992) | |
4.800 × 10−10 | 2.2 × 10−11 | 2.11 | 0.11 | 2.12 | 0.00 | 0.00 | G | 9752 | 9752 | 9092 | 3.62 | Williams (1992) | |
4.622 × 10−10 | 7.1 × 10−12 | 2.03 | 0.06 | 2.12 | 0.04 | 0.00 | G | 9752 | 9752 | 9092 | 3.62 | Sitarski & Todororovic-Juchniewicz (1995) | |
5.000 × 10−10 | 2.0 × 10−11 | 2.20 | 0.10 | 2.12 | −0.04 | 0.00 | G | 9752 | 9752 | 9092 | 3.62 | Viateau & Rapaport (1995) | |
4.670 × 10−10 | 9.0 × 10−12 | 2.05 | 0.06 | 2.12 | 0.03 | 0.00 | G | 9752 | 9752 | 9092 | 3.62 | Carpino & Knezevic (1996) | |
4.160 × 10−10 | 1.9 × 10−11 | 1.83 | 0.09 | 2.12 | 0.14 | 0.01 | G | 9752 | 9752 | 9092 | 3.62 | Kuzmanoski (1996) | |
4.785 × 10−10 | 3.9 × 10−12 | 2.10 | 0.05 | 2.12 | 0.01 | 0.00 | G | 9752 | 9752 | 9092 | 3.62 | Viateau & Rapaport (1997a) | |
4.759 × 10−10 | 2.3 × 10−12 | 2.09 | 0.05 | 2.12 | 0.01 | 0.00 | G | 9752 | 9752 | 9092 | 3.62 | Viateau & Rapaport (1998) | |
4.390 × 10−10 | 4.0 × 10−12 | 1.93 | 0.05 | 2.12 | 0.09 | 0.00 | G | 9752 | 9752 | 9092 | 3.62 | Hilton (1999) | |
4.700 × 10−10 | 4.0 × 10−12 | 2.07 | 0.05 | 2.12 | 0.02 | 0.00 | G | 9752 | 9752 | 9092 | 3.62 | Michalak (2000) | |
4.736 × 10−10 | 2.6 × 10−12 | 2.08 | 0.05 | 2.12 | 0.02 | 0.00 | G | 9752 | 9752 | 9092 | 3.62 | Kovačević & Kuzmanoski (2007) | |
4.755 × 10−10 | 8.0 × 10−13 | 2.09 | 0.05 | 2.12 | 0.01 | 0.00 | G | 9752 | 9752 | 9092 | 3.62 | Baer et al. (2008) | |
4.757 × 10−10 | 7.2 × 10−13 | 2.09 | 0.05 | 2.12 | 0.01 | 0.00 | G | 9752 | 9752 | 9092 | 3.62 | This paper | |
2 Pallas | 1.590 × 10−10 | 5.00 × 10−12 | 4.04 | 0.19 | 2.90 | −0.39 | −0.02 | B | 5703 | 5253 | 5003 | 33 | Hilton (1999) |
1.21 × 10−10 | 2.6 × 10−11 | 3.07 | 0.67 | 2.90 | −0.06 | −0.01 | B | 5703 | 5253 | 5003 | 33 | Michalak (2000) | |
1.17 × 10−10 | 3.0 × 10−12 | 2.97 | 0.13 | 2.90 | −0.02 | 0.00 | B | 5703 | 5253 | 5003 | 33 | Goffin (2001) | |
1.06 × 10−10 | 4.0 × 10−12 | 2.69 | 0.14 | 2.90 | 0.07 | 0.00 | B | 5703 | 5253 | 5003 | 33 | Baer et al. (2008) | |
1.010 × 10−10 | 6.5 × 10−12 | 2.57 | 0.19 | 2.90 | 0.12 | 0.01 | B | 5703 | 5253 | 5003 | 33 | This paper | |
3 Juno | 2.09 × 10−11 | 3.5 × 10−12 | 4.65 | 0.84 | 3.56 | −0.31 | −0.06 | S | 3205 | 2674 | 2005 | 34 | Chernetenko & Kochetova (2002) |
1.34 × 10−11 | 2.3 × 10−12 | 2.98 | 0.55 | 3.56 | 0.16 | 0.03 | S | 3205 | 2674 | 2005 | 34 | Baer et al. (2008) | |
1.44 × 10−11 | 2.3 × 10−12 | 3.20 | 0.56 | 3.56 | 0.10 | 0.02 | S | 3205 | 2674 | 2005 | 34 | This paper | |
4 Vesta | 1.396 × 10−10 | 4.3 × 10−12 | 3.58 | 0.23 | 3.56 | −0.01 | 0.00 | V | 5786 | 5606 | 4586 | 56 | Sitarski & Todororovic-Juchniewicz (1995) |
1.690 × 10−10 | 5.0 × 10−12 | 4.33 | 0.28 | 3.56 | −0.22 | −0.01 | V | 5786 | 5606 | 4586 | 56 | Hilton (1999) | |
1.360 × 10−10 | 5.0 × 10−12 | 3.49 | 0.24 | 3.56 | 0.02 | 0.00 | V | 5786 | 5606 | 4586 | 56 | Michalak (2000) | |
1.306 × 10−10 | 1.6 × 10−12 | 3.35 | 0.19 | 3.56 | 0.06 | 0.00 | V | 5786 | 5606 | 4586 | 56 | Viateau & Rapaport (2001) | |
1.290 × 10−10 | 8.0 × 10−12 | 3.90 | 0.24 | 3.56 | −0.10 | −0.01 | V | 5017 | 5017 | 5017 | 07 | Kovačević (2005) | |
1.310 × 10−10 | 2.0 × 10−12 | 3.96 | 0.06 | 3.56 | −0.11 | 0.00 | V | 5017 | 5017 | 5017 | 07 | Kovačević (2005) | |
1.120 × 10−10 | 5.0 × 10−12 | 3.39 | 0.15 | 3.56 | 0.05 | 0.00 | V | 5017 | 5017 | 5017 | 07 | Kovačević (2005) | |
1.120 × 10−10 | 1.7 × 10−11 | 3.39 | 0.51 | 3.56 | 0.05 | 0.01 | V | 5017 | 5017 | 5017 | 07 | Kovačević (2005) | |
1.319 × 10−10 | 3.0 × 10−13 | 3.38 | 0.19 | 3.56 | 0.05 | 0.00 | V | 5786 | 5606 | 4586 | 56 | Baer et al. (2008) | |
1.300 × 10−10 | 1.0 × 10−13 | 3.33 | 0.19 | 3.56 | 0.06 | 0.00 | V | 5786 | 5606 | 4586 | 56 | Kuzmanoski et al. (2010) | |
