Abstract
The narrow bandwidth and low amplification performance of a subwavelength acoustic resonator greatly limit its applications. In this study, broadband acoustic amplification using an array of two-dimensional quarter-wavelength resonators is investigated. A new acoustic resonator unit is designed, which contains one open end with an impedance-gradient meta-structure and another closed end to confine the acoustic transmission and achieve sound amplification. The sound pressure level (SPL) gain and bandwidth of the resonator can be controlled by changing its geometric parameters. In an energy-harvesting experiment, the broadband amplification resonator provides a 17.2-dB SPL gain and enhanced output voltages.
Export citation and abstract BibTeX RIS
The acoustic resonator cavity is of great interest for scientists and engineers because of its ability to collect, confine, and amplify sound waves.1–4) Various resonator cavities have been investigated, including the half-wavelength chamber resonator, Helmholtz resonator, and sonic crystal. Acoustic resonator cavities are widely used in many applications, such as acoustic amplification,2,5,6) redirecting sound waves,7–9) high-resolution acoustic imaging,10–12) and sound absorption for acoustic dampers or mufflers.13,14) By modifying the localized resonance structure, specific sound waves can be directly amplified and then utilized for energy harvesting and signal detection.15) Among the different types of resonators, the one employing coiled-up space as a sound wave guide is of vital importance because of its straightforward design,16–20) where the incident acoustic wave is controlled into narrow coiled-up channels, resulting in unusual effective parameters. Using this periodical subwavelength structure design, prosperous applications, including acoustic amplification resonators, have been realized.2,6,21–24)
Although the two-dimensional (2D) coiled-up design has been successfully applied for sound amplification and energy harvesting,2,22) the relatively low acoustic amplification and narrow bandwidth are two major impediments. These issues are largely due to the discontinuous acoustic impedance between different mediums: the impedance mismatch between the external environment (air) and the meta-structure leads to worse transmission when the working frequency is off-resonance.25) One of the most common ways to solve this problem is to add impedance-matched structures. This approach has been used in many studies to improve the broadband performance. When a gradient helical structure is attached to traditional three-dimensional (3D) metamaterials, the whole design shows high sound energy transmission over a significantly wider bandwidth.26–28) For a 2D labyrinthine unit, a tapered meta-structure with gradually changing cross sections can effectively mitigate the impedance mismatch at the interface between the air and the meta-structure.29,30) However, none of these impedance-matched designs has been used in an acoustic amplification resonator to realize broadband amplification performance.
In this paper, a subwavelength resonator with one impedance-gradient meta-structure slab that can confine and amplify the incident sound wave over a broad band is presented. Gradually varying the slit width contributes to the gradient effective density of the metamaterial, leading to the gradient distribution of the effective impedance from the inlet of a coiled-up structure to the outlet. Using the effective medium method and corresponding simulation results, the effective parameters of different designs are calculated. The acoustic amplification ratio and broadband performance of the 3D-printed quarter-wavelength resonator are measured according to the sound pressure level (SPL) gain, and the simulations agree with the experimental results. An electricity-generation experiment based on the impedance-matched resonator demonstrates its feasibility for acoustic energy harvesting.
The acoustic meta-structure resonator is based on a modification of the normal path-coiling structure with an additional gradient meta-structure, as shown in Fig. 1(a). The subwavelength impedance-matched structure can be described by several geometric parameters, including the flange width w, the length of the impedance-matched slab p, the path length in the transverse direction l, and the gradually variable slit width sn, as shown in Fig. 1(b). The acoustic waves are forced to propagate along the path filled with air, and the other parts are filled with a polymer (made by 3D printing), which has high acoustic impedance to prevent direct sound transmission. In the air gap region, the acoustic waves are continuously confined and reflected, resulting in high sound pressure amplification inside the cavity. To further investigate the impedance-matched structure, the effective medium method can be employed to calculate the effective parameters, including the effective refractive index and effective impedance. The ratio between the length of the wave propagation path in the metamaterial and the distance along the propagation direction can be estimated as the effective refractive index neff, which can be expressed as17,24)

where dl is the differential change of the wave propagation length, dz is the differential change of the projection distance in the wave propagation direction, c0 is the speed of sound in the background environment, and ceff is the effective sound speed. The effective density can be calculated using the minimum transmittance for which the acoustic wave passes through the effective medium.26,27,31) The minimum transmittance can be expressed as
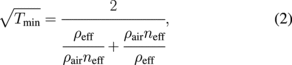
where ρair is the density of air, and ρeff is the effective density of the meta-structure. Consequently, the effective impedance Zeff can be calculated as

according to the definition of acoustic impedance.
Fig. 1. (a) Configuration of the acoustic meta-structure amplification resonator; (b) design of the 2D resonators with different IM structures; (c) 3D-printed meta-structure amplification resonators with different structural parameters. The scale bar represents 30 mm.
