Abstract
Phonon engineering is expected to contribute to further development of various fields and technologies such as electronics, photonics, thermal engineering, and materials science. Although phonons inherently exist in condensed matter, their behavior strongly depends on the scale of the system and the materials, and they play a major role in electrical, optical, thermal, and mechanical properties. Therefore, researchers have been attempting to find effective ways to control phonons to modify the material properties — in this regard, nanostructuring was found to be highly effective. Here, we review recent advances in the simulations and experiments on phonon transport and thermal conduction control in nanostructures. We mainly focus on tailored nanostructures, especially phononic crystals of which the design is based on the nanoscale phonon transport property.
Export citation and abstract BibTeX RIS
1. Introduction
The physics and control of heat transfer based on the understanding of nanoscale phenomena have attracted considerable attention for the past two decades owing to their application in thermoelectric energy harvesting and thermal management in electronic and optoelectronic devices. The physics and associated heat transfer problems are largely different at different length scales and also depend on the material (Fig. 1). This fact manifests itself in the increasing number of publications related to phonon and thermal transport, and thermoelectrics, as shown in Fig. 2. Most semiconductor devices contain nanostructures with dimensions smaller than the mean free path (MFP) of thermal phonons, which are defined as phonons that mainly contribute to heat transfer. Therefore, the classical model of heat diffusion, known as Fourier law, cannot describe the heat transport in the nanostructures and a partly ballistic description of phonon transport is more suitable for this purpose.1–4) Indeed, strongly reduced values of thermal conductivity have been reported and discussed in terms of the ballistic character of phonons in a variety of nanostructures, such as superlattices,5,6) nanowires,7–13) nanocomposites,14–18) porous films.19–28) Figures 3(a)–3(d) show examples of nanostructures; nanowires, nanodots, a porous membrane, and phononic crystals, all of which are used to reduce the thermal conductivity.
Fig. 1. Examples of materials and devices at each scale.
Download figure:
Standard image High-resolution imageFig. 2. Number of publications in each year searched by keywords in the legend (source: Web of Science).
Download figure:
Standard image High-resolution imageFig. 3. Images of (a) silicon nanowires,9) (b) Ge nanodots in silicon,15) (c) silicon porous membrane,20) and (d) silicon phononic crystal nanostructures.35) Reproduced with permission from Springer Nature for (a) and (b), and from American Chemical Society for (c).
Download figure:
Standard image High-resolution imageThe development of multi-scale phonon simulation effectively couples numerical methods functioning on different length scales. Major advances in the simulation of phonon transport have therefore enabled us to realize the quantitative evaluation of the thermal conductivity of a single crystal and furthermore engineer the thermal transport property of structured material. On the other hand, advances in metrology and microfabrication enabled measurements of the thermal properties at nanoscale. These measurements not only involved the thermal conductivity but also phonon MFP-dependent contributions to thermal conductivity, known as the thermal conductivity accumulation function, in nanostructures and interfaces.3,56) A series of theoretical and experimental contributions demonstrated effective thermal conduction control by tailored nanostructures, which were designed by taking into account the broad thermal phonon MFP spectrum. Heat transfer can be fundamentally understood in terms of phonons — ensembles of lattice vibrations. The phonon density of states, heat capacity, and phonon group velocity can be derived from the phonon dispersion relation. These properties are used to describe heat conduction from the point of view of the wave nature of phonons. However, in practice, to describe heat conduction, phonons are often treated as particles without taking into account their wave properties. In this case, the Boltzmann transport equation and the relaxation time approximation to include different scattering processes are used to obtain the thermal conduction in solids.
Lately, extensive review papers were published on nanoscale thermal transport,2) nanophononics,30) and thermoelectric nanomaterials.31–34) In this review, we focus on phonons and heat transport in tailored nanostructures, which are designed and precisely fabricated to control phonon transport properties, such as phononic crystal nanostructures35) [Fig. 3(d)]. In contrast to the bottom-up fabricated nanostructures shown in Figs. 3(a) and 3(b), phononic crystals are fabricated by a top-down process with systematically controlled structural parameters and can be good test beds to study thermal transport physics at the nanoscale. We highlight recent theoretical and experimental work and explain the underlying physics from both the viewpoints of the particle and wave nature of phonons.
In Sect. 2, we review various simulation techniques and key theoretical predictions on heat conduction in phononic nanostructures. Section 3 is dedicated to recent measurements of the thermal conductivity in nanostructures, particularly in phononic crystals.
2. Modeling of phonon transport and heat conduction in nanostructures
Modeling of heat conduction at the nanoscale is a complex task because of the variety of phonon interactions, wide range of phonon wavelengths, and complicated geometries of nanostructures. An ideal model should take into account the wave nature of phonons and various types of three-phonon, impurity, and boundary scattering events at various temperatures.
