Abstract
The putative and potential ocean worlds of our solar system span the asteroid belt to the Kuiper Belt, containing within their icy shells past or present global saltwater oceans. Among these worlds, those bearing signs of present-day geologic activity are key targets in the search for past or extant life in the solar system. As the icy surfaces of these bodies are modified by geologic processes, landforms are erased and replaced through what is called "resurfacing." To avoid contaminating sites for robotic spacecraft exploration, planetary protection requirements obligate missions to these ocean worlds to demonstrate a less than 10−4 probability of introducing a viable terrestrial microorganism into a liquid water body. To constrain the probability of subsurface contamination, we investigate the interaction with geologic resurfacing on an active ocean world. Through the example of Europa, we show how the surface age can be used to constrain the resurfacing rate, a critical parameter to estimate the probability that nonsterile spacecraft material present on the surface is geologically incorporated into the subsurface, and extend this example to mission scenarios at Ganymede and Enceladus. This approach was critical to demonstrating compliance with planetary protection requirements for the Europa Clipper mission, reducing its probability of contamination by two to five orders of magnitude. We also show how a Europa lander mission might be brought close to complying with planetary protection requirements, that a Ganymede impactor could easily comply, and that the situation of Enceladus, while more complex, can greatly benefit from this approach.
Export citation and abstract BibTeX RIS

Original content from this work may be used under the terms of the Creative Commons Attribution 4.0 licence. Any further distribution of this work must maintain attribution to the author(s) and the title of the work, journal citation and DOI.
1. Introduction
One of the most important insights in planetary science in the past few decades is the discovery that many worlds throughout our outer solar system may hide vast global oceans of liquid water (Hendrix et al. 2019). Ocean worlds are thus the targets of numerous planned robotic missions, including NASA's flagship-class Europa Clipper mission (Howell & Pappalardo 2020), NASA's New Frontiers−class Dragonfly mission (Lorenz et al. 2018) and Juno extended missions (Bolton et al. 2017), and ESA's L-class JUICE mission (Grasset et al. 2013).
As the first discovered ocean world beyond Earth, Jupiter's moon Europa has captivated the science community and general public. The Voyager missions first revealed a disrupted surface at Europa renewed through recent or active geologic activity, and Galileo mission magnetometry indicated the presence of a global saltwater ocean beneath the icy shell (Carr et al. 1998; Kivelson et al. 2000; Stevenson 2000). Recent observations also suggest the presence of plumes that may spew internal water into space (e.g., Sparks et al. 2016; Jia et al. 2018; Paganini et al. 2020), indicating the potential for shallow liquid water reservoirs beneath Europa's icy surface.
Intense radiation from Jupiter at Europa's surface likely forms water and impurities into oxidants. Moreover, active geologic cycling of seawater through rocky material on the Europan seafloor is expected to be chemically reducing (Hand et al. 2007, 2017; Vance et al. 2016). The relatively young average surface age of Europa (∼40–90 Myr, >20 Myr; Bierhaus et al. 2009), coupled with observations of widespread geologic activity (e.g., Lucchitta & Soderblom 1982; Buratti & Golombek 1988; Greeley et al. 2000; Figueredo & Greeley2004; Doggett et al. 2009), indicates that geologic processes actively remove, modify, and replace surface material (Figure 1). Thus, the geologic processes responsible for changing the surface of Europa through time may transport material from the surface of the icy shell to the interior ocean (e.g., Kattenhorn & Prockter 2014; Kalousová et al. 2016), or from the interior ocean to the surface (e.g., Prockter et al. 2002; Howell & Pappalardo 2018), possibly creating or sustaining chemical disequilibria that affect the body's potential habitability (e.g., Chyba & Phillips 2002; Marion et al. 2003; Hand et al. 2007, 2009; Vance et al. 2016).
Figure 1. Artistic depiction of geophysical processes within Europa's icy shell that result in resurfacing, modified from Howell & Pappalardo (2020). Extensional processes related to band formation may expose new material at the surface, including material recently frozen at the ice−ocean interface. Convergent processes may remove material to the icy interior and eventually the ocean. Surface features potentially related to subsurface melt, like chaos, pits, and domes, may create transient habitable niches. Observations of potential water plumes emanating from Europa's surface may be further indication of subsurface liquid water reservoirs.
Download figure:
Standard image High-resolution imageBuilding on the discovery of Europa as an ocean world, the Galileo mission also found oceans beneath the icy surfaces of Ganymede and Callisto in the Jupiter system, and the Cassini mission discovered oceans at Enceladus and Titan in the Saturn system. Indeed, we now understand that active or past planetary oceans may occur on worlds from the asteroid belt to the Kuiper Belt (e.g., Hendrix et al. 2019).
Like Europa, Enceladus exhibits present-day geologic activity at its south pole (Porco et al. 2006; Smith-Konter & Pappalardo 2008; Kite & Rubin 2016), where water vapor from a deep reservoir vents to space. Moreover, Enceladus shows signs of recurrent global deformation and renewal (Squyres et al. 1983; O'Neill & Nimmo 2010) that may occur episodically for periods of ∼10 Myr with a periodicity of 100 Myr–1 Gyr (O'Neill & Nimmo 2010). Ganymede also shows signs of at least one period of renewed activity since its formation, though at a much longer time interval. Ganymede's surface is bisected into dark and light terrains; the dark terrain is older than 4 Gyr (e.g., Zahnle et al. 2003), while the grooved light terrain has an estimated surface age of between 400 Myr and 4 Gyr (Zahnle et al. 1998, 2003).
On each ocean world, the age of the surface provides insight into the frequency of surface renewal and subsurface activity, which constitute "resurfacing" (e.g., Figueredo & Greeley2004). In the context of planetary protection, we define resurfacing as the geologically facilitated start of surface modification, potentially initiating transfer to the subsurface, which may or may not bring a microorganism that has been introduced by robotic exploration into contact with liquid water. The myriad of resurfacing processes play a critical role in understanding the spatial and temporal distribution of contaminants introduced to the Europan subsurface. Because the icy shell structure can be highly uncertain (e.g., Ojakangas & Stevenson 1989; Billings & Kattenhorn 2005; Howell 2021), and geologic transport mechanisms are thus poorly constrained (e.g., Billings & Kattenhorn 2005; Howell & Pappalardo 2019; Walker et al. 2021), we conservatively assume that any process that initiates surface modification poses the potential to bring contaminants in contact with habitable environments.
Implementing current, planned, and potential future mission concepts to explore active ocean worlds in our solar system could lead to terrestrial microbes being transported from spacecraft hardware to an extraterrestrial environment favorable to life. To ensure that these future missions do not produce false positives of life in ocean worlds by detecting contamination by Earth microbes, planetary protection requirements have been placed as a primary constraint on mission development (NASA 2020).
Planetary protection policy currently requires that the probability of inadvertent contamination of an ocean or other liquid water body must be less than 1 × 10−4 per mission (NRC 2012). In this context, contamination is defined as the introduction of a single viable terrestrial microorganism into a liquid water environment within the roughly 1000 yr period of biological exploration, ending in the year 3000. 1
This study describes in detail a critical component of the planetary protection strategy for the Europa Clipper mission, which is planned to launch in 2024 and make several dozen flybys of Europa (Howell & Pappalardo 2020). To conservatively demonstrate compliance with planetary protection requirements (DiNicola et al. 2018; McCoy et al. 2020), Europa Clipper utilized this notion of geologic resurfacing. Here we elaborate on the geologic basis for the resurfacing model summarized in McCoy et al. (2020) but also make mathematically rigorous the modeling of the resurfacing rate and its connection to the observed average surface age of an ocean world. A Poisson process model is developed to assess the probability of resurfacing in a contaminated region within timescales relevant to planetary protection, treating the resurfacing process as discrete spatial events that occur over time, informed by a range of resurfacing rates based on the average observed age of the surface. This model readily adapts to other icy bodies suspected to be geologically active in the sense of potential downward transport, providing a framework for other missions to demonstrate planetary protection compliance. Moreover, this study generalizes the probability of contamination model from McCoy et al. (2020) to consider missions that plan to make contact with the surface of an icy ocean world and shows how the resurfacing model can help bound the probability of contamination. We discuss the application of this model to Europa Clipper and illustrate how the model might be applied to a hypothetical lander mission to Europa. We also show how this model can help bound the contamination risk of missions to ocean worlds other than Europa, such as Enceladus and Ganymede.