1.300 × 10−10 | 5.3 × 10−13 | 3.33 | 0.19 | 3.56 | 0.06 | 0.00 | V | 5786 | 5606 | 4586 | 56 | This paper | |
6 Hebe | 6.90 × 10−12 | 2.2 × 10−12 | 4.07 | 1.31 | 3.72 | −0.09 | −0.03 | S | 2059 | 1858 | 1709 | 1.458 | Michalak (2001) |
6.90 × 10−12 | 9.0 × 10−13 | 4.07 | 0.56 | 3.72 | −0.09 | −0.01 | S | 2059 | 1858 | 1709 | 1.458 | Kochetova (2004) | |
6.46 × 10−12 | 3.2 × 10−13 | 3.81 | 0.26 | 3.72 | −0.02 | 0.00 | S | 2059 | 1858 | 1709 | 1.458 | Baer et al. (2008) | |
6.40 × 10−12 | 6.7 × 10−13 | 3.77 | 0.43 | 3.72 | −0.01 | 0.00 | S | 2059 | 1858 | 1709 | 1.458 | This paper | |
7 Iris | 1.41 × 10−11 | 1.4 × 10−12 | 5.58 | 0.96 | 3.56 | −0.57 | −0.10 | S | 24010 | 2008 | 20010 | 58 | Chernetenko & Kochetova (2002) |
6.86 × 10−12 | 5.0 × 10−13 | 2.72 | 0.43 | 3.56 | 0.24 | 0.04 | S | 24010 | 2008 | 20010 | 58 | Baer et al. (2008) | |
8.12 × 10−12 | 4.6 × 10−13 | 3.21 | 0.49 | 3.56 | 0.10 | 0.01 | S | 24010 | 2008 | 20010 | 58 | This paper | |
8 Flora | 4.26 × 10−12 | 4.5 × 10−13 | 3.89 | 1.76 | 3.56 | −0.09 | −0.04 | S | 160.811 | 160.811 | 160.811 | 11.812 | Baer et al. (2008) |
3.33 × 10−12 | 4.2 × 10−13 | 3.04 | 1.39 | 3.56 | 0.14 | 0.07 | S | 160.811 | 160.811 | 160.811 | 11.812 | This paper | |
9 Metis | 5.7 × 10−12 | 1.4 × 10−12 | 4.12 | 1.33 | 3.72 | −0.11 | −0.03 | S | 22213 | 18213 | 13013 | 613 | Baer et al. (2008) |
5.7 × 10−12 | 1.1 × 10−12 | 4.12 | 1.17 | 3.72 | −0.11 | −0.03 | S | 22213 | 18213 | 13013 | 613 | This paper | |
10 Hygiea | 5.570 × 10−11 | 7.00 × 10−12 | 2.65 | 0.36 | 2.46 | −0.08 | −0.01 | C | 53010 | 4078 | 37010 | 3.48 | Michalak (2001) |
5.010 × 10−11 | 4.1 × 10−12 | 2.39 | 0.23 | 2.46 | 0.03 | 0.00 | C | 53010 | 4078 | 37010 | 3.48 | Chernetenko & Kochetova (2002) | |
4.540 × 10−11 | 1.3 × 10−12 | 2.16 | 0.12 | 2.46 | 0.12 | 0.01 | C | 53010 | 4078 | 37010 | 3.48 | Chesley et al. (2005) | |
4.450 × 10−11 | 7.0 × 10−13 | 2.12 | 0.11 | 2.46 | 0.14 | 0.01 | C | 53010 | 4078 | 37010 | 3.48 | Baer et al. (2008) | |
2.500 × 10−11 | 4.0 × 10−12 | 1.19 | 0.20 | 2.46 | 0.52 | 0.09 | C | 53010 | 4078 | 37010 | 3.48 | Ivantsov (2008) | |
4.358 × 10−11 | 7.4 × 10−13 | 2.08 | 0.10 | 2.46 | 0.16 | 0.01 | C | 53010 | 4078 | 37010 | 3.48 | This paper | |
11 Parthenope | 2.58 × 10−12 | 1.0 × 10−13 | 2.74 | 0.20 | 3.56 | 0.23 | 0.02 | S | 1538 | 1538 | 1538 | 1.558 | Viateau & Rapaport (1997b) |
2.560 × 10−12 | 7.0 × 10−14 | 2.72 | 0.18 | 3.56 | 0.24 | 0.02 | S | 1538 | 1538 | 1538 | 1.558 | Viateau & Rapaport (2001) | |
3.090 × 10−12 | 2.0 × 10−14 | 3.28 | 0.20 | 3.56 | 0.08 | 0.00 | S | 1538 | 1538 | 1538 | 1.558 | Baer et al. (2008) | |
13 Egeria | 8.2 × 10−12 | 1.6 × 10−12 | 3.46 | 0.79 | 2.90 | −0.19 | −0.04 | G | 2088 | 2088 | 2088 | 4.158 | Baer et al. (2008) |
8.0 × 10−12 | 2.2 × 10−12 | 3.38 | 1.01 | 2.90 | −0.17 | −0.05 | G | 2088 | 2088 | 2088 | 4.158 | This paper | |
14 Irene | 4.13 × 10−12 | 7.3 × 10−13 | 4.42 | 1.59 | 3.56 | −0.24 | −0.09 | S | 16714 | 15214 | 13914 | 8 | Baer et al. (2008) |
3.49 × 10−12 | 8.2 × 10−13 | 3.73 | 1.47 | 3.56 | −0.05 | −0.02 | S | 16714 | 15214 | 13914 | 8 | This paper | |
15 Eunomia | 1.260 × 10−11 | 3.00 × 10−12 | 2.48 | 0.72 | 3.72 | 0.33 | 0.10 | S | 35710 | 2558 | 21210 | 7.58 | Michalak (2001) |
1.220 × 10−11 | 1.6 × 10−12 | 2.40 | 0.51 | 3.72 | 0.35 | 0.08 | S | 35710 | 2558 | 21210 | 7.58 | Chernetenko & Kochetova (2002) | |
1.060 × 10−11 | 1.6 × 10−12 | 2.09 | 0.47 | 3.72 | 0.44 | 0.10 | S | 35710 | 2558 | 21210 | 7.58 | Kochetova (2004) | |
1.640 × 10−11 | 6.0 × 10−13 | 3.23 | 0.55 | 3.72 | 0.13 | 0.02 | S | 35710 | 2558 | 21210 | 7.58 | Vitagliano & Stoss (2006) | |
8.000 × 10−12 | 3.0 × 10−12 | 1.58 | 0.65 | 3.72 | 0.58 | 0.24 | S | 35710 | 2558 | 21210 | 7.58 | Ivantsov (2008) | |