Atomistic modeling techniques are the most straightforward way to encapsulate all phonon properties in one model. In such models, the nanostructures are built atom by atom, as shown in Fig. 4, with certain interatomic potentials, such that the lattice vibrations are simulated directly. However, these simulations are computationally demanding and are limited in terms of space and time scales.
Fig. 4. Types of phonon transport simulation techniques.
Download figure:
Standard image High-resolution imageTo simulate realistic structures exceeding tens of nanometers in size, Monte Carlo (MC) techniques are often applied. In the MC models, phonon wave-packets are approximated by particles (bundles of phonons) that travel in straight lines, as shown in Fig. 4. This approach is highly convenient to account for the complicated geometries of the structures and can take into account several phonon scattering processes simultaneously. However, as phonons are represented by particles, the MC models naturally cannot account for phonon interference.
Phonons of which the wavelengths are longer than the atomic scale can be approximated as elastic waves. In this case, the theory of elasticity can describe phonon transport in solids, whereas the finite element method allows the elastic wave equation to be solved in complicated geometries, as shown in Fig. 4. The use of this method enables the modified phonon dispersion relation in complicated geometries to be obtained. This relation allows the changes in the phonon group velocity and the density of states to be computed and makes it possible to consequently study its impact on the thermal conductivity. Although this approach is very convenient to study the effects of phonon interference, fully coherent elastic models cannot account for the incoherent scattering processes that involve a phase change of the phonons.
We now consider these simulation techniques in more detail and discuss the most significant predictions of these simulations.
2.1. From the viewpoint of the particle nature of phonons
By their nature, phonons are lattice waves; however, from an energy transfer viewpoint, they are treated as wave packets. When the characteristic length of the system of interest is much longer than the spatial spread of a phonon wave packet, the phonons can be treated as particles, and then their heat conduction can be described in terms of kinetic theory. In phonon kinetics, the thermal conductivity (κ) of an isotropic system is given by36)

where Cm, vm, and τm are the heat capacity, group velocity, and relaxation time of the phonon, respectively, with mode m characterized by wavevector q and phonon branch j. The term Ω denotes the volume of the system and Λm represents the MFP, which is expressed by Λm = vmτm with vm as the group velocity and τm as the relaxation time. In this section, we review approaches to evaluate and engineer phonon heat conduction by tailored nanostructuring from the viewpoint of the particle nature of phonons.
As mentioned above, the development of nanotechnology enables us to fabricate nanostructured bulk materials such as nanoparticle sintered and nano-inclusion embedded materials.37,38) This class of nanostructures has mostly been studied for thermoelectrics with the aim to reduce the thermal conductivity.
Whereas the electrons and holes contributing to the electrical conductivity are basically limited to the Fermi surface, the frequency and MFP of thermal phonons are broad, indicating that single-length-scale structuring has limited effect on the overall phonon transport. Consequently, the combination of several structuring techniques with different length scales, namely hierarchical structuring, is effective in the manipulation of thermal conductivity.39) Obtaining an understanding of hierarchical structuring requires the development of insight into the microscopic mechanism of phonon transport and thermal conductivity spectrum. Therefore, a quantitative evaluation of the thermal conductivity spectrum on frequency and/or MFP space is demanded.