Note that the purpose of this study is not to create a model for idealized geologic behavior according to our current best understanding of the body but to derive conservative constraints on the interaction between Europa's surface and subsurface bounded by our current understanding of these processes. After we develop these constraints, we will revisit certain assumptions and discuss possible ways conservatism might be reduced.
2. Geologic Basis for Resurfacing Approach
Here we develop the basis for the resurfacing approach using geologic arguments for Europa. This will provide not only a concrete illustration of the approach to this especially relevant ocean world but also the framework for adapting the approach to other ocean worlds in Section 4.
The thickness of Europa's icy shell is poorly constrained, with a current best-estimate thickness of km (Howell 2021). Perched water lakes may be present for short periods within kilometers of the surface beneath tectonic features (e.g., Kalousová et al. 2016; Hammond 2020), chaos terrains (e.g., Schmidt et al. 2011), pits and domes (lenticulae; Sotin et al. 2002; Manga & Michaut 2017), smooth plains (Fagents 2003; Lesage et al. 2020), and impact craters (Steinbrügge et al. 2020). Further, indirect evidence for potential water plumes (e.g., Sparks et al. 2016; Jia et al. 2018; Paganini et al. 2020) may indicate that melt is being rapidly transported upward from a subsurface melt reservoir and erupted (e.g., Lesage et al. 2020).
However, the existence and temporal longevity of putative reservoirs of liquid water within Europa's ice shell are debated (e.g., Collins & Nimmo 2009; Kalousová et al. 2016; Lesage et al. 2020; Howell et al. 2021a), and it is unclear whether geologic processes maintain a sustained connection between the surface and subsurface (e.g., Kattenhorn & Prockter 2014; Howell & Pappalardo 2019; Hammond 2020; Walker et al. 2021). To create a robust approach to quantifying geologic processes for planetary protection applications, we therefore take a conservative approach that is agnostic to the specific mechanisms of resurfacing and the relationship of these various processes to the creation and sustenance of habitable environments. Instead, we characterize resurfacing processes through their observable influences on the surface, which can be constrained by spacecraft observations.
The approach described here is conservative because (1) it assumes the potential for instantaneous contamination of the subsurface at the onset of geologic activity and (2) it is insensitive to any particular geologic resurfacing or transport mechanism. Given the appreciable uncertainties that exist regarding the specifics of icy shell processes, we hold that this conservatism is warranted as to not introduce spurious information to this study.
Different resurfacing mechanisms on Europa operate over a wide range of spatial and temporal scales (Figure 2). For example, individual extensional bands may expose >104 km2 of fresh surface material through faulting and solid-state convection over timescales of 105–106 yr (Pappalardo & Sullivan 1996; Howell & Pappalardo 2018). Additionally, convergent margins have been observed where the cold and brittle surface ice is driven into the warmer icy interior of the outer shell before reincorporation. This is a process known as "subsumption" (Kattenhorn & Prockter 2014). These subsumption zones are >1000 km in length and may remove material toward the interior ocean at rates of tens of millimeters per year (Kattenhorn & Prockter 2014). Chaos features may form in response to rapid, large-scale subsurface melting affecting areas 102–105 km2 (Schmidt et al. 2011). Impacts of the surface by comets and other objects may allow some transport beneath the icy shell through geologic time and sustain melt reservoirs (Steinbrügge et al. 2020), though observations of Europa show that shell-penetrating impacts that would directly deliver material to the subsurface ocean are uncommon throughout its recent history (Moore et al. 1998; Zahnle et al. 1998; Turtle & Pierazzo 2001; Schenk 2002). In addition to the above, frictional heating on faults has been proposed to produce melt beneath double ridges that may drain to the subsurface ocean over timescales of 103–105 yr (Kalousová et al. 2016; Hammond 2020). While each geologic process may influence a unique area of the surface for a unique time, each contributes to an average exposed surface age that is likely between 40 and 90 Myr, based primarily on estimates from crater size–frequency distributions, but accounting for uncertainties in impact rates and spacecraft imagery coverage could extend this range to 20–200 Myr (e.g., Zahnle et al. 1998; Bierhaus et al.2009).
Figure 2. Mosaic of Galileo spacecraft images showing the region surrounding the large extensional band Phaidra Linea, and the different terrain types observed on Europa that correspond to different physical mechanisms of surface modification. Each process operates over different spatial and temporal scales. Highlighted within the image are (a) a large region of chaos terrain (red) comprising blocks of material that preserve older structures and smooth matrix material (∼5 × 103 km2 in area); (b) the prominent extensional band Phaidra Linea (blue) (>105 km2); (c) two out of dozens of visible double ridges (yellow), potentially formed by strike-slip motion, cutting across older terrains (103–104 km2); and (d) arrows pointing to three out of dozens of visible pits and domes (purple) (∼102 km2 each in this image).
Download figure:
Standard image High-resolution imageYounger surface ages imply higher rates of geologic activity that remove, replace, or modify older surface material. Potential contaminants placed on the surface of a body that maintain a young surface might participate in geologic activity quickly, while contaminants placed on an old surface might not participate in any geologic activity for extended periods of geologic time. While on the surface, microorganisms would continue to be exposed to lethality factors such as Jovian radiation and hard vacuum that may not persist once transferred into the subsurface. Thus, for the purposes of this study, conservative constraints are best derived from the youngest potential age estimations for the surface of Europa.
Because we are unable to observe the geologic evolution of Europa's outer icy shell prior to the formation of the present-day surface, we assume that observations of Europa today are applicable for this conservative resurfacing study. Specifically, we assume that resurfacing on Europa is unlikely to produce an average surface age younger than 20 Myr over timescales much smaller than 20 Myr. We therefore select an average surface age of 20 Myr as our initial condition at the time of a future spacecraft coming into contact with Europa. 2 This is formalized by assuming that, on the timescale relevant to biologic exploration for planetary protection requirements (103 yr), the average surface age remains constant. We assume that the conservatively young average surface age, αE = 20 Myr, is maintained across all regions of Europa, corresponding to a resurfacing rate of λ = 1/αE for each region. The inverse relationship between resurfacing rate and the time-independent average surface age (especially for timescales ≪ αE, such as the period of biological exploration) results in a resurfacing rate that is also constant over the timescales relevant to this study. The mathematical reasoning for this relationship is discussed in Section 3.2.
Thus, while some regions of the body may resurface during or very soon after a robotic mission, others may not exhibit activity for tens or hundreds of millions of years afterward. The average surface age adopted here comprises the totality of regional ages on the body, and activity within a small region over an anomalously short or long interval does not greatly influence the global resurfacing rate.
Stratigraphic relationships on Europa are complex, and older terrains are a generally poor predictor of current geologic activity (Greeley et al. 2000; Figueredo & Greeley 2004; Doggett et al. 2009; Leonard et al. 2018b). Because past geologic change recorded in the icy surface is not a reliable indicator of future geologic resurfacing, we assume that resurfacing is equally as likely anywhere on the body at any given time. That is, because geologic features vary widely in time and space, we assume that each potential resurfacing event is independent of any other, and not an indicator of past or future activity in that region. This conservative approach assumes that the poles, leading and trailing hemispheres, and Jovian and sub-Jovian hemispheres all experience a high level of geologic activity at present day, resulting in a young average global surface age. We thus formally ignore any potential spatial variations in geologic activity, which may manifest due to, for example, spatial variations in tidal activity (e.g., Ojakangas & Stevenson 1989) or be concealed by poor global coverage of spacecraft observations (e.g., Phillips et al. 2000; Doggett et al. 2009; Leonard et al. 2018b; Schenk 2020).