Download figure:
Standard image High-resolution imageThe acoustic amplification resonator has dimensions of 7 × 7.1 × 10 cm3 and includes seven identical meta-structure resonator units. Three different designs of the impedance-matched layers attached to the normal coiled-up structures are investigated [Fig. 2(a)]. They show the same traditional coiled-up structures with a flange width of w = 7 mm, a path length in the transverse direction of l = 8 mm, a slab length of k = 9 mm, and a fixed slit width of 1 mm. For the impedance-matched structure, the three designs exhibit the same slab length p = 42 mm, with a gradually varying slit width sn, which is defined as follows:

where n is the sequence number of the slit in the transverse direction, and a is the increasing value of each slit width. In Eq. (4), because the gradient slit widths deviate from the fixed slit width of 1 mm, 1 mm should be added to each slit width. Using Eqs. (1)–(3), the effective refractive index and effective impedance can be calculated for special designs of the impedance-matched layer. The gradient distributions of the effective refractive index neff and the effective impedance Zeff along the x-direction (sound wave propagation direction) are shown in Figs. 2(b) and 2(c), respectively. The air–metamaterial interface is at x = 0 mm, and the interface between the impedance-matching (IM) layer and the normal coiled-up structure is at x = 42 mm. By adding the impedance-matched structure, the effective parameters gradually increase between the two interfaces instead of a sharp rise, which is beneficial for transmission enhancement. The sound wave transmission mechanism inside the coiled-up acoustic meta-structure is attributed to the Fabry–Perot resonance.2,25) The acoustic transmission efficiency is enhanced by the Fabry–Perot resonance, which induces destructive interference between the sound wave reflections.32)
Download figure:
Standard image High-resolution imageDownload figure:
Standard image High-resolution imageFig. 2. (a) Three designs of 2D acoustic amplification resonators with different IM layers; (b) the calculated effective impedance distribution along the sound wave propagation direction; (c) the calculated effective refractive index distribution along the sound wave propagation direction. The shadow region indicates the normal coiled-up structure with specific effective parameters.
Download figure:
Standard image High-resolution imageNumerical simulation is performed using finite-element analysis software to study the effect of broadband acoustic amplification. The material properties of the air and poly(lactic acid) (PLA) are presented in Table I. The amplification ratio of the impedance-gradient meta-structure cavity is measured in an anechoic region using a sound pressure meter (Sauter SU130, 30–130 dB). The sound signals of a microphone and the output voltage of a piezoelectric plate are recorded using a data-acquisition device (National Instruments USB-6210). The designed meta-structure acoustic amplification resonator is made of PLA and was constructed via fused deposition modeling 3D printing (MakerBot Replicator Z18). The layer resolution was set as 150 µm to enhance the surface quality and accuracy. The height of three specimens was controlled below 100 mm to prevent the structure from warping in the top region. Figure 1(c) shows the three fabricated acoustic amplification resonators.
Table I. Parameters of the materials for the calculation.
PLA | Air (298 K) | |
---|---|---|
Density (kg/m3) | 1,210 | 1.1839 |
Sound speed (m/s) | 2,550 | 346.13 |
Refractive index | 1.465 | 1 |
Impedance (N·s/m3) | 3,090,000 | 409.4 |
The simulation and experimental results for the SPL gain with different impedance-matched designs are presented in Fig. 3. The results in Fig. 3(a) show that the traditional half-wavelength amplification resonator produced a lower SPL gain than the quarter-wavelength amplification resonator. For the resonator with normal coiled-up space, the SPL gain becomes negative at 1,483 Hz, indicating that the sound attenuation completely replaced the sound amplification. In contrast, Figs. 3(b)–3(d) show that in the studied frequency range, the acoustic amplification resonators with IM layers exhibit more than one peak of the SPL gain and relatively broadband sound amplification performance. The higher effective refractive index and impedance prove that a higher amplification ratio can be achieved by the first IM layer design, as shown in Fig. 3(b). The highest SPL gain of 17.2 dB at the resonance frequency of 625 Hz is slightly different from the simulation results, which may be caused by processing imperfections and the oblique incidence sound waves. As shown in Figs. 3(c) and 3(d), the broadband performance of the other two designs is significantly improved compared with the working bandwidth of the traditional amplification resonator without the IM layer. The bandwidth of the amplification resonator is defined as the frequency range for which the corresponding SPL gain is higher than zero. By adding three different IM layers (a = 0.4, a = 1, and a = 3.4) to the quarter-wavelength resonator with a normal coiled-up structure, the bandwidths are increased from 1,483 Hz to 1,804, 1,982, and 2,043.3 Hz, respectively. The SPL gain in the valley region is increased by increasing the value of a, which provides better amplification over the whole bandwidth. This can be explained by the effective-parameter distribution along the sound wave propagation direction, as shown in Figs. 2(b) and 2(c). At the air–metamaterial interface, when the structure parameter a increases, the effective refractive index and impedance decrease, resulting in better IM between the two mediums.26) Therefore, to achieve a perfect broadband property, high values of a and n are necessary, but this inevitably yields a large IM structure. Similarly, with the increase of a, the resonant frequency of the acoustic resonator gradually increases, as the sound propagation length decreases. For sound wave amplification, the design with a high effective refractive index and a mismatched impedance between the coiled-up structure and the air gap can better confine acoustic waves in the cavity region. Thus, the IM is capable of enhancing both the SPL gain and the amplification bandwidth of the amplification meta-structure.
