Abstract
The spatial distribution of OH radical density was measured in an atmospheric-pressure dc helium glow plasma produced between a nozzle anode and an electrolyte cathode. Laser-induced fluorescence imaging spectroscopy was applied to measuring OH density. To obtain the accurate distribution of OH density, we carefully examined the spatial distributions of collisional quenching frequency and rotational temperature. The distribution of OH radical density indicates that the surface of the electrolyte cathode works as the sink of OH radicals, suggesting the transport of OH radicals from the gas phase into the inside of the electrolyte.
Export citation and abstract BibTeX RIS
1. Introduction
The development of various methods for producing atmospheric-pressure plasmas has opened a new subject in the field of reactive plasmas. The new subject is the fundamentals of plasma–liquid interaction. The studies on the interactions between plasmas and solid surfaces have a long history, but the plasma–liquid interaction includes processes different from those of the plasma–solid interaction such as evaporation and mist formation. The particle transport in the condensed-matter side in the plasma–liquid interaction is faster than that in the plasma–solid interaction, while the particle transport in the gas phase at atmospheric pressure, which is a necessary condition for studying the plasma–liquid interaction, is slower than that in plasmas at reduced gas pressures, which were conventionally used in studies of the plasma–solid interaction.
The attention focused on the plasma–liquid interaction is driven by its importance in practical applications. An important application of the plasma–liquid interaction is the treatment of liquid media. The decomposition of toxic materials in water,1,2) including sterilization,3,4) is realized by irradiating atmospheric-pressure plasmas. In addition, it is believed that the interaction between plasmas and biological tissues has in common with the plasma–liquid interaction in terms of physics and chemistry, since major parts of biological tissues are electrolyte media. The most radical application of the plasma–liquid interaction is plasma medicine,5,6) which has attracted much attention from many researchers recently.
There are many methods of producing plasmas in contact with liquids.7) One method involves depositing an electrical power into a liquid. The power deposition into liquid produces bubbles in the liquid, and gas-phase plasmas are produced in the bubbles by electrical discharges.8–12) Another method is the use of various kinds of plasma jets.13–16) Since the plasma jet source spouts a plasma into the free space in atmosphere, it is possible to have a plasma jet in contact with a liquid by placing the liquid in the downstream region of the plasma jet. The third way is to use a liquid as an electrode and to produce an electrical discharge between another electrode and the liquid surface.17,18)
In this work, we employed an electrical discharge between a nozzle electrode and an electrolyte to carry out a fundamental investigation on the plasma–liquid interaction. Note that the discharge mode of the plasma used in this study is glow. It has been reported by Shirai and coworkers that a dc glow discharge can be produced in open atmosphere by utilizing a miniature helium flow.19–23) This plasma source is suitable for the fundamental investigation of the plasma–liquid interaction because of its high stability. We focused our interest on OH radicals in the atmospheric-pressure dc glow discharge plasma in contact with the electrolyte. OH radicals have a high oxidation potential and are believed to be important active species in the purification of water, sterilization, and plasma medicine. Recently, the densities of OH radicals in atmospheric-pressure plasmas have been measured by several groups.24–28) In this work, we adopted laser-induced fluorescence (LIF) imaging spectroscopy to measure the spatial distribution of OH radical density. We paid considerable attention to the influences of collisional quenching and rotational temperature to obtain an accurate distribution of OH radical density.
2. Experimental methods
2.1. Plasma source
The plasma source used in this work was identical to that reported by Shirai and coworkers19–23) and is schematically shown in Fig. 1(a). The electrolyte cathode was 1% NaCl solution, which filled a square acrylic vessel with quartz windows. A stainless-steel nozzle anode was placed at a distance of 4 mm from the electrolyte surface. The inner and outer diameters of the nozzle were 0.48 and 0.70 mm, respectively. Helium as a working gas was fed from the nozzle toward the electrolyte surface. The gas flow rate was controlled at 73 sccm using a mass flow controller. The nozzle was connected to a dc power supply via a register of 100 kΩ. A platinum wire immersed in the electrolyte connected the electrolyte to the electrical ground. We report the experimental results obtained at a discharge voltage of 950 V and a discharge current of 40 mA in this paper.
Download figure:
Standard image High-resolution imageFig. 1. Experimental apparatus. (a) Side view showing the details of the plasma source and (b) top view showing the apparatus for LIF imaging spectroscopy.