As the average global surface age of Europa provides an upper bound on the resurfacing rate for the body, mechanical arguments can provide an approximate lower bound on the size of geologic regions that may spontaneously experience surface modification. The depth to which the cold, upper portion of the icy shell deforms through brittle failure on faults and cracks is predicted to be ∼3–7 km (Howell 2021), and individual estimates of the brittle thickness are no thinner than ∼1 km (Billings & Kattenhorn 2005). In general, tectonic features that penetrate the brittle layer, permitting subsurface transportation, should have lengths greater than the brittle thickness (e.g., Scholz et al. 1993). We therefore assume conservatively that the spatial dimensions of a resurfacing area on Europa may be as small as 1 km2, as described below.
We note that Phillips et al. (2000) performed a thorough search for change detection on Europa's surface between the Voyager and Galileo missions and found that the lack of observed change implies a maximum resurfacing area of 4 km2 over 20% of the surface. However, continued reanalysis by Schenk (2020) of observations spanning cy 1979–2007 found no evidence for small-scale resurfacing. Thus, we assume that spatial scales of ≥1 km2 facilitate the majority of resurfacing and downward material transport on Europa, capturing the potential for small resurfacing areas (e.g., Phillips et al. 2000) without allowing an unbounded minimum resurfacing area to drive the analysis. This value is consistent with the comparatively large extents covered by features relatively recent in the stratigraphic column (Figueredo & Greeley 2004; Leonard et al. 2018a), including extensional and convergent bands (Kattenhorn & Prockter 2014; Howell & Pappalardo 2018), chaos regions (Collins & Nimmo 2009; Schmidt et al. 2011), lenticulae (Sotin et al. 2002), ridge features (Hammond 2020), and large melt-producing impacts (Steinbrügge et al. 2020).
This conservative interpretation of the geologic environment maximizes the potential for spontaneous downward transport into the icy shell and resulting contamination of the subsurface. Resurfacing is assumed to occur regularly across the body through the smallest-scale processes that penetrate the brittle layer. In an alternative scenario, we could assume that the maximum resurfacing area for a single event on Europa is the entire surface area of the satellite and that resurfacing occurs catastrophically everywhere. In both scenarios, the probability of resurfacing is controlled by λ such that the average surface age of 20 Myr is maintained. These end-members are explored further in Section 5.
3. Mathematical Method
The geologic basis for resurfacing developed in Section 2 allows us to derive a mathematical model of resurfacing suitable for planetary protection contamination risk assessment of a mission to an ocean world. We now develop this model by first showing in Section 3.1 that this geologic basis describes what is called a Poisson process. A critical parameter of this model is the rate at which resurfacing occurs. How the resurfacing rate is derived from the average observed age of the ocean world's surface is the topic of Section 3.2. Finally, how the resurfacing model is incorporated into the larger probability of contamination model is discussed in Section 3.3.
3.1. A Mathematical Model of Resurfacing
As discussed in Section 2 for Europa, historical geologic activity is not a reliable indicator of present or future geologic modification (e.g., Doggett et al. 2009; Leonard et al. 2018b), and the lower bound of spatial scales for structures involved in resurfacing events is conservatively assumed to be no less than 1 km2 (Scholz et al. 1993; Billings & Kattenhorn 2005; Howell 2021). Our resurfacing model therefore partitions the surface of an ocean world into a finite number, G, of square kilometer grid elements vulnerable to an independent resurfacing event. The size of the grid element is an assumption that will be revisited in Sections 4 and 5 as we apply this model to non-Europan ocean worlds and address conservatism in the modeling.
When the average surface age should not change significantly over geological timescales (as is the case on Europa, discussed in Section 2), it can be shown (Section 3.2) that the rate at which a given grid element is thought to resurface can be conservatively taken to be a fixed value, λ, equal to the reciprocal of the estimated surface age.
Let Prk
(t) be the probability that a given grid element of an ocean world undergoes its kth resurfacing event by time t. Characterizing the resurfacing process at time t + Δt in terms of how many resurfacing events have occurred by time t, the probability that a given grid element undergoes its resurfacing event is described by the equation

subject to the initial condition Pr0(0) = 1 (no resurfacing event occurs at time 0), and higher-order terms are ignored (two or more resurfacing events occurring to the same grid element has a vanishingly small probability over smaller and smaller time intervals). Here λΔt is the probability that the next resurfacing event occurs in some small increment of time, Δt. Using mathematical induction on k, the general solution to this recursion can be written as

which gives the probability that a given region has had exactly k resurfacing events by time t and is recognized as a Poisson process with rate λ. Figure 3 provides an illustration of the resurfacing model given by Equation (2) using a Monte Carlo model. This illustration considers the surface of Europa broken into equal-area grid elements. Initially, the surface has an average age of 20 Myr and a prescribed 20 Myr resurfacing rate. At each time step of length Δt, each grid element will resurface independently of the others based on a random draw from the unit interval if this draw is less than λΔt; otherwise, the grid element does not resurface. The average age of the surface after each time step is then updated, and the simulation continues.
Figure 3. Monte Carlo simulation illustrating the resurfacing model described by Equation (2). The background map of Europa is a Mollweide projection of the Europa geologic map (Leonard et al. 2018b), included here as a reminder of a critical assumption of this model that the resurfacing behavior cannot be predicted based on current knowledge of the terrain. Grid lines represent regions of equal area shown for 6.25 × 104 km2 to illustrate resurfacing, but we implemented 1 km2 in the analysis because smaller values are more conservative. At (a) time t = 0, the average surface surface age is 20 Myr and new periods of geologic activity are recorded. As time progresses (panels (b)–(d)), regions are highlighted with a transparent orange overlay to show that resurfacing has occurred within that region. Regions darken as resurfacing occurs repeatedly in the same location. The average surface age of ∼20 Myr is maintained through time. Note that after 20 Myr of elapsed time, some regions may not have had any geologic activity, while others may have undergone multiple resurfacing events. In this way, multiple timescales and spatial scales are represented by this simplified model. An animated version of this figure is available online and in the online Journal; duration: 25 s. Animation time stamps 0:00:00–0:00:12 simulate an elapsed time of approximately 30 Myr on Europa, during which the average surface age changes from 20.00 to 21.24 Myr as resurfacing events occur randomly within discrete grid elements. From time stamp 0:00:12 to the end of the animation at 0:00:25 the animation continues simulating an additional 30 Myr on Europa. The average surface age can be seen converging to the reciprocal of the resurfacing rate, as discrete resurfacing events continue. The average surface age remains constant, given some minor random fluctuations, thereafter.
(The data used to create this figure are available.)(An animation of this figure is available.)
Download figure:
Video Standard image High-resolution imageImportantly, a consequence of this model is that the time until the next resurfacing event is memoryless. Formally, the memoryless property of a continuous random variable T means that , for all t > v > 0. For example, say we know that a resurfacing event has not happened for over 1 Myr. The memoryless property implies that this knowledge does not affect the probability that a resurfacing event happens some number of years in the future. The probability has no "memory" of the past, and the timing of the next resurfacing event can be evaluated as if the resurfacing process is starting over. In fact, it is a well-known result of probability theory that, for a continuous memoryless process, the time between events follows an exponential distribution. Hence, the process implied by Equation (2) describing the number of resurfacing events is equivalent to an exponential distribution that describes the time between resurfacing events. This equivalence allows us to analytically derive the resurfacing rate based on the average observed surface age of the ocean world being investigated.
3.2. Derivation of the Resurfacing Rate
Determination of the resurfacing rate, λ, for an ocean world is of central importance to the resurfacing model given by Equation (2). For Europa, observational images of the surface and estimates of crater age provide an average age of the surface between 20 and 200 Myr (e.g., Bierhaus et al. 2009). With this as the only source of data to determine λ for Europa, the resurfacing model selects a reasonably conservative value for this parameter such that the global average surface age is maintained at a value in this range over time. While this is the case for Europa, it may not be the case for other ocean worlds (e.g., Enceladus), so care must be taken when deriving the resurfacing rate in general (examples are given in Section 4).
Let a0 be the surface age of a particular grid element of the ocean world's surface at the beginning of the most recent era of resurfacing processes, which occur at some fixed rate λ. Appendix C shows that the expected age of this grid element at time t is

where t = 0 is taken as the start of this era of resurfacing processes. Hence, the resurfacing rate is related to the average observed age of this region by way of its initial age at the time this resurfacing process began and the time at which we observe this region in the process. Note that as t goes to infinity—as we observe this region further and further into an era of resurfacing—the average observed age converges to the reciprocal of the resurfacing rate. Moreover, if the resurfacing rate is equal to the reciprocal of the initial age a0, then the average observed age at any time t is constant, equal to 1/λ. Finally, assuming that all grid elements have a similar initial age (e.g., due to a widespread geological transition from an era of quiescence to an era of activity), this same expression governs the global average observed age of the ocean world.