1.570 × 10−11 | 2.0 × 10−13 | 3.09 | 0.52 | 3.72 | 0.17 | 0.03 | S | 35710 | 2558 | 21210 | 7.58 | Baer et al. (2008) | |
1.620 × 10−11 | 5.0 × 10−13 | 3.19 | 0.54 | 3.72 | 0.14 | 0.02 | S | 35710 | 2558 | 21210 | 7.58 | Zielenbach (2010) | |
1.597 × 10−11 | 1.5 × 10−13 | 3.14 | 0.53 | 3.72 | 0.15 | 0.03 | S | 35710 | 2558 | 21210 | 7.58 | This paper | |
16 Psyche | 8.700 × 10−12 | 2.6 × 10−12 | 5.14 | 2.78 | 7.50 | 0.32 | 0.17 | M | 24016 | 18516 | 14516 | 1416 | Viateau (2000) |
3.380 × 10−11 | 2.8 × 10−12 | 6.98 | 0.58 | 7.50 | 0.07 | 0.01 | M | 26415 | 26415 | 26415 | 0 | Kuzmanoski & Kovačević (2002) | |
1.340 × 10−11 | 2.2 × 10−12 | 7.91 | 3.80 | 7.50 | −0.05 | −0.03 | M | 24016 | 18516 | 14516 | 1416 | Kochetova (2004) | |
1.100 × 10−11 | 4.0 × 10−13 | 6.49 | 2.94 | 7.50 | 0.13 | 0.06 | M | 24016 | 18516 | 14516 | 1416 | Baer et al. (2008) | |
1.140 × 10−11 | 4.2 × 10−13 | 6.73 | 3.05 | 7.50 | 0.10 | 0.05 | M | 24016 | 18516 | 14516 | 1416 | This paper | |
17 Thetis | 5.91 × 10−13 | 3.8 × 10−14 | 2.37 | 0.31 | 3.56 | 0.33 | 0.04 | S | 1179 | 908 | 909 | 1.858 | Baer et al. (2008) |
7.21 × 10−13 | 2.5 × 10−14 | 2.89 | 0.34 | 3.56 | 0.19 | 0.02 | S | 1179 | 908 | 909 | 1.858 | This paper | |
18 Melpomene | 1.51 × 10−12 | 5.1 × 10−13 | 1.69 | 0.66 | 3.56 | 0.53 | 0.21 | S | 17013 | 15513 | 1299 | 513 | Baer et al. (2008) |
19 Fortuna | 6.38 × 10−12 | 2.5 × 10−13 | 2.70 | 0.48 | 2.90 | 0.07 | 0.01 | G | 22513 | 20513 | 19513 | 613 | Baer et al. (2008) |
4.18 × 10−12 | 3.6 × 10−13 | 1.77 | 0.34 | 2.90 | 0.39 | 0.08 | G | 22513 | 20513 | 19513 | 613 | This paper | |
20 Massalia | 2.40 × 10−12 | 4.0 × 10−13 | 2.98 | 0.76 | 3.56 | 0.16 | 0.04 | S | 16010 | 1458 | 13210 | 4.658 | Bange (1998) |
2.85 × 10−12 | 4.1 × 10−13 | 3.54 | 0.85 | 3.56 | 0.01 | 0.00 | S | 16010 | 1458 | 13210 | 4.658 | Baer et al. (2008) | |
1.68 × 10−12 | 3.5 × 10−13 | 2.08 | 0.59 | 3.56 | 0.41 | 0.12 | S | 16010 | 1458 | 13210 | 4.658 | This paper | |
21 Lutetia | 1.29 × 10−12 | 1.2 × 10−13 | 4.21 | 1.61 | 3.70 | −0.14 | −0.05 | M | 12441 | 10141 | 9341 | 6.541 | Baer et al. (2008) |
1.31 × 10−12 | 4.4 × 10−13 | 4.27 | 2.14 | 3.70 | −0.16 | −0.08 | M | 12441 | 10141 | 9341 | 6.541 | This paper | |
22 Kalliope | 3.700 × 10−12 | 2.22 × 10−13 | 2.37 | 0.38 | 3.56 | 0.33 | 0.05 | M | 1818 | 1818 | 1818 | 4.58 | Margot & Brown (2003) |
Note: Binary. Mass stated for system | |||||||||||||
4.100 × 10−12 | 1.30 × 10−13 | 3.35 | 0.33 | 3.56 | 0.06 | 0.01 | M | 23517 | 16417 | 12417 | 2.817 | Descamps et al. (2008) | |
2817 | 2817 | 2817 | 217 | Note: Binary. Mass stated for primary | |||||||||
4.070 × 10−12 | 1.00 × 10−13 | 2.80 | 0.20 | 3.56 | 0.21 | 0.02 | M | 24318 | 19618 | 17518 | 1018 | Marchis et al. (2008a) | |
2018 | 2018 | 2018 | Note: Binary. Mass stated for system | ||||||||||
24 Themis | 2.89 × 10−12 | 1.26 × 10−12 | 1.42 | 0.75 | 2.46 | 0.42 | 0.23 | C | 19819 | 19819 | 19819 | 10 | Lopez Garcia et al. (1997) |
5.67 × 10−12 | 2.15 × 10−12 | 2.78 | 1.35 | 2.46 | −0.13 | −0.06 | C | 19819 | 19819 | 19819 | 10 | Baer & Chesley (2008) | |
29 Amphitrite | 7.70 × 10−12 | 1.3 × 10−12 | 3.07 | 0.60 | 3.56 | 0.14 | 0.03 | S | 23310 | 2128 | 19310 | 3.48 | Kochetova (2004) |
5.92 × 10−12 | 3.0 × 10−13 | 2.36 | 0.26 | 3.56 | 0.34 | 0.04 | S | 23310 | 2128 | 19310 | 3.48 | Baer et al. (2008) | |
7.63 × 10−12 | 3.1 × 10−13 | 3.04 | 0.32 | 3.56 | 0.15 | 0.02 | S | 23310 | 2128 | 19310 | 3.48 | This paper | |
31 Euphrosyne | 9.40 × 10−12 | 5.2 × 10−12 | 2.13 | 1.21 | 2.90 | 0.27 | 0.15 | C | 25610 | 2568 | 25610 | 5.758 | Kochetova (2004) |
3.13 × 10−11 | 5.9 × 10−12 | 7.09 | 1.64 | 2.90 | −1.45 | −0.33 | C | 25610 | 2568 | 25610 | 5.758 | Baer et al. (2008) | |