Fig. 3. Numerical simulation and experimental results for (a) the SPL gain of the quarter-wavelength resonator and half-wavelength resonator without IM structures and (b)–(d) the SPL gain of the quarter-wavelength resonators with different impedance-matched designs, for a = 0.4, 1, and 3.4. The SPL of the incident sound wave is 171 dB.
Download figure:
Standard image High-resolution imageThe acoustic amplification and attenuation behaviors inside the resonator structures are investigated according to the pressure field amplitude patterns. Figures 4(a) and 4(b) show the pressure amplitude distributions of the resonators with and without the IM layer at their resonant frequencies, indicating stronger localization in the improved design. In both cases, the pressure amplitudes originate from the traditional amplification resonator cavity, which contains double coiled-up spaces that have a lower amplification ratio and a narrower bandwidth compared with those of our new amplification resonator design, as shown in Figs. 3(a) and 4(c). Comparison of Figs. 4(d)–4(f) reveals that when the frequency of the incident sound wave is set as the second peak frequency of 1,590 Hz [Fig. 3(c)], the obtained acoustic pressure is lower compared with the value under resonance mode but still shows the amplification effect. However, the other two samples exhibit significantly worse localization or even sound attenuation under the same frequency, which confirms the better broadband property of our design. In the simulation, we neglected the friction loss, which largely originates from the air viscosity in the boundary layer, because the slit is not narrow enough to affect the sound wave in the entire slit region.2,33) However, the simulation results would be more reliable if the boundary-layer effect induced by the thermal viscosity in the acoustic air channel was considered.
Fig. 4. Amplitude distribution of the acoustic pressure field inside (a) the quarter-wavelength resonator with the IM layer (a = 1, n = 7), (b) the quarter-wavelength resonator without the IM layer, and (c) the half-wavelength resonator with double coiled-up spaces at the eigenfrequencies of 630, 810, and 1,100 Hz. (d)–(f) Pressure field amplitude patterns of the three amplification resonators at 1,590 Hz.
Download figure:
Standard image High-resolution imageAn energy-harvesting experiment is performed using an experimental setup that contains both an acoustic amplification resonator and a piezoelectric plate. The acoustic resonator with an IM layer of a = 1 is used to confine and amplify the incident sound wave. To realize electricity generation, poly(vinylidene fluoride) piezoelectric plate (Measurement Specialties LDT-028K) is placed at the center of the cavity. By reducing the effective vibration length of the piezoelectric plate, the structural eigenfrequency of the plate can be tuned to the desired frequency. To achieve a high energy-harvesting efficiency, the eigenfrequency of the piezoelectric plate is set as approximately 630 Hz, which matches the resonant frequency of the acoustic amplification cavity. The results for the measured output voltage under the working frequencies are presented in Fig. 5. The acoustic energy harvester with the well-designed amplification resonator can obtain a peak output voltage of 51.2 mV, which is 2.98 times higher than that of the single piezoelectric harvesting plate, and a broad bandwidth of approximately 2,000 Hz. This means that by adopting our design, the maximum efficiency be increased by a factor of approximately 8.87 under the same incident sound power and load resistance.
Fig. 5. Measured output voltage with respect to the frequency for the acoustic energy harvester with and without the acoustic amplification resonator. The acoustic resonator contains the impedance-matched structure of a = 1 and n = 7.
Download figure:
Standard image High-resolution imageIn summary, we demonstrated that by adding an impedance-matched meta-structure at the open end, high acoustic amplification and broadband performance can be achieved inside the resonator. By selecting different structural parameters of the sound wave guide, the effective parameters, such as the refractive index and impedance, can be modulated to satisfy the requirements of the gradient distribution. A sparse structure is beneficial for IM between the medium (air) and the metamaterial, but a dense structure can provide a high effective index. According to concurrent experimental and numerical simulation results, a high SPL gain over a large bandwidth can be obtained compared with the resonator without the IM layer. Owing to its improved electricity-generation performance, the minimized acoustic resonator with the impedance-gradient structure is expected to be applicable in many circumstances, such as strong acoustic wave localization, noise energy harvesting, and noise cancellation.
Acknowledgments
The authors acknowledge the support provided by the Singapore Maritime Institute research project (Project No. SMI-2015-OF-10), the Shenzhen Science and Technology Innovation Commission (Grant No. ZDSYS201703031748354), and the Development and Reform Commission of Shenzhen Municipality. The authors also greatly appreciate the support of the Humbolt Research Fellowship for Experienced Researchers and the Open Project (Project No. SKLA-2018-09) of the State Key Laboratory of Luminescence and Applications.