Figure 4 shows the expected age of a grid element on Europa over time as calculated by the expression in (3). Each panel shows a dashed gray line at 20 Myr, the minimum average age of the Europa surface consistent with observation. Panel (a) shows an extremely low initial age of 1000 yr, together with the average age of this region observed throughout time, for various resurfacing rates. Other panels show this information for initial ages of (b) 1 Myr, (c) 20 Myr, and (d) 1 Gyr. The different curves in each panel show the average age for a range of values selected for the resurfacing rate, λ. The special case when is accented with a thicker line in each panel.
Figure 4. Expected surface age of a grid element as a function of the time of observation given various average resurfacing rates, as calculated using the expression in (3), when the initial age of the region is (a) 1 kyr, (b) 1 Myr, (c) 20 Myr, and (d) 1 Gyr. Assuming that the observed average age is not expected to change significantly over timescales equivalent to the observed surface age, we find ourselves on the right side of each of these graphics, where the average age has reached steady state. In these cases, the resurfacing rate, λ, can be set equal to the reciprocal of the average observed surface age. For Europa, this value can be no greater than 1/20 Myr−1.
Download figure:
Standard image High-resolution imageAs can be seen in this figure, the observed average age converges to 1/λ once t reaches . We also note the following:
- 1.To observe a 20 Myr average age at some point in time, t, when a0 is less than or equal to 20 Myr, λ must be less than
yr−1. For example, when a0 = 1 kyr, the red dashed line in Figure 4(a) only intersects curves with λ less than
yr−1.
- 2.To observe a 20 Myr average age when a0 is greater than or equal to 20 Myr, λ must be greater than
yr−1.
- 3.To observe a 20 Myr average age when a0 is equal to 20 Myr, λ can be anything as long as the observation is made soon enough.
If it can be justified that the observed average age is not expected to change significantly for some amount of time that is geologically relevant, as is the case made for Europa in Section 2, then we find ourselves looking at portions of each of the graphics in Figure 4 where the time of observation is >106 yr and the average age has converged to 1/λ. In this case, values of λ higher than yr−1 can be ruled out even for cases where the initial age is thought to be higher than 20 Myr. In fact, the only values of a0 and λ that guarantee overall maintenance of the average surface age of Europa, αE, are when a0 = αE and
. This provides a conservative position taken by this resurfacing model for Europa: since resurfacing is unlikely to produce an average surface age younger than 20 Myr over timescales much smaller than 20 Myr, an average surface age of 20 Myr is used as the initial condition at the time a spacecraft comes in contact with Europa, as shown in Figure 4(c).
3
Other, less conservative positions for Europa applications are also considered by the resurfacing model, where the observed average age is as high as 200 Myr and is not expected to change significantly for a geologically significant amount of time.
3.3. Assessing the Probability of Contamination
In general, a mission can contaminate an ocean world in one of two mutually exclusive ways: (1) by way of its nominal mission, if it plans to come in contact with the ocean world (e.g., through in situ exploration); or (2) through some off-nominal mission scenario where there is a spacecraft failure (e.g., a maneuverability failure) leading to contact (possibly an inadvertent impact) of the spacecraft with the ocean world. In further detail, the event that contamination occurs is equivalent to the following:
- (1)The spacecraft contaminates the ocean world by way of its nominal mission, which occurs if
OR
- (2)The spacecraft contaminates the ocean world by way of some off-nominal event, which occurs if
Let E0 denote the event that the nominal mission is successfully completed as planned and contact is made with the ocean world as in (1a). Let u be the time elapsed since the launch of the spacecraft. Denote by E1(u) the event that an off-nominal event leading to contact with the ocean world occurs as a result of a failure at time u as in (2a), and denote its probability density function by f1(u). The probability of each of these events can be assessed for a given mission by way of reliability analysis and assessment of the mission design (DiNicola et al. 2018; McCoy et al. 2020). Since these events are mutually exclusive, the probability that some off-nominal event leading to contact with the ocean world occurs over the course of the mission is

where E1 is the union of all events E1(u), where u ∈ (0, ∞). Note that the function f1 will likely become identically zero beyond some finite value of u since the spacecraft will typically be decommissioned and disposed of in some way that precludes further operations.
Let Rτ denote the event that a microorganism, originally on board the spacecraft, resides on an area of the ocean world that resurfaces within the period of biological exploration, where τ is the calendar year marking the end of the period of biological exploration (τ = 3000 under current NASA planetary protection policy). The number of grid elements of the ocean world's surface with a microorganism delivered by the spacecraft can be conservatively bounded through impact and debris modeling (McCoy et al. 2020). The speed at which the spacecraft contacts the ocean world, as well as the shallowness of the contact angle relative to the surface (sometimes referred to in terms of "obliquity"), and ice properties (most notably, ice porosity) have been shown to be important drivers in these analyses (McCoy et al. 2020). The speed and angle at which the spacecraft contacts the ocean world, as well as location and time of the spacecraft's initial contact, are typically provided by way of Monte Carlo simulation of the spacecraft trajectory and orbital dynamics (e.g., Arrieta et al. 2016). Ice porosity can be a highly uncertain quantity for these worlds and is set to zero in the modeling. This is a conservative value from a planetary protection perspective, representing hard ice that leads to increased breakup of the spacecraft and ricochet of fragments, resulting in a larger amount of the surface potentially being contaminated.
The Monte Carlo analysis is performed such that the sample size M is large enough to describe the distributions of these driving parameters. For off-nominal scenarios, trajectories are evaluated based on the time u of failure and predicted orbital dynamics at that time. In this case the number of trajectories simulated will depend on u and be denoted by Mu
. In practice, a finite number of times u will suffice to assess the probability of contamination, such as the times of maneuvers of an orbiter or separation events of a landing system. For example, the probability that an off-nominal event leading to contact with a given ocean world occurs as a result of a failure between two consecutive maneuvers at times u1 and u2, u2 > u1 can typically be given by , where the form of f1 is a continuous reliability function multiplied by a single probability value of being on a trajectory that impacts the ocean world, determined by the maneuver completed at time u1.
Through analysis of each trajectory bringing the spacecraft in contact with the ocean world, as well as a conservative assessment of factors pertaining to impact dynamics (e.g., material properties of the spacecraft and surface ice), an upper bound on the number of grid elements of the ocean world's surface with a microorganism delivered by the spacecraft is determined, denoted by Am (u). Note that the event Rτ is equivalent to one or more of these Am (u) grid elements resurfacing within the period of biological exploration.
The event that one or more microorganisms launched with the spacecraft survive to come in contact with the subsurface is denoted by S. Biological analysis of microorganism mortality, potentially lethal environmental conditions to which the spacecraft is exposed, and microbial abundance inform the probability calculation in this part of the model (McCoy et al.2020).
Finally, a postulate of the modeling is that liquid water within the ocean world can be contaminated by a microorganism delivered by a spacecraft only if a resurfacing event occurs within the region hosting that microorganism after it was delivered to that location. Hence, contamination is precluded until the first resurfacing event occurs to an area of the ocean world that contains a microorganism delivered by the spacecraft.
The probability of contamination due to the nominal mission, described by (1a)–(1c) above, is given by Pr[E0 ∩Rτ ∩ S] = Pr[E0] × Pr[Rτ ∣E0] × Pr[S∣E0 ∩ Rτ ], where the law of total probability and definition of conditional probability have been used to give the right-hand side of the equality. Similarly, the probability of contamination by way of an off-nominal event caused by a failure occurring at time u, described by (2a)–(2c) above, is given by f1(u) × Pr[Rτ ∣E1(u)] ×Pr[S∣E1(u) ∩ Rτ ] du, where du is a small interval about the time u of failure leading to the spacecraft coming in contact with the ocean world. By integrating over all possible failure times, the total probability of contamination, PC , is calculated as

To understand how to calculate the conditional probabilities of resurfacing in Equation (5), we proceed as follows, first for Pr[Rτ ∣E1(u)] and then for Pr[Rτ ∣E0].