Quantitative heat conduction analysis requires accurate modeling of the vibrational modes in a crystal; thus, it is necessary to obtain the interatomic force constants (IFCs) that describe the interactions among atoms. The IFCs are defined as the Taylor expansion coefficients of potential energy (V) with respect to the atomic displacement (u)

Here, the integers i, j, and k denote atomic indices; α, β, and γ are Cartesian coordinates; V0 is the equilibrium potential energy; Φ and Ψ are harmonic and third-order anharmonic IFCs, respectively. For the calculation of harmonic IFCs, ab initio methods such as the frozen phonon method with a force–displacement dataset from the first principles40) and density function perturbation theory41) have been well established. As for third-order cubic IFCs, numerical methods combined with first principles have now become accessible.42–45)
Once harmonic IFCs are obtained, the phonon dispersion relation can be readily calculated by solving a dynamical matrix with the given wavevector in the framework of lattice dynamics. The harmonic phonon properties, heat capacity, and group velocity are easily obtained from the calculated phonon dispersion relation. By contrast, the relaxation time is caused by intrinsic lattice anharmonicity and external effects such as impurities and boundaries. On the basis of perturbation theory to third-order anharmonic potential, the relaxation time for three-phonon scattering, which is the most dominant in intrinsic thermal resistance, is given by36,40,46)
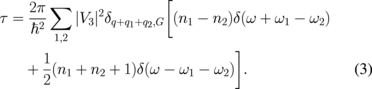
Here, V3 is a measure of intrinsic three-phonon scattering and is composed of third-order cubic IFC, eigenvector, and phonon frequency. Equation (3) is based on the single-mode relaxation time approximation, which assumes that two other phonons involved in the scattering are in equilibrium. Finally, the thermal conductivity can be evaluated through Eq. (1). This framework is known as anharmonic lattice dynamics (ALD), and has been demonstrated to be an effective method for the quantitative evaluation of heat conduction in materials (silicon,44,45) half-Heusler compound,47) lead chalcogenides,48,49) magnesium silicide.50)) More recently, the framework has also been successfully applied to materials with complex structures such as skutterudite,51) clathrate,52,53) and strontium titanate.54,55)
The thermal conductivity spectrum and its accumulation function are the key quantities for manipulating thermal conductivity of material by structural control. The accumulation function of the thermal conductivity with respect to the MFP proposed by Dames and Chen56) is given by

Here we assume an isotropic material. Λ0 denotes the cut-off MFP. An example of the thermal conductivity accumulation function is shown in Fig. 5(a). When the system of interest is quite large such that MFP distribution is continuous, it is convenient to express Eq. (4) in its integral form.57)

The integrant in this equation is the thermal conductivity spectrum. Since the equations indicate that summation or integration accounts for the contributions of phonons with an MFP shorter than Λ0 to the overall heat conduction, κC naturally becomes zero for Λ0 = 0 and reaches the bulk thermal conductivity for Λ0 = ∞.
Fig. 5. (a) Simulated thermal conductivity accumulation function and (b) thermal conductivity as a function of hole diameter in simulated and measured phononic crystals.35)
Download figure:
Standard image High-resolution imageThe ALD is a powerful tool for the quantitative analysis of heat conduction; however, this method is based on reciprocal space and is not applicable to not only combination but also each structure of alloying, interface, and nanostructure, where real-space information, such as spatial and size distributions, becomes important. As for alloying, it is possible to take into account the mass-difference as an alloy effect in the ALD framework by perturbation theory.58) By contrast, the force-field difference is commonly included phenomenologically and explicit treatment for the effect based on perturbation theory has not been available.59) Furthermore, even for the mass-difference effect, the validity of the perturbative approach is limited to a small mass contrast and low concentration.60,61) In the case of large mass contrast and impurity concentration, one should employ an alternative approach such as the T-matrix method beyond the Born approximation.62,63)
In this sense, a real-space approach with the ability to directly incorporate the effects of alloying, interface, spatial distribution, and the complex geometrical shape of nanostructures is needed. Molecular dynamics (MD), which is a representative real-space method, is advantageous in that it can take those effects into account. When performing an MD simulation, the potential function to be used should reasonably reproduce the thermophysical properties of the material. In this regard, an MD simulation with first-principles-based IFCs is noticeably useful. Thus, Murakami et al.64) developed a local virtual crystal scheme for alloy systems and calculated the thermal conductivity of lead telluride and lead selenide alloys by performing IFCs-MD simulations. Their calculated thermal conductivities are in good agreement with the experimental values, and the importance of incorporating the local effects of force-field change is verified. Apart from the IFCs-MD simulation for alloy systems, IFCs-MD enables us to obtain insight into phenomena arising from the large lattice anharmonicity of lead telluride, for instance, the local lattice distortion and presence of an anharmonic mode as observed by neutron scattering experiments.65) In this way, IFCs-MD becomes significant in the investigation of thermophysical properties and thermal conductivity. However, the typical length scale of the simulation domain in MD simulation is of a magnitude of the order of micrometers, thus the applicability of MD simulation to the heat conduction calculation of an actual nanostructured bulk material is limited.
When investigating heat conduction in such a mesoscopic system, phonon MC simulation with harmonic and anharmonic properties obtained from ALD is effective.66) This method simply solves the phonon Boltzmann transport equation with a relaxation time approximation in real space.