Download figure:
Standard image High-resolution image2.2. Diagnostics
We adopted LIF imaging spectroscopy to measure the spatial distribution of OH radical density. In the LIF measurement, OH radicals at the electronic ground state are transferred to an excited state by absorbing laser photons at the resonant wavelength with the transition line.29) The LIF intensity, which is obtained by the radiative decay of the excited state, is proportional to the density of ground-state OH radicals. As shown in Fig. 1(b), the light source in this experiment was an optical parametric oscillator (OPO), which generated a tunable pulsed laser beam at a wavelength of approximately 283.0 nm. The shape of the laser beam was arranged to be planar using two cylindrical lenses to illuminate the r–z plane of the cylindrically symmetric discharge space uniformly. The thickness of the planar laser beam was 1 mm, while the width of the planar laser beam was slightly larger than 4 mm. The laser beam excited OH radicals from the ground state [] to an electronic excited state [
]. The laser-induced fluorescence formed an image on the planar laser beam. The fluorescence image was captured using a charge-coupled device camera with a gated image intensifier (ICCD camera) from the angle normal to the incident laser beam. An interference filter, which had a transmission at
nm, was placed in front of the camera to discriminate LIF at the transition bands of
and
from the self-emission of the plasma. The latter LIF was caused by the vibrational energy transfer from laser-excited
to
.24) The LIF images for 40 laser shots were accumulated on the ICCD camera to compensate for the poor shot-to-shot reproducibility of LIF intensity, which was caused by the shot-to-shot fluctuation in the intensity of the OPO laser pulse. In addition, we merged
camera pixels when obtaining the final LIF image to improve the signal-to-noise ratio. Since the ICCD camera had
pixels, the final LIF images were composed of
pixels. The images shown in this paper are composed of
pixels, which were obtained by trimming the signal parts from the recorded images.
In this work, we examined the temporal variation of the LIF image to evaluate the characteristics of collisional quenching. We adjusted the gate width of the ICCD camera at ns. The gate opening of the ICCD camera was synchronized with the oscillation of the OPO laser pulse, and the delay time between the laser oscillation and the gate opening was adjusted using a delay controller. The jitter in the delay time between the laser oscillation and the gate opening was governed by the Q-switch delay time (the delay time between the Q-switch trigger and the laser oscillation) in the YAG laser, which was used for pumping the OPO laser, and was approximately 2 ns in this experiment. LIF intensity has the following waveform after the termination of the excitation:

where and
means the LIF intensity obtained by the excitation from a rotational energy state with a rotational quantum number
. The signal recorded at a pixel of the ICCD camera is given by
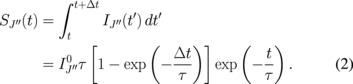
The time constant τ is determined by radiative decay and the collisional quenching of the laser-excited state such that

with A and being the transition probability of the LIF line and the frequency of collisional quenching, respectively. Accordingly, we can examine
by measuring
at various delay times between the laser oscillation and the gate opening. Since the population density of the initial energy state, which is a rotational state of OH
with a rotational quantum number
, is proportional to
, the relative density of the rotational state is obtained by

where

The relationship between the population density of a rotational state and the total OH density at the state is given by

where is the total OH density, Tr is the rotational temperature of the
state, and k is the Boltzmann constant. The energy of the rotational state is given by

where E0 is the energy of the rotational state with ,
cm−1 is the rotational constant of the
state, h is the Planck constant, and c is the speed of light. The rotational temperature Tr can be evaluated using Eqs. (4)–(7) by measuring
for various
numbers.30) We employed five excitation wavelengths corresponding to the Q branch excitations from rotational states with rotational quantum numbers of
, and 10 in this experiment.
Note that is affected by the transition probability of the excitation line and the laser intensity at the excitation wavelength, when the intensity of the OPO laser beam is insufficient for realizing the optical saturation. We compensated for the difference in laser intensity at the excitation wavelengths on the basis of the proportional relationship between the laser and LIF intensities, which was observed experimentally. We ignored the influence of the transition probability on
since the difference between the transition probabilities of the excitation lines was less than 12%.31) The influence of vibrational energy transfer was also negligible under this experimental condition, since we detected the time-integrated LIF intensity using the ICCD camera, and the frequency of collisional quenching was much higher than the transition probability (as will be described below).24)
3. Results
An image of the self-emission of the plasma was captured using the ICCD camera via the interference filter, as shown in Fig. 2. The positions of the nozzle anode and the electrolyte cathode are illustrated in the figure. An intense optical emission was observed in the region of the roughly straight connection between the nozzle anode and the electrolyte cathode. The diameter of the region with the optical emission was roughly the same as the outer diameter of the nozzle anode. The region with the optical emission may correspond to the path of electrical current.
Fig. 2. Distribution of the intensity of optical emission from the plasma, which was observed via an interference filter with transmission at nm.