Assume that a failure occurs at time u, that contact with the ocean world occurs on trajectory m at time tm (u) ≤ τ, and that the number of grid elements of the surface hosting a microorganism delivered by the spacecraft is Am (u). The conditional probability of the event Rτ , denoted by Pr[Rτ ∣E1(u)] in Equation (5), is therefore the probability that one or more of the Am (u) grid elements resurface within the period of biological exploration.
Consistent with the geological basis for resurfacing in Section 2, this model remains agnostic to the particular mechanism of surface modification that may be acting in any given region and assigns the same constant resurfacing rate to each grid element in a way that maintains the average observed surface age. Note that this results in the model being more conservative, from a planetary protection perspective, the younger the assumed surface age. We therefore apply Equation (2) with the same value of λ to each individual grid element. Hence, the probability that an individual grid element does not resurface within the period of biological exploration is calculated by setting k = 0 in Equation (2), equal to .
Following Section 2, we conservatively assume that knowledge of a resurfacing event in one region does not provide additional information to estimate the probability that another grid element will resurface. The model, therefore, treats resurfacing events between grid elements as independent of one another. That each grid element resurfaces independently implies that the conditional probability that none of the Am (u) grid elements resurface is equal to

Therefore, the probability that one or more of the Am (u) grid elements experiences a resurfacing event within the period of biological exploration—and a microorganism, originally on board the spacecraft but now residing on one of the Am (u) grid elements of the ocean world, comes in contact with the subsurface—is equal to

Summing these probabilities over all possible trajectories m that contact the ocean world when a failure occurs at time u gives the conditional probability that a microorganism, originally on board the spacecraft, is brought into contact with the subsurface of the ocean world within the period of biological exploration:

Note that when tm
(u) > τ, as we are only concerned with resurfacing events that occur within the period of biological exploration.
Similarly, the conditional probability that a microorganism originally on board the spacecraft is brought into contact with the subsurface of the ocean world within the period of biological exploration (that is, prior to the year 3000) when the nominal mission completes successfully is given by

where dependency of terms on u has been removed since no failure leading to off-nominal contact is applicable.
For the case of Europa, modeling of the surface age (Zahnle et al. 1998; Bierhaus et al. 2009), together with the discussion in Section 2, provides a range of possible resurfacing rates. From a planetary protection perspective, the worst case, yr−1, utilizes the smallest average surface age estimate since it maximizes the resurfacing rate and therefore the probability of subsurface contamination. A "current estimate" is
yr−1, which uses the best-informed current surface age. Finally, the best case,
yr−1, utilizes the largest average surface age estimate. Note that each of these resurfacing rates, λ, carries with it the assumption that the average surface age is unlikely to change significantly over a timescale of
.
3.3.1. Bounding the Probability of Contamination
The resurfacing method described here provides a simplified avenue for developing conservative estimates of the probability of contamination that incorporate geologic principles. Modeling the events related to microorganism survival in Equation (5) requires complex biological models outside the scope of this study. An alternative bounding approach can be implemented using the resurfacing model, together with a top-level assessment of failure and impact probabilities. In this case we assume that the probability of survival given that a microorganism is brought into the subsurface is equal to unity. This is conservative, but not overly so in the context of the results demonstrated by Europa Clipper (see Table 1).
Table 1. Results for the Best-case, Current Best Estimate (CBE) Case, and Worst-case Input Parameters for the Probability of Contamination of Europa by the Europa Clipper Mission, as Well as the Decomposition of This Probability into Its Constituent Elements Involving Impact, Resurfacing and Survival. Reproduced from McCoy et al. (2020)
Probabilities of Interest | Best Case | CBE Case | Worst Case |
---|---|---|---|
Probability of impact | 4.20 × 10−3 | 1.25 × 10−2 | 2.43 × 10−2 |
Probability of resurfacing, given impact | 6.56 × 10−5 | 2.66 × 10−3 | 3.47 × 10−2 |
Probability of survival, given impact and resurfacing | 6.98 × 10−1 | 6.75 × 10−1 | 9.79 × 10−1 |
Probability of contamination | 1.92 × 10−7 | 2.25 × 10−5 | 8.24 × 10−4 |
Note. Best-case, CBE, and worst-case parameters used in the resurfacing model calculations were ,
, and
yr−1, respectively, with a grid element size of 1 km2 in all cases.
Download table as: ASCIITypeset image
Suppose the number of grid elements associated with nominal and off-nominal scenarios can be bounded from above by and
, respectively. Note that since all terms are probabilities (thereby taking values on the unit interval), the probability of contamination given by Equation (5) can be bounded by the resurfacing model as follows:
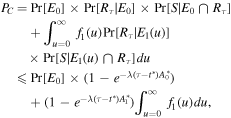
where t* is taken to be a conservative time of the earliest spacecraft contact possible with the ocean world.
Using Equation (4), the result above becomes

Example calculations for hypothetical missions to Europa, Ganymede, and Enceladus are shown and discussed in Sections 4.2–4.4.
4. Model Applications
Four applications of the resurfacing model and its effect on the probability of contamination are discussed here. First, we discuss its role in demonstrating compliance with the Level 1 Planetary Protection Requirement for the Europa Clipper mission presented in McCoy et al. (2020). To illustrate how the resurfacing model can help bound the contamination risk for other kinds of missions to Europa, we apply the resurfacing model to a hypothetical Europa lander mission concept. Finally, we apply the resurfacing model to hypothetical missions impacting Ganymede, as well as an Enceladus orbiter, in order to demonstrate how the resurfacing model might be adapted to help bound the probability of contamination of missions to non-Europan ocean worlds.
4.1. Application to Europa Clipper
The Europa Clipper mission utilized the resurfacing model from Equation (8) as part of its calculation of the probability of contamination. The resurfacing model presented in McCoy et al. (2020) differs slightly in its formulation from that shown in this study in that it also includes a sum over potential additional factors such as spacecraft orientations and ice properties at impact, relevant to the models of organism survival, but not significant to the resurfacing model. In addition, note that the probability of contamination model in McCoy et al. (2020) is based on a particular case of the integral shown in Equation (5); the top line of Equation (5) to the right of the equals sign is not applicable to Europa Clipper since the mission does not intentionally contact Europa, and the integral in Equation (5) is taken to be a sum over all maneuvers in the Clipper tour that could lead to a contamination event.
Reproduced from McCoy et al. (2020), Table 1 shows the results using the planned trajectory at the time of the Critical Design Review in 2020 December for the CBE, best-case, and worst-case input parameters for the probability of contamination of Europa by the Europa Clipper mission, as well as the decomposition of this probability into its corresponding probabilities of impact, resurfacing (given impact), and survival (given impact and resurfacing). The model formulation and all model inputs were agreed to via the Memorandum of Understanding between the NASA Office of Planetary Protection and Jet Propulsion Laboratory, including the current estimate and worst-case resurfacing rates from Section 3.3.
The resurfacing model, together with the agreement to a period of biological exploration ending in the calendar year 3000, proved to be mission enabling for Europa Clipper to demonstrate compliance with the Level 1 Planetary Protection Requirement. As can be seen in Table 1, and as McCoy et al. (2020) discuss in detail, the probability of inadvertent impact (4.20 × 10−3–2.43 × 10−2) was not sufficiently low to satisfy the planetary protection requirement on its own, and biological considerations had an almost negligible effect on the probability of contamination (6.75 × 10−1–9.79 × 10−1). However, the resurfacing model reduced the probability of contamination by 2–5 orders of magnitude (6.56 × 10−5–3.47 × 10−2), bringing the CBE probability of contamination below 1 × 10−4 as required, without having to leverage biological aspects of the model. Notably, the resurfacing model also provided three out of the top five most influential parameters to the probability of contamination model. These parameters relate to disintegration and fragmentation of the spacecraft after an inadvertent impact, many of which contain high levels of uncertainty that cannot be reduced at this time or until the mechanical properties of Europa's icy shell are better characterized.