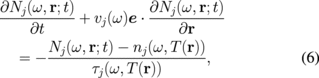
where denotes a non-equilibrium distribution function of a phonon with frequency ω and branch j at a position r and nj is an equilibrium distribution, i.e., the Bose–Einstein distribution function. The unit vector e points in the phonon propagation direction. The phonon MC simulation has been successfully applied to nanostructured materials.35,67,68) For instance, Hori et al. performed the simulation for thermoelectric lead telluride with nanovoid as best case scenario, and evaluated the impacts of the size and volume fraction of nano-inclusion on the reduction of the thermal conductivity in terms of the thermal conductivity spectrum.69) Figure 5(a) shows an example of the thermal conductivity accumulation function obtained for phononic crystals with holes of different diameters using the MC simulations.35) Figure 5(b) shows that this simulations are in agreement with the experiment.35)
Another approach for analyzing the heat conduction on a mesoscopic system is the phonon MC ray-tracing (RTMC) method, which shoots a phonon into a system with complex internal structures and calculates the transmission probability. The drawback of this method is that RTMC simulation does not treat the mode conversion due to phonon–phonon scattering and incorporate the phonon branch effect. However, this method enables us to study the influence of a complicated internal structure on the thermal conductivity. Hori et al.70) performed an RTMC simulation for a polycrystalline nanostructure and systematically investigated the distribution of nanoparticle size on the heat conduction. In the phonon MC and RTMC schemes, phonon transmittance at the interface is needed. Phonon transmittance has been often evaluated by acoustic mismatch and/or diffusive mismatch empirical models. However, MD-based methods71–74) or the methods based on Green's function75,76) have become available and allowed obtaining mode-dependent phonon transmittance without any fitting parameters.
As described above, the heat conduction analysis for an actual nanostructured material requires us to combine different schemes that are effective at specific length scales and perform multi-scale simulation.
2.2. From the viewpoint of the wave nature of phonons
As phonons are waves of the atomic lattice, they are naturally able to experience interference, which can affect heat conduction. Indeed, in periodic nanostructures, phonons reflect from the periodic boundaries and interfere in a way similar to that of the photons in photonic crystals. Such interference may cause phononic bandgaps — frequency ranges in which phonons cannot exist.77) However, these bandgaps usually cover only narrow frequency ranges and cannot significantly affect heat conduction.24) Nevertheless, the interference also flattens branches of phonon dispersion in a much wider frequency range than the overall bandgaps.24,78) Naturally, this flattening of the phonon dispersion results in the reduction of the group velocity and affects heat conduction. For example, simulations79–81) and experiments5,6,82) showed that the phonon interference can play a significant, if not dominant, role in heat conduction in superlattices. However, because most practical applications rely on planar silicon-on-insulator technology, a two-dimensional in-plane periodicity is more convenient than cross-plane periodicity of superlattices.83) Thus, in this section, we consider the wave effects that take place in periodic two-dimensional membranes known as phononic crystals, as acoustic analogues of photonic crystals.
Most two-dimensional phononic crystals consist of arrays of either holes in a membrane (hole-based) or pillars on the membrane (pillar-based). In hole-based phononic crystals, the periodicity of holes results in phonon interference, which flattens the branches of phonon dispersion,24,78) as shown in Fig. 6(a) for the first two branches. In pillar-based phononic crystals, in addition to the interference caused by the periodicity, the phonons experience local resonances in the pillars. Figure 6(a) shows that the modes that are physically located inside the pillar (dark color), and thus correspond to the local resonances, are flatter than those that are located in the membrane (green color).84)
Download figure:
Standard image High-resolution imageFig. 6. (a) Dispersion of a membrane (dashed-dotted lines), eigenfrequencies of the single pillar (dashed lines), and dispersion of a pillar-based phononic crystal (solid lines). The color represents the physical location of the mode on the vertical axis. (b) Schematics and relative thermal conductance of hole- and pillar-based phononic crystals as a function of the period at the temperature of 0.5 K. The membrane and pillar thickness is 80 nm and the radius-to-period ratio is kept constant at 0.4.78,84) Reprinted with permission from Ref. 84. © (2018) American Physical Society.
Download figure:
Standard image High-resolution imageTheoretical studies24,78,84–86) of heat conduction in phononic crystals in the fully coherent regime showed several counterintuitive phenomena. Figure 6(b) shows that phononic crystals generally suppress heat conduction, as compared to the unpatterned membrane. However, at very low temperatures, phononic crystals with short periods can actually become more conductive than unpatterned membranes,85) despite the removal of the material. This increase in thermal conductance is caused by the increase in the phonon density of states due to the flattening of the first few bands.78,85,86) Moreover, Fig. 6(b) shows that unlike in the diffusive heat conduction regime, the stronger suppression of thermal conductance is found in phononic crystals of a longer period,24,78,84,86) despite the lower surface-to-volume ratio which is usually proportional to the suppression of heat conduction.87)
In general, simulations showed that both hole- and pillar-based phononic crystals behave alike, yet local resonances introduce a certain difference.84) On the one hand, as the local resonances cause dispersion flattening, some authors assume that this causes the suppression of heat conduction, as observed by atomistic simulations.88–91) For example, by evaluating the thermal conductivity of a pillar-based phononic crystal using lattice dynamics, Davis and Hussein88) found a reduction in thermal conductivity of approximately 50% and attributed it to the local resonance and hybridization. Subsequent studies also found similar impacts on the thermal conductivity reduction.90–92) On the other hand, other authors84) argued that flattened bands increase the phonon density of states, which can only contribute to the enhancement of heat conduction, thus the local resonances only limit the reduction in thermal conductance caused by structural periodicity, hence the saturation of thermal conductance in pillar-based phononic crystals shown in Fig. 6(b). However, even in simulations it is difficult to separate the impact of the local resonances from the impact of the periodicity; thus, debates about the impact of the local resonance on the heat conduction are ongoing.