Download figure:
Standard image High-resolution imageFigure 3 shows the LIF images [ in Eq. (2)] observed at three different delay times between the laser oscillation and the gate opening of the ICCD camera. The rotational quantum number of the initial state in the LIF measurement shown in Fig. 3 was
. The intervals of the delay times among Figs. 3(a)–3(c) were 5 ns. It is understood from the figures that the decay time constant of the LIF intensity was dependent on the position. A shorter decay time constant was observed in the neighboring region to the surface of the electrolyte cathode.
Download figure:
Standard image High-resolution imageDownload figure:
Standard image High-resolution imageFig. 3. Distributions of LIF intensities observed at various delay times of (a) 0, (b) 5, and (c) 10 ns.
Download figure:
Standard image High-resolution imageThe temporal variations of LIF intensity observed at distances of , 1, and 4 mm from the surface of the electrolyte cathode are plotted in Fig. 4. The radial position is on the cylindrical axis (
mm). The horizontal axis of Fig. 4 is the delay time between the laser oscillation and the gate opening of the ICCD camera. Exponential decreases in LIF intensity were observed at
. The decay frequencies ranged from
to
s−1, which were much higher than the decay frequency corresponding to the radiative lifetime of the laser-excited state. This result indicates that collisional quenching was the dominant destruction process of the laser-excited state; therefore, the quenching frequency was evaluated by the exponential fitting of the decay curve (
). The two-dimensional map of the quenching frequency was obtained as shown in Fig. 5 by repeating the curve fitting procedure at each merged pixel of the ICCD camera. Figure 5 clearly indicates a high quenching frequency from the neighboring region to the surface of the electrolyte cathode (
mm), especially in the inside of the path of the electrical current (
mm). On the other hand, a very low quenching frequency was observed in the region below the nozzle anode.
Fig. 4. Temporal variations of the LIF intensities observed on the cylindrical axis of the discharge. The axial observation positions are (a) 0.3, (b) 1, and (c) 4 mm from the surface of the electrolyte cathode.
Download figure:
Standard image High-resolution imageFig. 5. Two-dimensional distribution of the collisional quenching frequency of OH.
Download figure:
Standard image High-resolution imageFigure 6 shows the relationship between and
(the Boltzmann plot) observed at radial positions of
and 1.5 mm. The axial position was at a distance of
mm from the surface of the electrolyte cathode. According to Eqs. (6) and (7), the rotational temperature Tr is evaluated from the exponential fitting of the Boltzmann plot, as indicated in Fig. 6. The same Boltzmann fitting was repeated at each merged pixel of the ICCD camera, and we obtained a two-dimensional map of the rotational temperature, as shown in Fig. 7. The rotational temperatures evaluated in this work include large error bars (
%) because of the data scattering in the Boltzmann plots. However, the map of the rotational temperature shown in Fig. 7 indicates the following meaningful trends. As shown in Fig. 7, a rotational temperature higher than 2000 K was observed near the cylindrical axis of the discharge. The rotational temperature decreased with the radial distance from the cylindrical axis.
Fig. 6. Boltzmann plots for evaluating the rotational temperatures of OH at two radial distances of 0 and 1.5 mm from the cylindrical axis of the discharge. The axial distance from the surface of the electrolyte cathode is 1.2 mm.
Download figure:
Standard image High-resolution imageFig. 7. Two-dimensional distribution of the rotational temperature of OH.
Download figure:
Standard image High-resolution imageBy making use of the knowledge on the spatial distributions of the quenching frequency and the rotational temperature Tr, we obtained the two-dimensional distribution of the total OH radical density (
) at the
state, as shown in Fig. 8. It is known by comparing Fig. 8 with Fig. 3 that an LIF image observed at a certain
number without considering the influences of the collisional quenching and the rotational temperature does not give us the correct spatial distribution of OH radical density.
Fig. 8. Two-dimensional distribution of OH radical density. This distribution was obtained from an LIF image by considering the distributions of quenching frequency (Fig. 5) and rotational temperature (Fig. 7).