4.2. Application to a Hypothetical Europa Lander Mission Concept
Suppose a hypothetical mission concept plans to land on Europa in the year 2040. Assume that failure and impact analyses have been completed and have determined that there is a 95% chance that this mission will land on Europa's surface as planned and a 5% chance that a failure will occur that will lead to a shallow (oblique) angle impact on the surface. Finally, assume a resurfacing rate based on the youngest average surface age estimates, where each grid element resurfaces at an average rate of once every 20 Myr. For this example, the number of years remaining in the period of biological exploration is bounded by 3000 − 2040 = 960 yr. Although, in practice, the time of each trajectory coming in contact with Europa should be considered on a case-by-case basis, 960 yr will be assumed to be the time remaining in the period of biological exploration for all trajectories in this simplified example.
In addition, suppose for all landing cases and that impact modeling has shown that the number of Europa surface grid elements with a microorganism delivered to them is bounded above by
for all oblique impact cases. Each of these cases is illustrated in Figure 5. Under these assumptions, the upper bound on the probability of contaminating Europa can be calculated using Equation (10):
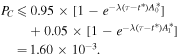
A less conservative assumption is that the resurfacing rate corresponds to an average surface age of 65 Myr (e.g., Bierhaus et al. 2009). Keeping all other inputs the same, the probability of contamination is then bounded by 5 × 10−4. In this case, the hypothetical mission is close to meeting the planetary protection requirement. Further refining the analyses (e.g., factoring in biological considerations, more detailed trajectory analysis), making less conservative assumptions (e.g., 960 yr remaining in the period of biological exploration for all trajectories coming into contact with Europa), and performing targeted engineering trades to mitigate contamination risk could allow this mission to demonstrate compliance with the planetary protection requirement.
Figure 5. Illustration of the number of grid elements of the Europan surface affected by (a) a landing event or impact normal to the surface and (b) an oblique impact, and the probability that at least one of the affected grid elements resurfaces within the remaining years of the period of biological exploration (960 yr in this example). Grid elements brought in contact with spacecraft debris are highlighted in orange.
Download figure:
Standard image High-resolution image4.3. Application to a Hypothetical Ganymede Impactor
As a first application to a non-Europan body, we use the resurfacing model to bound the probability of contamination of an impactor mission to Ganymede. This also parallels the current design of the Europa Clipper mission, which tentatively plans to dispose of the spacecraft into Ganymede at the end of the approximately 4 yr prime mission, pending the proposal of an extended mission. 4 We do this by first briefly discussing geologic characteristics of Ganymede and why the resurfacing model developed for Europa can be conservatively applied to this ocean world. We then select a resurfacing rate based on a reasonable average surface age from the literature and use this to calculate a conservative bound on the probability of contamination. Note that our intention here is not to provide an in-depth discussion of Ganymede geology but to present to the reader the key considerations that need to be thought through in order to apply the resurfacing model. For the reader who would like a more thorough account of Ganymede's global geology, see Patterson et al. (2003).
As with Europa, Ganymede is an icy moon of Jupiter with negligible atmosphere and grooved terrain features that provide evidence of past geologic resurfacing (Patterson et al. 2003). Ganymede has significant differences from Europa, including a milder radiation environment, an ice shell no less than 50 km thick (Grasset et al. 2013), and a much older surface age as evidenced by its higher crater density. The average age of younger grooved terrain regions is estimated to be 2 Gyr (Zahnle et al. 2003), with other estimates ranging between 1 and 3 Gyr (Patterson et al. 2003; Zahnle et al. 2003; Grasset et al. 2013). There is no evidence of current surface activity, the youngest features being >100 Myr old, and only a remote chance that there exist shallow reservoirs in the first few kilometers of the icy crust of Ganymede (Grasset et al. 2013). It is argued in Grasset et al. (2013) that it would require millions of years for material to exchange between the surface and liquid layer owing to the thickness of Ganymede's stagnant upper lid, with 7000 yr being the minimum time required to exchange material through the convective sublayer. Given the lack of interstitial liquid water and the time required to reach a liquid ocean, contamination is likely precluded within the period of biological exploration. That said, we continue this example to show how the resurfacing model can quantify a conservative upper bound on the probability of contamination.
The key assumption that must be met to provide this upper bound is that contamination cannot occur until contact is made with the subsurface. The absence of an atmosphere, the extreme cold temperatures on the surface, and the lack of any liquid water close to the surface make this a plausible assumption. In order to apply the resurfacing model as developed for Europa, we must also satisfy four other assumptions:
- 1.Resurfacing events can be treated as discrete events occurring at a constant rate over time.
- 2.The average age of the surface will remain unchanged for geologic timescales comparable to the average surface age (allowing us to take the resurfacing rate to be the reciprocal of the average surface age).
- 3.Resurfacing events occur on regions (i.e., "grid elements") of a minimal size.
- 4.Resurfacing events on grid elements occur independently of one another.
If Ganymede is still geologically active, the average surface age estimates, combined with similarities of the surface terrain with Europa, give credibility to (1), (2), and (4). Since the brittle lithosphere is thought to be much thicker on Ganymede than on Europa (Howell & Pappalardo 2019), we predict that the minimum resurfacing size should be greater than the value assumed for Europa, as discussed in Section 2 of this study. Additionally, the smallest landforms potentially related to resurfacing on Europa, such as microchaos, are not observed on Ganymede (Pappalardo et al. 2004). Therefore, we conservatively assume in this example that the minimal size of resurfacing regions required in (3) is no less than the 1 km2 assumed for Europa.
With the assumptions above verified, we can calculate an upper bound on the probability of contamination of Ganymede from a hypothetical impactor mission. We will assume that the spacecraft impacts the surface of Ganymede at a relatively normal angle to the surface at speeds between 4 and 6 km s−1 (similar to the expected impact characteristics of the Europa Clipper spacecraft into Ganymede at disposal). As in the example given in Section 4.2, it will be assumed that this hypothetical impactor mission has a 95% chance of successfully performing its impact objective, whereupon it will potentially contaminate a single grid element (). There is no more than a 5% chance of some failure occurring that leads to an off-nominal impact event, where the number of Europa surface grid elements with a microorganism delivered to them is bounded above by
. The resurfacing rate will be taken to be
, the reciprocal of the midrange value for the average surface age discussed above, and we assume, as before, that there are 960 yr remaining in the period of biological exploration. With these inputs, we use Equation (10) to calculate
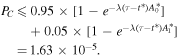
Hence, a conservative upper bound on the probability of contamination of the impactor mission described here is 1.63 × 10−5, well below the 1 × 10−4 requirement. Finally, we note that a similar evaluation can be performed for a similar impactor to Callisto, which would present a much lower contamination risk owing to its older surface age.
4.4. Application to a Hypothetical Enceladus Orbiter Mission Concept
When implementing this approach for the exploration of Enceladus, it is critical to note key differences between activity on Enceladus and activity on other ocean worlds. Unlike Europa, the surface age of Enceladus is regionally dependent. The age of cratered plains is 1.7–4.2 Gyr, the folded plains of Sarandib Planitia are 170 Myr–3.8 Gyr old, the ridged plains of Samarkand Sulcus are 10–980 Myr old, and the surface of the south polar terrain is 0.5–100 Myr old (Porco et al. 2006). This implies that Enceladus does not experience global "conveyor-belt" resurfacing, but rather ∼10 Myr of global resurfacing activity at intervals of 100 Myr–1 Gyr (O'Neill & Nimmo 2010). Additionally, we know that the south polar terrain of Enceladus is currently active, with a putative subsurface reservoir jetting water vapor plumes to space (Porco et al. 2006; Smith-Konter & Pappalardo 2008; Kite & Rubin 2016). The deposition of material from these water vapor plumes results in surface burial at a maximum rate of ∼0.1 mm yr−1 (Southworth et al. 2019). For a south polar ice shell thickness of ∼10 km (Hemingway et al. 2018), this results in a burial timescale of ∼100 Myr.