We previously mentioned that, when the spatial spread of a propagating phonon wave packet [Fig. 7(a)] is much shorter than the characteristic length scale in a system, the phonon can be treated as a particle. Therefore, if the length scale of a nanostructure [such as an alloyed thin-layer, membrane with a pillar and an embedded nanoparticle, as illustrated in Fig. 7(b)] becomes shorter than the spatial spread of the wave packet, it is possible to manipulate the phonon transport by exploiting the effects of interference and resonance. Namely, this approach locally uses the wave nature of phonons for controlling phonon transport, which is conceptually different from interference due to the periodicity of phononic crystals.
Fig. 7. Schematics for (a) phonon wave-packet (b) phonon interference at nanostructured interface (two-path destructive interference) (c) local resonance and hybridization of phonons modes by substructure (d) local resonance by embedded nanoparticle. In (c), m and M are the atomic masses of the host lattice and substructure and Φ, Φint, and Φ' denote the spring constants of atoms for the host lattice, interface between the host lattice, and substructure, respectively.
Download figure:
Standard image High-resolution imageLet us first consider destructive phonon interference in a one-dimensional lattice with an impurity atom [Fig. 7(b)]. In the lattice, an incident phonon propagating from the left semi-infinite region is split into two phonons because of the existence of two pathways. Because the introduction of impurity mass yields different impedance for different pathways, a phase difference between two phonons appears and suppresses the propagation of superimposed phonons due to interference.93) This effect can be readily applied to a three-dimensional lattice. Han et al.94) conducted a phonon-wave-packet simulation for thin layers of alloys embedded in a Van der Waals crystal and found that the effect significantly inhibits low-frequency phonons contributing to thermal conductivity. Furthermore, by adding two thin layers of alloys to a system, they succeeded in confining a specific phonon, which resembles a phonon capacitor.95)
A typical example of phonon transport utilizing resonance is a structure with a pillar. We consider a one-dimensional lattice with a sidebranch as illustrated in Fig. 7(c).93,96) The dynamical equation for an atom connected to the sidebranch is given by −mω2u0 = Φ(u1 − 2u0 + u−1) + Φint(U1 − u0). Here m and Φ are the atomic mass and spring constant of the host lattice, Φint is the spring constant at the interface, and u and U are the atomic displacements of the host lattice and sidebranch, respectively. When the energy of the propagating phonon is identical to the discretized energy levels of the sidebranch, the propagating phonon is absorbed by the sidebranch and not allowed to pass. Using this concept, Xiong et al.92) studied heat conduction in silicon and silicon/germanium alloyed nanowires with pillars and obtained thermal conductivity comparable to the amorphous limit (∼1 W m−1 K−1) by reducing the contributions of low-frequency and high-frequency phonons due to the phononic effect and alloy scattering, respectively.
In terms of interference and resonance, the presence of constructive interference and strong selectivity of the resonant phonon, respectively, can be problematic. With respect to interference, the presence of constructive interference sometimes allows perfect transmission of a phonon, which can be seen in the Fabry–Pérot interference for multi-layered structures. However, since constructive and destructive interference events are not yielded equally, one may enhance the impact of destructive interference by designing the structure appropriately. In fact, Ju et al.97) recently applied materials informatics by applying an approach combining Green's function and Bayesian optimization to interfacial phonon transport. As a result, they identified that a tailored aperiodic superlattice has maximum thermal boundary resistance by maximizing the overall impact of destructive interference. As for resonance, selectivity in resonance does not affect the transport of non-resonate phonons. According to the study on phonon resonance with a nanoparticle embedded in a matrix [Fig. 7(d)],98) the resonant frequency of a nanoparticle with a diameter of a few nanometers is of the order of 0.1–1 THz, which is useful for suppressing lower-end-frequency phonon transport. However, the impact of phonon-nanoparticle resonance is quite limited for reducing the overall thermal conductivity.