2.92 × 10−11 | 9.9 × 10−12 | 6.61 | 2.41 | 2.90 | −1.28 | −0.47 | C | 25610 | 2568 | 25610 | 5.758 | This paper | |
39 Laetitia | 2.83 × 10−12 | 7.3 × 10−13 | 3.19 | 0.99 | 3.56 | 0.10 | 0.03 | S | 21010 | 1508 | 10710 | 4.38 | This paper |
45 Eugenia | 3.00 × 10−12 | 1.0 × 10−13 | 1.14 | 0.08 | 2.90 | 0.61 | 0.04 | FC | 30810 | 2208 | 14710 | 2.18 | Merline et al. (1999) Note: Binary |
2.860 × 10−12 | 6.0 × 10−14 | 1.10 | 0.10 | 2.90 | 0.62 | 0.06 | FC | 23218 | 19318 | 16118 | 818 | Marchis et al. (2008a) | |
718 | 718 | 718 | Note: Binary. Mass stated for system | ||||||||||
47 Aglaja | 1.09 × 10−12 | 4.3 × 10−13 | 2.02 | 0.88 | 2.90 | 0.30 | 0.13 | C | 1278 | 1278 | 1278 | 3.858 | Baer et al. (2008) |
48 Doris | 6.1 × 10−12 | 3.0 × 10−12 | 2.12 | 1.07 | 2.90 | 0.27 | 0.13 | CG | 221.88 | 221.88 | 221.88 | 3.88 | Kochetova (2004) |
49 Pales | 1.35 × 10−12 | 2.5 × 10−13 | 1.52 | 0.30 | 2.90 | 0.48 | 0.10 | CG | 1508 | 1508 | 1508 | 1.98 | Baer et al. (2008) |
52 Europa | 2.61 × 10−11 | 8.8 × 10−12 | 3.60 | 1.23 | 2.90 | −0.24 | −0.08 | CF | 36210 | 3028 | 25210 | 2.78 | Michalak (2001) |
1.28 × 10−11 | 2.5 × 10−12 | 1.77 | 0.36 | 2.90 | 0.39 | 0.08 | CF | 36210 | 3028 | 25210 | 2.78 | Chernetenko & Kochetova (2002) | |
8.29 × 10−12 | 8.1 × 10−13 | 1.14 | 0.13 | 2.90 | 0.61 | 0.07 | CF | 36210 | 3028 | 25210 | 2.78 | Baer et al. (2008) | |
4.20 × 10−11 | 1.1 × 10−11 | 5.79 | 1.55 | 2.90 | −1.00 | −0.27 | CF | 36210 | 3028 | 25210 | 2.78 | Ivantsov (2008) | |
1.139 × 10−11 | 7.9 × 10−13 | 1.57 | 0.14 | 2.90 | 0.46 | 0.04 | CF | 36210 | 3028 | 25210 | 2.78 | This paper | |
65 Cybele | 5.80 × 10−12 | 1.5 × 10−12 | 1.08 | 0.30 | 2.46 | 0.56 | 0.15 | P | 30220 | 29020 | 23220 | 420 | Chernetenko & Kochetova (2002) |
8.93 × 10−12 | 6.1 × 10−13 | 1.67 | 0.19 | 2.46 | 0.32 | 0.04 | P | 30220 | 29020 | 23220 | 420 | Baer et al. (2008) | |
5.30 × 10−12 | 9.6 × 10−13 | 0.99 | 0.20 | 2.46 | 0.60 | 0.12 | P | 30220 | 29020 | 23220 | 420 | This paper | |
87 Sylvia | 7.340 × 10−12 | 5.03 × 10−13 | 1.20 | 0.15 | 2.46 | 0.51 | 0.06 | P | 38421 | 26221 | 23221 | 521 | Margot & Brown (2001) |
Note: Binary. Mass stated for primary | |||||||||||||
7.431 × 10−12 | 3.0 × 10−14 | 1.21 | 0.13 | 2.46 | 0.51 | 0.05 | P | 38421 | 26221 | 23221 | 521 | Marchis et al. (2005a) | |
Note: Binary. Mass stated for primary | |||||||||||||
88 Thisbe | 7.40 × 10−12 | 1.3 × 10−12 | 2.46 | 0.59 | 2.90 | 0.15 | 0.04 | CF | 2559 | 23222 | 1939 | 622 | Michalak (2001) |
5.90 × 10−12 | 1.2 × 10−12 | 1.96 | 0.51 | 2.90 | 0.32 | 0.08 | CF | 2559 | 23222 | 1939 | 622 | Kochetova (2004) | |
5.30 × 10−12 | 9.0 × 10−13 | 1.76 | 0.41 | 2.90 | 0.39 | 0.09 | CF | 2559 | 23222 | 1939 | 622 | Baer et al. (2008) | |
9.19 × 10−12 | 5.5 × 10−13 | 3.06 | 0.52 | 2.90 | −0.05 | −0.01 | CF | 2559 | 23222 | 1939 | 622 | This paper | |
90 Antiope | 2.060 × 10−13 | 6.0 × 10−14 | 1.24 | 0.37 | 2.90 | 0.57 | 0.17 | C | 85.823 | 85.823 | 85.823 | 123 | Merline et al. (2002) |
2.060 × 10−13 | 6.0 × 10−14 | 1.24 | 0.37 | 2.90 | 0.57 | 0.17 | C | 85.823 | 85.823 | 85.823 | 123 | Note: Binary. Mass stated for each component | |
4.173 × 10−13 | 1.0 × 10−14 | 1.25 | 0.05 | 2.90 | 0.57 | 0.02 | C | 9324 | 8724 | 83.624 | 0.524 | Descamps et al. (2007) | |
89.424 | 82.824 | 79.624 | 0.524 | Note: Binary. Mass stated for system | |||||||||
107 Camilla | 5.63 × 10−12 | 1.5 × 10−13 | 1.40 | 0.30 | 3.41 | 0.59 | 0.13 | C | 3449 | 24618 | 2059 | 718 | Marchis et al. (2008a) |
1618 | 1618 | 1618 | 618 | Note: Binary. Mass stated for system | |||||||||
121 Hermione | 4.700 × 10−12 | 8.00 × 10−13 | 1.96 | 0.61 | 2.90 | 0.32 | 0.10 | C | 26825 | 18625 | 18325 | 925 | Viateau (2000) |