Therefore, applying the resurfacing model to a hypothetical Enceladus orbiter requires modification of some key assumptions. To start, no individual resurfacing rate will capture the present-day age of all terrains on Enceladus. Instead, an assumption of λ must be made that maximizes resurfacing and is conservative against the youngest observed ages. Additionally, given the likelihood of fresh surface deposits at the South Pole, where the minimum age is 0, some information about subsurface transport must be taken into account. Noting that the burial timescale of South Pole material is ∼100 Myr (Hemingway et al. 2018; Southworth et al. 2019) and that the minimum recurrence interval for large-scale catastrophic resurfacing is also ∼100 Myr (O'Neill & Nimmo 2010), we take the following approach: (1) we assume that resurfacing is global, with a resurfacing area equal to the surface area of the body, and (2) the resurfacing rate is nominally set at . This case differs from the previous example because λ is not strictly the reciprocal of the average surface age. Rather, it includes information regarding both resurfacing and subsurface transport. A more careful study application to Enceladus would consider the full range of applicable subsurface processes associated with material burial and transport.
As in the previous two examples, we assume the same bound on the time remaining in the period of biological exploration (960 yr) and that the event this hypothetical mission is successful is 95%. We also assume that the spacecraft is disposed into Enceladus within the period of biological exploration at the end of its nominal mission. Since we assume global resurfacing, the entire surface of Enceladus is treated as a single grid element. Therefore, unlike the previous examples, the number of Enceladus grid elements potentially contaminated under any scenario (nominal or off-nominal) is equal to 1, regardless of the impact dynamics. Therefore, an initial bound on the probability of contamination can be calculated as
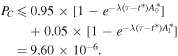
We note that in the practical application of this method to an orbital Enceladus mission, additional assurance would likely be required to demonstrate a low probability of encountering an active south polar fissure, where the surface age may be ∼0 (Porco et al. 2006). In such a scenario, the fissures and vents themselves may be treated similarly to Mars's special regions, where a separate planetary protection classification applies to the areas most sensitive to contamination and critical to the search for life (Rummel et al. 2014). A south polar lander would be similarly sensitive to vent contamination. Still, when landing at the south pole, we recommend application of the process described above. While the burial timescale due to water vapor fallout is constrained, the mechanisms that may trigger widespread geologic activity are not (O'Neill & Nimmo 2010), and the south polar terrain may be susceptible to additional modes of resurfacing.
5. Relaxing Conservatism
Layers of conservatism in a model can have significant consequences for a project attempting to demonstrate compliance with planetary protection requirements. This is exemplified by the "Worst Case" column of Table 1; piling worst case upon worst case, if not vetted further, could lead to a mission such as Europa Clipper not complying with planetary protection requirements. 5 Exposing the conservatism in the modeling gives assurance to decision makers that the planetary protection risk is as low as practically possible. Furthermore, judicious removal of conservatism can demonstrate the margin between the estimated probability of contamination and the requirement, which is typically part and parcel with demonstrating compliance with the requirement to stakeholders.
Consideration of surface terrain characteristics is one possible way of relaxing this conservatism in a geologically meaningful way and is further discussed in this section. We demonstrate this for Europa, which serves as a concrete example that can be carried over to other ocean worlds as well. Specifically, we discuss an area of conservatism in the model discussed earlier—the size of a grid element vulnerable to a resurfacing event—and how geologic diversity of surface characteristics can be represented in the model to relax some of this conservatism.
The probability that a region of the Europan surface hosting a microorganism resurfaces (given contact with the surface has occurred) may change with geologic context and for different amounts of time remaining in the period of biological exploration. Figure 6 shows a sensitivity of the conditional probability that a resurfacing event occurs given by Equation (7) when the time remaining in the period of biological exploration (horizontal axis) is varied. Level curves show this relationship for various grid element size assumptions, each identifiable with currently known Europa surface features. This figure assumes yr−1 (the most conservative resurfacing rate) and Am
(u) = 1000. Note that level curves are almost indistinguishable once the grid element size is greater than 1000 km2.
Figure 6. Sensitivity of the conditional probability of resurfacing as calculated by Equation (7) when the time remaining in the period of biological exploration is varied. Values for λ and Am
(u) are fixed at yr−1 and 1000, respectively. Level curves show this sensitivity for various grid element size assumptions. Each grid element size can be related to various Europa landforms indicative of surface modification (right pictures). For planetary protection modeling, a smaller grid element size implies a higher chance of resurfacing and therefore a higher probability of contamination (hence, the current model assumption of 1 km2 grid elements is conservative). Moreover, the longer the time remaining in the period of biological exploration, the greater time a resurfacing event has to take place, resulting in a higher probability of contamination.
Download figure:
Standard image High-resolution imageA first observation is that, given enough time, the model will allow any region to resurface with probability approaching unity. Hence, a finite period of biological exploration is needed in order to realize a significant reduction in the probability of contamination. As previously stated, the NASA Office of Planetary Protection set the bound on the period of biological exploration, τ, equal to the calendar year 3000 for Europa Clipper.
Of geologic interest is that, holding the time remaining in the period of biological exploration fixed, the probability that a region resurfaces decreases as the size of the region increases. This is because the average surface age must be maintained at a value of . The landforms on the surface of Europa vary greatly in size (e.g., Doggett et al. 2009), significantly affecting the probability of a resurfacing event (see Table 2). Ridges cover areas 101–104 km2 in size (e.g., Doggett et al. 2009; Prockter & Patterson 2009), chaos terrain can vary from 103 to 106 km2 in size (e.g., Collins & Nimmo 2009), extensional bands and convergent margins range 104–106 km2 in size (e.g., Prockter & Patterson 2009; Kattenhorn & Prockter 2014), and large impact events affect regions 102–104 km2 in size (e.g., Schenk & Turtle 2009).
Table 2. Sensitivity of the Probability a Resurfacing Event Occurs (τ − tm
(u) = 1000 [yr], yr−1, Am
(u) = 1000) According to Equation (7) Based on Different Landform Assumptions and Grid Element Sizes
Landform Type | Grid Element Size (km2) | Probability of Resurfacing Given Impact |
---|---|---|
Current conservative assumption | 1 | 1.53 × 10−2 |
Lenticulae, microchaos | <100 | <1.54 × 10−4 |
Ridges | 10–10,000 | 1.54 × 10−3–1.54 × 10−5 |
Large impacts | 100–10,000 | 1.54 × 10−4–1.54 × 10−5 |
Chaos | 1000–1,000,000 | 1.54 × 10−5 |
Bands | 10,000–1,000,000 | 1.54 × 10−5 |
Catastrophic resurfacing | 31,000,000 | 1.54 × 10−5 |
Download table as: ASCIITypeset image
A physical upper bound for the affected area is that of global, catastrophic resurfacing, where the entire surface of Europa (≈3.1 × 107 km2) is treated as one grid element. Table 2 summarizes the probability of resurfacing given by Equation (7) for τ − tm (u) = 1000 (i.e., time remaining in the period of biological exploration is 1000 yr after contact with Europa). Note that when the area spanned by Am (u) grid elements is less than or equal to the landform size, there is no change in the resurfacing probability since the region covered by Am (u) is assumed to be entirely in one resurfacing region for this example.
A future study may consider the local geological context of the region of planetary protection interest, such as an unintentional impact site or landing site. As mentioned previously, not all geologic landforms on Europa are thought to be active today, nor are all landforms thought to involve shallow subsurface water. Additionally, the majority of landforms are orders of magnitude larger in size than the 1 km2 active resurfacing area assumed here. Therefore, future studies can relax model conservatism by increasing the minimum resurfacing area, decreasing the fraction of surface thought to directly participate in surface interactions with liquid reservoirs, and applying a feature-appropriate resurfacing rate (surface features indicative of processes that enable downward transport are rare; e.g., Kattenhorn & Prockter 2014).
To implement this less conservative approach, it must be known where debris and ejecta (potentially hosting microorganisms delivered by the spacecraft) reside on the surface after impact or contact, particularly for oblique (shallow angle) impacts. While the number of grid elements can be reasonably bounded by analysis and experiment, reliably determining the trajectory and final destination of debris is much more difficult and beyond the scope of this study.