The most critical issue on utilizing the wave nature of phonons for transport is phonon coherence. Since the coherence of a phonon is sensitive to lattice anharmonicity and roughness, the coherence length correspondingly changes noticeably depending on the internal and external conditions. The effects of phonon interference and resonance would be suppressed when the coherence length is short because a short length does not allow the spatial length or sufficient time for interference and resonance. In this context, the robustness of interference and resonance should be carefully assessed.95,98,99) Thus far, phonon coherence loss or coherent-incoherent phonon transport have not been directly addressed. Latour et al.79,100) attempted to directly estimate the coherence length of a thermal phonon in Si/Ge (heavy Si) superlattice and graphene by calculating the mutual correlation function of atomic velocity and wavenumber linewidth of spectral energy density. As a result, they found that similar to a phonon MFP, the coherence length of a phonon is strongly dependent on frequency and is widely distributed, ranging from a few angstroms to several tens of nanometers. Although knowledge on phonon coherence remains limited, progress on understanding the microscopic mechanism of coherence loss would be developed by a combination of numerical calculation and measurement, leading us to material design with the desired heat conduction property.
3. Experiments on phonon transport and heat conduction in nanostructures
Advances in nanofabrication enabled experimental studies on heat conduction in nanostructures. In the past decade, researchers measured the thermal properties of semiconductor nanowires, ultra-thin membranes, and various other nanostructures. In this section, we summarize recent experimental results on heat conduction in periodic nanostructures.
3.1. From the viewpoint of the particle nature of phonons
In semiconductor nanostructures, phonons frequently encounter the boundaries of the structure, and surface scattering processes strongly affect heat conduction. When the wavelengths of phonons are shorter than the surface roughness, the surface causes the phonons to scatter diffusely, thereby hindering heat conduction in the nanostructures. In this case, the thermal conductivity becomes inversely proportional to the surface-to-volume ratio of the structure, as shown by experiments87,90,101) and simulations.101–103) On the other hand, diffuse surface scattering limits phonon MFP, thus limiting the thermal conductivity,12,35,68) as also shown in Fig. 4(a). From this point of view, phonon MFP, and thus the thermal conductivity, becomes approximately proportional to the limiting dimension of the structure.35,101)
To illustrate this point of view, let us compare the reported experimental values of effective thermal conductivity measured at room temperature in two-dimensional phononic crystals. We compare the data from Kim et al.,104) Anufriev et al.,27,87) Nakagawa et al.,105) Tang et al.,20) Yu et al.,22) Lee et al.,106) Wagner et al.,25) and Lim et al.107) Note that the effective thermal conductivity of a porous material (κeff) is related to the thermal conductivity of the material between the pores (κ) as κeff = κ × F(φ), where F(φ) is the volume reduction factor given by (1 − φ)/(1 + φ/2) with φ as the porosity. Here, for convenience, we converted the data from Refs. 27, 87, and 105 into the effective thermal conductivity. For clarity, we mainly plot the data on a square lattice of holes.
Figure 8 shows all the effective thermal conductivity values of two-dimensional silicon phononic crystals as a function of neck (n) — the distance between two neighboring holes. The literature data seem to form a common trend. Yu et al.22) and Lim et al.107) measured extremely small phononic crystals fabricated using block copolymer lithography and their structures display little thermal conductivity. The trend seems to be continued by the data obtained by Anufriev et al.27,87) who measured phononic crystals with periods in the 120–500 nm range. Finally, the data from Kim et al.,104) who generally measured larger phononic crystals, behaved similarly.
Fig. 8. Experimental values of effective thermal conductivity at room temperature as a function of neck size.
Download figure:
Standard image High-resolution imageHowever, the data from Tang et al.20) and Wagner et al.25) are lower than the other data in terms of their thermal conductivity, which is most probably due to the very high surface roughness (≥8 nm) of their samples.
Remarkably, the data in the literature seem to follow this common trend despite the very different periods (a) and thickness (h) of the structures. Thus, the neck, rather than any other parameter, seems to be the limiting dimension determining the thermal conductivity. Indeed, Anufriev et al.87) showed that, when the neck becomes smaller than 100 nm, the thermal conductivity is completely determined by the neck, regardless of even the density of holes.
Moreover, as the neck becomes smaller an interesting phenomenon of phonon directionality emerges. Recent experiments27) showed that heat propagates faster in phononic crystals with a square lattice than in those with a staggered lattice because phonons can travel ballistically in direct passages between holes of the aligned lattice, whereas in the staggered lattice the direct passage is blocked. MC simulations27) showed that the aligned lattice of holes creates directional heat fluxes, which can be used to guide and even focus heat fluxes in a spot, as shown in Fig. 9. Remarkably, unlike the effects of phonon interference described in the next section, these ballistic directionality effects have been observed experimentally12) and in simulations,101,108) even at room temperature.