2.705 × 10−12 | 1.50 × 10−13 | 1.13 | 0.30 | 2.90 | 0.61 | 0.16 | C | 26825 | 18625 | 18325 | 925 | Marchis et al. (2005b) | |
2.363 × 10−12 | 1.006 × 10−13 | 1.37 | 0.14 | 2.90 | 0.53 | 0.06 | C | 18726 | 18726 | 18726 | 326 | Descamps et al. (2009) | |
130 Elektra | 3.32 × 10−12 | 2.0 × 10−13 | 1.30 | 0.30 | 2.90 | 0.55 | 0.13 | G | 10827 | 10827 | 10827 | 7.527 | Marchis et al. (2008b) Note: Binary |
189 Phthia | 1.93 × 10−14 | 4.1 × 10−15 | 1.34 | 0.51 | 3.56 | 0.62 | 0.24 | S | 388 | 388 | 388 | 28 | Baer et al. (2008) |
243 Ida | 1.90 × 10−14 | 1.0 × 10−15 | 2.56 | 0.33 | 3.54 | 0.28 | 0.04 | S | 59.828 | 25.428 | 18.628 | 0.628 | Petit et al. (1997) |
253 Mathilde | 5.194 × 10−14 | 2.20 × 10−15 | 1.35 | 0.21 | 2.90 | 0.53 | 0.08 | C | 6630 | 4830 | 4630 | 1.329 | Yeomans et al. (1997) |
283 Emma | 6.938 × 10−13 | 1.51 × 10−14 | 0.81 | 0.08 | 2.90 | 0.72 | 0.07 | P | 1488 | 1488 | 1488 | 2.38 | Marchis et al. (2008b) Note: Binary |
379 Huenna | 1.926 × 10−13 | 1.00 × 10−14 | 0.93 | 0.07 | 2.90 | 0.68 | 0.05 | C | 92.38 | 92.38 | 92.38 | 0.858 | Marchis et al. (2008b) Note: Binary |
433 Eros | 3.362 × 10−15 | 1.5 × 10−18 | 2.67 | 0.03 | 3.56 | 0.25 | 0.00 | S | 34.431 | 11.231 | 11.231 | 0.131 | Yeomans et al. (2000) |
444 Gyptis | 3.6 × 10−12 | 1.6 × 10−12 | 3.16 | 1.52 | 2.90 | −0.09 | −0.04 | C | 1638 | 1638 | 1638 | 58 | Michalak (2001) |
6.3 × 10−12 | 1.2 × 10−12 | 5.53 | 1.46 | 2.90 | −0.91 | −0.24 | C | 1638 | 1638 | 1638 | 58 | Kochetova (2004) | |
451 Patientia | 1.02 × 10−11 | 3.4 × 10−12 | 3.40 | 1.20 | 2.90 | −0.17 | −0.06 | CU | 2258 | 2258 | 2258 | 4.48 | Kochetova (2004) |
511 Davida | 3.340 × 10−11 | 2.8 × 10−12 | 5.24 | 2.32 | 2.90 | −0.81 | −0.36 | C | 35732 | 29432 | 23132 | 2132 | Michalak (2001) |
2.400 × 10−11 | 2.4 × 10−12 | 3.76 | 1.68 | 2.90 | −0.30 | −0.13 | C | 35732 | 29432 | 23132 | 2132 | Chernetenko & Kochetova (2002) | |
2.200 × 10−11 | 1.0 × 10−12 | 3.45 | 1.51 | 2.90 | −0.19 | −0.08 | C | 35732 | 29432 | 23132 | 2132 | Baer et al. (2008) | |
1.896 × 10−11 | 9.9 × 10−13 | 2.97 | 1.30 | 2.90 | −0.02 | 0.01 | C | 35732 | 29432 | 23132 | 2132 | This paper | |
532 Herculina | 1.68 × 10−11 | 2.8 × 10−12 | 5.80 | 1.17 | 3.56 | −0.63 | −0.13 | S | 222.48 | 222.48 | 222.48 | 4.28 | Kochetova (2004) |
617 Patroclus | 6.84 × 10−13 | 5.5 × 10−14 | 0.80 | 0.08 | 2.12 | 0.62 | 0.06 | P | 121.833 | 121.833 | 121.833 | 1.633 | Marchis et al. (2006) |
112.633 | 112.633 | 112.633 | 1.633 | Note: Binary. Mass stated for system | |||||||||
6.033 × 10−13 | 1.006 × 10−13 | 1.08 | 0.33 | 2.12 | 0.49 | 0.15 | P | 10634 | 10634 | 10634 | 5.534 | Mueller et al. (2010) | |
9834 | 9834 | 9834 | 534 | Note: Binary. Mass stated for system | |||||||||
702 Alauda | 3.04 × 10−12 | 2.0 × 10−13 | 1.56 | 0.19 | 2.90 | 0.46 | 0.05 | C | 194.738 | 194.738 | 194.738 | 3.28 | Margot & Rojo (2007) |
5.535 | 5.535 | 5.535 | Note: Binary | ||||||||||
704 Interamnia | 3.70 × 10−11 | 1.7 × 10−11 | 4.34 | 2.19 | 2.90 | −0.50 | −0.25 | F | 34942 | 33942 | 27442 | 1142 | Landgraff (1992) |
3.52 × 10−11 | 9.3 × 10−12 | 4.13 | 1.39 | 2.90 | −0.42 | −0.14 | F | 34942 | 33942 | 27442 | 1142 | Michalak (2001) | |
8.10 × 10−12 | 4.2 × 10−12 | 0.95 | 0.53 | 2.90 | 0.67 | 0.38 | F | 34942 | 33942 | 27442 | 1142 | Chernetenko & Kochetova (2002) | |
1.86 × 10−11 | 1.1 × 10−12 | 2.18 | 0.47 | 2.90 | 0.25 | 0.05 | F | 34942 | 33942 | 27442 | 1142 | Baer et al. (2008) | |
5.70 × 10−11 | 1.6 × 10−11 | 6.68 | 2.33 | 2.90 | −1.30 | −0.45 | F | 34942 | 33942 | 27442 | 1142 | Ivantsov (2008) | |
1.950 × 10−11 | 8.9 × 10−13 | 2.29 | 0.48 | 2.90 | 0.21 | 0.04 | F | 34942 | 33942 | 27442 | 1142 | This paper | |