Download figure:
Standard image High-resolution image4. Discussion
The spatial distribution of quenching frequency shown in Fig. 5 reflects the spatial distributions of the densities of quencher species. The possible quenchers under this experimental condition are N2, O2 (ambient air), and H2O (the product from the electrolyte cathode). The possibility of the quenching by collision with Na and Cl, which are other possible products from the electrolyte cathode, is negligible, since the distribution of the Na density measured by LIF imaging spectroscopy was different from the distribution of quenching frequency. The distribution of Na density will be reported in a separate paper. The quenching by collision with He is also negligible because of the small rate coefficient.32) The rate coefficient for the collisional quenching by H2O is largest, and is cm3/s, while O2 and N2 have the rate coefficients of
and
cm3/s, respectively.33,34) On the basis of the rate coefficients listed above, the region with a smaller quenching frequency, which was observed below the nozzle anode in Fig. 5, is understood to be due to the jetlike flow of He from the nozzle. The higher quenching frequency observed in the outside region of the He flow is caused by the mixing between He and ambient air.24) On the other hand, the high quenching frequency observed in the region neighboring the surface of the electrolyte cathode can be attributed to the collisional quenching by H2O. The absolute H2O density can be evaluated from the rate coefficient and the quenching frequency observed experimentally. The maximum H2O density thus evaluated is
cm−3, which corresponds to a partial pressure of 11 kPa at a gas temperature of 2000 K. This partial pressure is approximately five times higher than the saturated vapor pressure of water at 20 °C.
The rotational temperature is believed to be an accurate estimation of the gas temperature under the present experimental condition with frequent collisions; hence, the spatial distribution of the rotational temperature of OH (Fig. 7) represents the distribution of gas temperature. The region with a high gas temperature is observed along the current path of the discharge, indicating that the dominant mechanism of gas heating is the recoil of collisions with electrons. The surface of the electrolyte cathode is in contact with the flow of a high-temperature gas at 1000–2500 K, which stimulates the production of H2O from the electrolyte cathode.
A production process of OH is the electron impact dissociation of H2O (), which is active in the region with high-energy electrons. The region with high-energy electrons is roughly represented by the distribution of optical emission intensity shown in Fig. 2. Another possibility is dissociative recombination,
. Since the rate of dissociative recombination is roughly proportional to the square of plasma density, it is also active in the path of the electrical current between the nozzle anode and the electrolyte cathode. Therefore, it is certain that the OH radical density shown in Fig. 8 has broader radial distribution than the production region of OH radicals. That is, the OH radical density shown in Fig. 8 indicates the transport of OH radicals. It is well known that the dominant process of OH loss is the self-association reaction
, which has a two-body rate coefficient of
cm3/s at atmospheric pressure and a temperature of 2000 K.35,36) Considering the flow speed of the gas, a lifetime of ∼0.5 ms is necessary for OH radicals to be transported to the region at a distance of ∼1 mm from the path of the electrical current. According to the rate coefficient and lifetime, the absolute OH radical density is estimated to be on the order of 1015 cm−3.
The final point to be discussed is the axial transport of OH radicals. Figure 9 shows the axial distributions of the OH radical densities observed at three different radial positions. It should be pointed out that the peak position of OH radical density is separated from the surface of the electrolyte cathode. A reason for the low OH radical density in the vicinity of the electrolyte cathode is the less efficient production in the cathode sheath. However, since the thickness of the sheath is less than 1 mm, OH radicals produced in the positive column can be transported across the sheath. The distance between the peak position and the surface of the electrolyte cathode is longer at a longer radial distance from the cylindrical axis, which is due to the fact that the axial flow velocity of the gas is dependent on the radial position. Note that when OH radicals are not reactive with the electrolyte, the decrease in OH density toward the electrolyte surface would not be observed. It should be also pointed out that the OH radical density on the surface of the electrolyte cathode is not zero. The OH radical density on the electrolyte surface would become zero when OH radicals are too reactive in the gas phase, and in this case we cannot expect the interaction of OH radicals with the electrolyte surface. Therefore, the axial distribution of the OH density shown in Fig. 9 indicates that the surface of the electrolyte cathode interacts with OH radicals as their sink. That is, OH radicals are lost on the surface of the electrolyte cathode. A possibility of the loss process is recombination to form H2O2 and H2O. In addition, we can also expect the transport of OH radicals into the inside of the electrolyte.
Fig. 9. Axial distributions of the OH radical density at radial distances of (a) 0.3, (b) 0.75, and (c) 1.5 mm from the cylindrical axis of the discharge.
Download figure:
Standard image High-resolution image5. Conclusions
In this work, we measured the spatial distribution of OH radical density in an atmospheric-pressure dc helium glow plasma in contact with an electrolyte solution by LIF imaging spectroscopy. The LIF image did not give us the distribution of OH radical density, and it was necessary to take into account the spatial distributions of quenching frequency and rotational temperature to obtain the accurate distribution of OH radical density. We discussed the production of H2O from the electrolyte cathode on the basis of the spatial distribution of quenching frequency. The distribution of OH radical density indicates that the surface of the electrolyte cathode works as the sink of OH radicals. This suggests the transport of OH radicals from the gas phase into the inside of the electrolyte.
Acknowledgements
The authors would like to thank Fumiyoshi Tochikubo and Naoki Shirai for the detailed information about the apparatus of the dc glow discharge plasma source.