Fig. 9. MC simulations27) show ballistic heat guiding (a) and heat focusing (b) in phononic nanostructures.
Download figure:
Standard image High-resolution image3.2. From the viewpoint of the wave nature of phonons
In periodic nanostructures, phonons that reflect from periodic boundaries can experience interference, which can affect heat conduction, as discussed in Sect. 2.2. To develop the interference patterns, phonons need to preserve their phase when undergoing surface scattering. The phase preservation is possible only if the mean surface roughness is smaller than the phonon wavelength. At room temperature, at which the phonon wavelengths are rather short (0.5–5 nm in silicon), this condition can be satisfied only for atomically smooth surfaces, which can be found, for example, in epitaxial superlattices.
Indeed, in superlattices coherent heat conduction has been experimentally observed even at room temperature.5,6,82) Figure 10 shows that the thermal conductivity of a (STO)30/(BTO)1 superlattice shows nonlinear dependence on the thickness of the period and the nonlinearity becomes stronger as the temperature is decreased.5) The dip of the curve indicates the cross-over between coherent and incoherent heat conduction regimes. Indeed, in the case of long periods, phonons do not "sense" the interference, thus the heat transport is diffusive and the thermal conductivity is simply proportional to the number of scattering interfaces. However, when the periods are sufficiently short, phonon interference reverses the trend and the thermal conductivity becomes inversely proportional to the period thickness. A similar dependence on the period has already been discussed in Fig. 6 for phononic crystals. However, one-dimensional superlattices can be used only for a limited number of applications, and most applications require two-dimensional periodicity.83) Thus, we subsequently provide an overview of advances in coherent heat conduction control in two-dimensional periodic nanostructures. Sub-Kelvin experiments on microscopic SiN phononic crystals (a ∼ 1, 2.5, and 4 µm) show that below 0.5 K heat conduction is fully ballistic and is controlled only by coherent modifications of phonon dispersion.24,109) From Maasilta et al.109) we estimated the coherence of heat conduction by comparing the experimental results with theoretical predictions in a fully coherent regime, and plotted several data points for clarity. The data show that the coherence is quickly lost above 0.5 K.
Download figure:
Standard image High-resolution imageFig. 10. (a) TEM image of the (STO)30/(BTO)1 superlattice and (b) thermal conductivity as a function of density of the interfaces in the superlattice.5) Reprinted with permission from Ref. 5. © 2014 Springer Nature.
Download figure:
Standard image High-resolution imageMaire et al.26) studied ordered and disordered silicon phononic crystals with a period shortened by one order (a = 300 nm) and demonstrated that heat conduction can remain partly coherent at 4 K. They showed that the impact of phonon coherence depends on the periodicity of holes and weakens as the temperature is increased. Researchers determined the working temperature range and surface roughness conditions by studying phonon transport at different frequencies25) and temperatures.26) They developed a model based on the phenomenological fact that phonon coherence is lost when the phonon wavelength is ten times shorter than the mean surface roughness. Figure 11 shows predictions of this model26) for different values of the mean surface roughness (σ). Although the experimental data are scattered because of the different scales and quality of measured samples, the model seems to capture the general trend. This general trend shows that phonon coherence completely disappears at temperatures above a few tens of Kelvin. Indeed, experiments25,26,106) at room temperature could not find any sign of coherent heat conduction. Recently, Lee et al.106) demonstrated no difference in heat conduction in ordered and disordered phononic crystals, even for a period as small as 100 nm. However, below 100 K, their data seem to start showing some difference, although the data points are widely scattered.
Fig. 11. Coherence of heat conduction in phononic crystals reported25,26,106,109) as a function of temperature (dots). Lines are predictions of simulation model26) for different values of the mean surface roughness (σ).
Download figure:
Standard image High-resolution imageEven though hole-based phononic crystals became the subject of many experimental studies, until recently, the heat conduction in pillar-based phononic crystals was only studied theoretically. On the one hand, Brillouin light scattering experiments110–112) demonstrated that pillars can indeed flatten phonon dispersion in the low-frequency spectrum range and form both Bragg and locally resonant bandgaps. On the other hand, it remains unclear whether these low-frequency changes are sufficient to affect heat conduction at room temperature. Recently, Iskandar et al.113) created pillar arrays on the surface of silicon using reactive ion etching and demonstrated that this surface treatment changes the heat capacity of silicon. However, since different samples showed a decrease as well as an increase in the heat capacity, more experimental data are needed to conclude whether these changes are caused by local resonances. Conversely, Anufriev et al.114) showed that aluminum pillars deposited on silicon nanobeams significantly reduce the thermal conductivity of the nanobeams. However, they discovered that, rather than originating from the local resonances in the pillars, they simply have their origins in the interface roughness created by the metal deposition. Thus, the experimental demonstration of the thermal conductivity by local resonances in pillars remains a challenging matter.