762 Pulcova | 1.300 × 10−12 | 2.0 × 10−13 | 1.92 | 0.32 | 2.90 | 0.34 | 0.06 | F | 1378 | 1378 | 1378 | 1.68 | Merline et al. (2002) |
7.04 × 10−13 | 5.0 × 10−14 | 0.90 | 0.10 | 2.90 | 0.69 | 0.08 | F | 1378 | 1378 | 1378 | 1.68 | Marchis et al. (2008a) | |
2018 | 2018 | 2018 | Note: Binary. Mass stated for system | ||||||||||
804 Hispania | 5.00 × 10−12 | 4.0 × 10−12 | 4.82 | 3.89 | 2.46 | −0.96 | −0.77 | PC | 1588 | 1588 | 1588 | 2.98 | Landgraff (1992) |
2.02 × 10−12 | 4.3 × 10−13 | 1.95 | 0.47 | 2.46 | 0.21 | 0.05 | PC | 1588 | 1588 | 1588 | 2.98 | Baer et al. (2008) | |
1.75 × 10−12 | 4.0 × 10−13 | 1.69 | 0.43 | 2.46 | 0.31 | 0.08 | PC | 1588 | 1588 | 1588 | 2.98 | This paper | |
3749 Balam | 2.56 × 10−16 | 1.0 × 10−17 | 2.61 | 0.45 | 3.56 | 0.27 | 0.05 | S | 7.227 | 7.227 | 7.227 | 0.227 | Marchis et al. (2008b) Note: Binary |
25143 Itokawa | 1.760 × 10−20 | 5.3 × 10−22 | 1.90 | 0.13 | 3.56 | 0.47 | 0.03 | S | 0.53536 | 0.29436 | 0.20936 | 0.00 | Fujiwara et al. (2006) |
1999 KW4 | 1.183 × 10−18 | 5.0 × 10−20 | 1.97 | 0.24 | 3.56 | 0.45 | 0.05 | S | 1.53237 | 1.49537 | 1.34737 | 0.0437 | Ostro et al. (2006) |
(66391) | 6.788 × 10−20 | 1.21 × 10−20 | 2.81 | 0.82 | 0.57137 | 0.46337 | 0.34937 | 0.03437 | Note: Binary. Mass stated for each component | ||||
2000 DP107 | 2.300 × 10−19 | 2.5 × 10−20 | 1.71 | 1.04 | 2.90 | 0.41 | 0.25 | C | 0.838 | 0.838 | 0.838 | 0.0838 | Margot et al. (2002) |
(185851) | 1.211 × 10−20 | 1.316 × 10−21 | 1.70 | 1.04 | 2.90 | 0.41 | 0.25 | C | 0.338 | 0.338 | 0.338 | 0.0338 | Note: Binary. Mass stated for each component |
2000 UG11 | 4.70 × 10−21 | 8.0 × 10−22 | 1.47 | 0.81 | 0.2338 | 0.2338 | 0.2338 | 0.0238 | Margot et al. (2002) | ||||
2002 CE26 | 1.95 × 10−17 | 2.5 × 10−18 | 1.73 | 0.63 | 2.90 | 0.40 | 0.15 | C | 3.539 | 3.539 | 3.539 | 0.239 | Shepard et al. (2006) |
2003 YT1 | 6.38 × 10−19 | 2.00 × 10−19 | 2.01 | 0.7 | 0.8640 | 0.8640 | 0.8640 | 0.540 | Brooks (2006) |
References. (1) Neese (2006). (2) Thomas et al. (2005). (3) Dunham et al. (1990). (4) Millis et al. (1981). (5) Kaasalainen et al. (2002). (6) Thomas et al. (1997). (7) Kovačević (2005). (8) Tedesco et al. (2002). (9) Torppa et al. (2003). (10) Kaasalainen et al. (2002). (11) Shevchenko & Tedesco (2006). (12) Shevchenko & Tedesco (2007). (13) Storrs et al. (2005). (14) Yeomans (2010a). (15) Kuzmanoski & Kovačević (2002). (16) Shepard et al. (2008). (17) Descamps et al. (2008). (18) Marchis et al. (2008a). (19) Yeomans (2010b). (20) Müller & Blommaert (2004). (21) Marchis et al. (2005a). (22) Millis et al. (1983). (23) Merline et al. (2002). (24) Descamps et al. (2007). (25) Marchis et al. (2005b). (26) Descamps et al. (2009). (27) Marchis et al. (2008b). (28) Thomas et al. (1996). (29) Yeomans et al. (1997). (30) Veverka et al. (1997). (31) Veverka et al. (2000). (32) Conrad et al. (2007). (33) Marchis et al. (2006). (34) Mueller et al. (2010). (35) Margot & Rojo (2007). (36) Fujiwara et al. (2006). (37) Ostro et al. (2006). (38) Margot et al. (2002). (39) Shepard et al. (2006). (40) Brooks (2006). (41) Drummond et al. (2010). (42) Drummond et al. (2009).
Furthermore, with the help of D. Britt (2010, private communication; Consolmagno et al. 2008), we have made assumptions for each asteroid's mineral grain density, based on the meteorite class that represents the closest analog to each asteroid's taxonomic class. This allows estimation of each asteroid's bulk porosity; and since a negative bulk porosity is impossible, this derived quantity can help indicate unrealistic results. In general,
- 1.S-class asteroids are modeled using L chondrites;
- 2.G, B, F, and inner-belt C-class asteroids are modeled using CM chondrites;
- 3.P, D, and outer-belt C-class asteroids are modeled using CI chondrites;
- 4.M-class asteroids are modeled as nickel-irons.
Absent samples returned from these bodies, however, the assumed grain densities must be recognized as an unmodeled source of uncertainty in the resulting bulk porosities. Additionally, while we have used the best available data on each asteroid's size and shape, we must note that the calculated bulk densities and porosities assume that there are no significant concavities that would reduce the asteroid's volume and increase its bulk density. This should be recognized as another unmodeled source of uncertainty in the resulting derived quantities.
Several of our results merit commentary.
1 Ceres, 2 Pallas, and 4 Vesta. The masses of these asteroids are already well determined; our results here are given simply for comparison purposes. We have assumed a "dusty ice" composition for 1 Ceres, a CM analog for 2 Pallas, and an L chondrite analog for 4 Vesta; the resulting small porosities are consistent with monolithic bodies.
3 Juno. The mass of 3 Juno is less certain, owing to its relatively high inclination, which limits close interactions with other bodies. An L chondrite analog yields a bulk porosity of approximately 10%, suggesting that this large asteroid is not completely monolithic.
6 Hebe. Dynamical studies suggest that 6 Hebe, lying near the 3:1 mean motion and v6 secular resonances, is a significant source of chondritic meteorites (Farinella et al. 1993). However, there is disagreement as to whether 6 Hebe may be the source of the H chondrite and IIE iron meteorites (Gaffey & Gilbert 1998), or whether its surface contains hydrated minerals, which would exclude the heating necessary to create stony-irons (Rivkin et al. 2001). The SkyMorph precovery observations allowed us to obtain a mass for this asteroid. While the resulting bulk density closely matches an H chondrite analog, the uncertainty is such that an L chondrite analog cannot be excluded.
8 Flora. Also bordering the v6 secular resonance, 8 Flora is the largest member of a dynamical family believed to result from the catastrophic disruption of a larger parent body a few hundred million years ago (Nesvorný et al. 2002). Spectroscopic studies further suggest that 8 Flora may be a significant source of both LL chondrites and NEAs (Vernazza et al. 2008). Once again, the SkyMorph precovery observations allowed us to calculate the mass of this body. Assuming an LL chondrite analog, we derive a bulk porosity of approximately 14%, consistent with an impact-fractured body.
9 Metis. 9 Metis has been classified as S-class, leading one to expect a chondritic analog. While the mass of 9 Metis obtained from the encounter with asteroid 43 leads to a bulk density of approximately 3.0 g cm−3, the masses resulting from the encounters with asteroids 20 and 29,818 lead to much higher densities and negative bulk porosities, suggesting that these masses are inconsistent with its taxonomic classification.
Based on dynamical family classification and spectroscopic analysis, Kelley & Gaffey (2000) have suggested that 9 Metis is a mantle/core fragment of a 300–600 km diameter differentiated parent body, whose other surviving fragments include 113 Amalthea. Spectroscopic modeling suggests that the composition of 9 Metis is 50% olivine, and 50% nickel-iron, which would lead to an average bulk density of approximately 5.37 g cm−3.
Our weighted mean values of mass and bulk density are inconsistent with this prediction. Given the spread in our mass values, however, we are frankly unable to confirm or refute this hypothesis.