4. Conclusions and outlook
We reviewed heat conduction simulation techniques based on both the particle and wave nature of phonons, and highlighted the most notable theoretical predictions and experimental results obtained on various nanostructures and especially on phononic crystals.
In addition to the accurate calculation of the thermal conductivity, a remarkable finding obtained by first-principles-based heat conduction analysis is that phonons in a wide range of frequencies and/or MFP contribute to heat conduction. This multi-scale character of phonon transport often becomes problematic in the manipulation of thermal conductivity. Yet, progress on multi-scale phonon simulation coupling has led to the development of several methods that are effective on different length scales and provide several pathways for engineering the thermal transport property of materials. However, several problems, in particular for coherent phonons, remain. Although a phononic crystal is widely believed to potentially have intrinsically small thermal conductivity, the mechanism of coherence loss in a phononic crystal has not been elucidated. In order to resolve this mechanism and realize low-coherence loss, further studies relevant to the physics of phonon coherence are required.
As far as experimental work is concerned, the main results can be divided into those showing incoherent and those showing coherent control of the heat condition. In the case of incoherent heat conduction, we showed that the data in the literature indicate that the thermal conductivity of nanostructures is mainly controlled by the narrowest dimension of the structure. The narrow regions naturally scatter phonons and thus limit the phonon MFP and the thermal conductivity.
As for the coherent control of heat conduction, whereas superlattices can coherently control heat conduction even at room temperature, two-dimensional hole-based phononic crystals can support coherent heat conduction only at low temperatures. To expand the working temperature range, future studies should search for techniques to reduce surface roughness and study materials with longer phonon wavelength, such as alloys.83) Pillar-based phononic crystals require sound proof-of-concept experiments to demonstrate the potential predicted in theoretical work. In the near future, thermal conduction control by using tailored nanostructures taking into account both coherent and incoherent control can be used to control the heat conduction more efficiently. Moreover, a functional thermal device may be demonstrated based on the ballistic properties and wave nature of phonons.
Acknowledgments
The authors would like to thank Professor K. Hirakawa, Professor S. Volz, S. Gluchko, R. Yanagisawa, Dr. L. Feng, Dr. S. Ju, and Professor T. Hori of the University of Tokyo for discussions.
Biographies
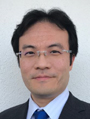
Masahiro Nomura is Associate Professor in Institute of Industrial Science, The University of Tokyo. He received his bachelor, master, and Ph.D. degrees in Applied Physics in 2000, 2002, and 2005, respectively, from The University of Tokyo. His research interests include hybrid quantum science, phonon/heat transport in semiconductor nanostructures, and energy harvesting by thermoelectrics. The concept of his current research is "from photonics to phononics" using phononic crystals, which have some physical analogy with photonic crystals. He is a recipient of The Young Scientists' Prize by the Minister of Education, Culture, Sports, Science and Technology (2012), ISCS Young Scientist Award (2017), German Innovation Award — Gottfried Wagener Prize (2018), and 10 other awards.
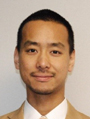
Junichiro Shiomi is Professor in Department of Mechanical Engineering, School of Engineering, the University of Tokyo. He received B.E. (1999) from Tohoku University, and Ph.D. (2004) from Royal Institute of Technology (KTH), Sweden. Leading the Thermal Energy Engineering Lab, he has been pursuing research to advance thermal management, waste heat recovery, and energy harvesting technologies based on nano-to-macro innovation in materials, structures, and systems. He is Fellow of Japan Society of Mechanical Engineers and serves as associate editors of Applied Physics Express, Japanese Journal of Applied Physics, and Transactions of the Japan Society of Mechanical Engineers. He is a recipient of the Zeldovich Medal from the Committee on Space Research, the Young Scientists' Prize, the Commendation for Science and Technology by the Minister of Educational, Culture, Sports, Science and Technology, and the Academic award of Heat Transfer Society of Japan.
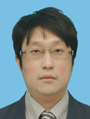
Takuma Shiga is Assistant Professor of the department of Mechanical Engineering, The University Tokyo. He received his Ph.D. in Engineering in 2013 from the University of Tokyo. His research focuses on heat conduction in materials from first principles and coherence control of phonon in phononic crystals.
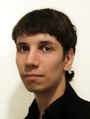
Roman Anufriev is a project research associate of Institute of Industrial Science of the University of Tokyo. He received his Ph.D. in 2013 from the INSA-Lyon in France. His research focuses on the heat conduction control in silicon nanostructures and phonon transport engineering using phononic crystals and metamaterials.