21 Lutetia. This asteroid is listed as M class, but there is considerable disagreement as to its composition. Recent spectroscopic analyses in support of the Rosetta flyby have suggested that its surface is more consistent with carbonaceous chondrites (Carvano et al. 2008), or even enstatite chondrites (Vernazza et al. 2009). Our bulk density value appears a fair match for enstatite chondrite; and we have adopted an EH enstatite chondrite analog, yielding a slightly negative bulk porosity. However, given the uncertainty in our bulk density, a CM/CI chondrite or porous nickel-iron analog cannot be excluded.
31 Euphrosyne. A comparison of the reflectance spectra of several carbonaceous chondrites with that of 31 Euphrosyne reinforces its classification as C class (Hiroi et al. 1993). However, our measured mass yields a bulk density that is inconsistent with a C-class asteroid. We would note that our mass results from only a single encounter with 3176 Paolicchi; we must therefore consider this mass suspect.
39 Laetitia. The dimensions of 39 Laetitia, which are derived from IRAS and light-curve studies, are consistent with several occultation observations (Dunham & Herald 2009). Gaffey et al. (1993) have classified this as an S(II) asteroid, with a high ratio of olivine to calcic pyroxene; we have therefore used an L chondrite analog, yielding a very reasonable porosity.
52 Europa. There have been multiple published masses for 52 Europa, but they tend to vary widely. Our new mass is somewhat larger than our previous value and slightly smaller than that of Chernetenko & Kochetova (2002). This asteroid is classified as CF class, so we have used a CM analog; this implies a significant porosity, suggesting 52 Europa may be a rubble pile.
704 Interamnia. This asteroid is classified as F class, and Hiroi et al. (1993) suggest a good match to carbonaceous chondrites. We have therefore used a CM analog.
Again, there is considerable spread in the reported masses for 704 Interamnia. Our new value lies within 1σ of our previous value and while fairly close to that of Chernetenko & Kochetova (2002), our new value suggests a significantly smaller porosity.
5. POROSITY IMPLICATIONS
The data in Table 3 yield a sampling of the physical properties of over 50 main-belt asteroids. When the derived bulk porosities are plotted against effective diameter in Figure 1, an interesting observation can be made.
Figure 1. Bulk porosity as a function of effective diameter for the asteroids in Table 3.
Download figure:
Standard image High-resolution imageThe very largest asteroids are either monolithic or fractured, with porosities less than 20%. As an asteroid's size decreases below 300 km, however, an abrupt change occurs; and we observe a wide range of porosities, from near zero up to 70% (or more). Significantly, approximately half of the asteroids smaller than 300 km in diameter would be characterized as "rubble piles," with porosities in excess of 30%.
Once asteroids cooled soon after formation, their porosities could only be altered dynamically, either by collisions or by the YORP effect, but the YORP effect is only noticeable over the age of the solar system for minor planets smaller than 10 km in diameter (Rubincam 2000); thus changes in porosity due to YORP-driven rotational torques could only be significant for the very smallest asteroids.
We estimate, therefore, that asteroids as large as 300 km in diameter must have experienced collisions that were sufficiently violent as to disrupt them, leaving them to recombine subsequently as loose aggregates.
The fact that a majority of large asteroids may be rubble piles is not unexpected. Based on light-curve studies that found a near absence of large asteroids rotating faster than the break-up rate for objects lacking tensile strength, Pravec & Harris (2000) concluded that most asteroids larger than a few hundred meters in size are rubble piles.
Nevertheless, our finding appears to have direct relevance to the numerical modeling of main-belt collisional evolution. Thus far, depending on the values assumed for various parameters (including the scaling law for the impact strength), such models have made different predictions as to the existence and fraction of rubble piles at different diameters (Campo Bagatin et al. 2001). We believe that Figure 1 will place specific constraints on those model parameters.
Moreover, Figures 2 and 3 show that, below 300 km in diameter, the bulk porosities of C-group asteroids range from 40% to 70%, while the bulk porosities of S-group asteroids range from 10%to50%. While there is some overlap in these plots, it appears that C-group asteroids tend to have a significantly higher macroporosity than S-group asteroids. We would note that a similar conclusion was reached independently by Consolmagno et al. (2008).
Figure 2. Bulk porosity as a function of effective diameter for the C-group asteroids in Table 3.
Download figure:
Standard image High-resolution imageFigure 3. Bulk porosity as a function of effective diameter for the S-group asteroids in Table 3.
Download figure:
Standard image High-resolution image6. FUTURE PLANS
The availability of high-precision astrometric data, and the rapid discovery of small main-belt asteroids (each a potential test body), should enable many future mass determinations. With new computing technology, however, we can improve upon the methodology described above.
First, the iterative development of an asteroid ephemeris, in parallel with the refinement of asteroid masses, is a cumbersome approach. Ideally, we would undertake a single least-squares solution for the orbits of the ephemeris and test asteroids, and the masses of the subject asteroids. Given the number of observations involved in such a simultaneous solution, this approach would result in a truly enormous covariance matrix. At present, this is not a feasible option; but given the recent rapid advances in storage technology, this approach may be possible in the near future.
Second, the two-body algorithm described in Baer & Chesley (2008), which is used to identify potential mass determination events, is an approximation. In practice, some events forecast to yield significant perturbations have not, and several successful events discovered by other researchers have been missed by this algorithm. With the widespread availability of multi-processor workstations, it may now be feasible to integrate the orbit of a small test asteroid through the period of available observations, both with and without the influence of the large subject asteroid; cases in which the perturbed and unperturbed trajectories result in significant differences in predicted right ascension and declination are obvious candidates for detailed analysis. This method, suggested by Michalak (2000), should be especially useful in identifying suitable encounters for large asteroids like 12 Victoria, whose masses remain unknown.
The authors sincerely thank Dan Britt, Alan Harris, and Carol Neese for collaboration and guidance. The authors are also deeply indebted to the referee for extremely valuable comments. This research was conducted in part at the Jet Propulsion Laboratory, California Institute of Technology, under a contract with the National Aeronautics and Space Administration.
Footnotes
- 4
- 5
- 6