6. Conclusions
Missions to ocean worlds must currently demonstrate that the probability of contaminating liquid water environments within the period of biological exploration is less than 1 × 10−4. The model introduced in this paper leverages the geologic understanding of the current or recent resurfacing of Europa to conservatively demonstrate paths to compliance with this requirement for Europa Clipper, as well as future exploration missions to Europa and ocean worlds other than Europa. Highlighting the important role of geophysical processes in distributing material through space and time, this model can also greatly help to bound the probability of contamination for missions early in their development life cycle, thereby influencing the engineering architecture to proactively address planetary protection concerns well ahead of final design decisions. While this model does not consider timescales of transport through the icy subsurface, future work in this area will act to relax the conservatism assumed here to provide more realistic bounds on the probability of contamination.
We identify the following items as the crucial constraints that affect the potential of geologically active ocean worlds to facilitate subsurface transfer and biological contamination:
- 1.The average age of the surface, applied either globally or regionally, as it inversely controls the resurfacing rate.
- 2.The minimum size of a region that may spontaneously undergo geologic activity and surface modification, as this controls the probability that contaminants on the body's surface will overlap with a region of active geologic change.
- 3.The rate and depth to which various applicable processes may transport material to potentially habitable subsurface environments, as these can be used to relax conservative assumptions to meet requirements.
- 4.The amount of surface that is potentially contaminated by the space mission upon contact with the ocean world, in both nominal and off-nominal scenarios.
As future missions pursue more and more ambitious investigations of these worlds, including the potential fly-through of water vapor plumes, in situ exploration of their surfaces (Hand et al. 2017; MacKenzie et al. 2020), and deep subsurface exploration of their ice and oceans (Howell et al. 2021b), this requirement will continue to be a challenging constraint to consider. Because this model can be implemented conservatively with limited information about the body, this approach may be especially suitable for considering missions to potentially habitable, active ocean worlds and potential ocean worlds that are poorly observed, such as Neptune's moon Triton or the Uranian satellites.
The authors would like to acknowledge B. Pappalardo and B. Schmidt for their guidance and insight in considering geologic constraints applied to planetary protection, E. Leonard for her help in providing and interpreting the Europa geologic map, and G. Collins and S. Kattenhorn for providing feedback on this approach and paths toward relaxing conservatism in the Europa Clipper planetary protection review process. The authors also acknowledge the contributions of the NASA Office of Planetary Protection and the broader 2018 November Planetary Protection Workshop Panel for their review and critique of this work. This work was supported by NASA through the Europa Clipper project. The research was carried out at the Jet Propulsion Laboratory, California Institute of Technology, under a contract with the National Aeronautics and Space Administration (80NM0018D0004). ©2021. California Institute of Technology. Government sponsorship acknowledged.
Appendix A: Notation
- •αE: The average surface age of Europa.
- •a0: The initial age of a surface grid element of an ocean world at the start of resurfacing processes.
- •Am : An upper bound on the number of grid elements of the ocean world's surface with a microorganism delivered by the spacecraft, when the spacecraft completes its nominal mission and is on trajectory m.
- •Am (u): An upper bound on the number of grid elements of the ocean world's surface with a microorganism delivered by the spacecraft, when the spacecraft experiences a failure at time u and is on trajectory m.
- •CBE: Current best estimate.
- •cy: Calendar year.
- •E0: The event that the nominal mission is successfully completed as planned and the spacecraft makes contact with the ocean world.
- •E1: The event that some off-nominal event leading to contact with the ocean world occurs over the course of the mission owing to a spacecraft failure.
- •E1(u): The event that an off-nominal event leading to the spacecraft making contact with the ocean world occurs owing to a failure at time u.
- •f1(u): The probability density function associated with the event E1(u).
- •G: The number of grid elements partitioning the ocean world's surface that are vulnerable to a resurfacing event.
- •Gyr: Billions of years.
- •kyr: Thousands of years.
- •λ: The resurfacing rate of a given ocean world.
- •M: The number of trajectories simulated to evaluate the probability of contact and associated impact/contact characteristics (e.g., impact speed, obliquity) associated with the nominal mission.
- •Mu : The number of trajectories simulated to evaluate the probability of contact and associated impact/contact characteristics (e.g., impact speed, obliquity) associated with off-nominal events where a failure occurs at time u.
- •Myr: Millions of years.
- •PC : The probability of contamination as defined by the events defined in this study. Specifically, this is the probability that one or more terrestrial microorganisms come in contact with the ocean world's subsurface and remain viable.
- •Pr: Denotes a measure of probability.
- •Prk (t): The probability that a given grid element of the ocean world undergoes its kth resurfacing event by time t.
- •Rτ : The event that a microorganism, originally on board the spacecraft, resides on an area of the ocean world that resurfaces within the period of biological exploration defined by τ.
- •S: The event that one or more microorganisms launched with the spacecraft survive to come in contact with the ocean world's subsurface.
- •τ: The calendar year marking the end of the period of biological exploration.
- •tm : The time at which the spacecraft comes in contact with the ocean world along trajectory m when the nominal mission occurs.
- •tm (u): The time at which the spacecraft comes in contact with the ocean world along trajectory m when a spacecraft failure occurs at time u.
- •u: A variable used to denote the time at which a spacecraft failure occurs. The types of failures considered here are those that lead to off-nominal mission outcomes, such as a loss of maneuverability or the ability to complete some other critical event (such as separation of two flight elements).
Appendix B: Monte Carlo Simulation of the Resurfacing Model
The Monte Carlo MATLAB code used to illustrate the application of resurfacing to Europa in Figure 3 is included with associated input files as Data behind the Figure. The MATLAB script resurfacingMC. m shows the evolution of Europa's surface over time (tmax), evaluated at each time step (dt), and maintaining an average surface age (age) of 20 Myr.
The model partitions Europa into equal-area regions and assumes a probability of resurfacing based on the resurfacing rate λ, as described in Section 2. The background map of Europa is a Mollweide projection of the Europa geologic map (Leonard et al. 2018b). Grid lines represent regions of equal area set in the input parameters. As regions become geologically active, a transparent orange layer is applied to show activity.
The model results are output as an MPEG-4 video file (Figure 3).
Appendix C: Additional Mathematical Discussion Regarding the Average Surface Age of an Ocean World
This appendix provides a mathematical derivation of expression (3) describing the average surface age of regions of a given ocean world's surface.
In what follows, Tn is the time of the nth resurfacing event, where tn = Tn − Tn−1 is a random variable representing the time between resurfacing events n and n − 1. Here n > 0 and T0 ≡ 0 is a convention representing the time at which the latest resurfacing process of rate λ started. Given the resurfacing model defined by Equation (2), tn are independent and identically distributed times between resurfacing events, each following an exponential distribution with rate λ, i.e., tn ∼ Exponential(λ). Let Ai (t) be a random variable representing the observed age of grid element i of an ocean world at time t, where i = 1,...,G. The age of grid element i at time 0 is assumed to be ai,0. The average age of the ocean world's surface when observed at time t is denoted by A(t) and is calculated as a weighted average of each grid element's age based on its surface area, with expected value
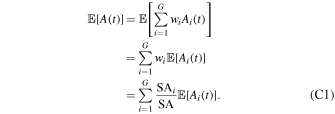
In Equation (C1), is the expected value function and wi
is a weighting factor associated with the age of each grid element, estimated here based on the surface area of grid element i, SAi
, and the total surface area of the ocean world, SA.
Letting N(t) denote the number of resurfacing events that have occurred by time t,
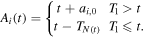
Therefore, using the law of total expectation,
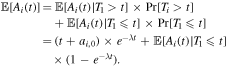
Note that, when T1 ≤ t, it follows that t − T1 ≤ t − TN(t) ≤ t. Thus, to calculate in the above equation, we apply the law of conditional expectation again and integrate according to values of T1 = u, where u goes from 0 to t:
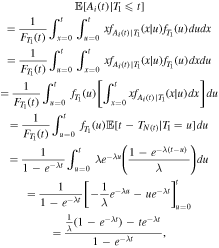
where is the probability density function of
conditional on T1,
is the probability density function of T1, and
is the cumulative distribution function of T1 evaluated at time t. The first equality above follows by the definition of conditional expectation and properties of conditional probability.
6
The second equality, which switches the order of integration, follows by the Fubini–Tonelli theorem and continuity of the functions under the integral. The third equality brings the function
to the outside of the integral since it does not depend on x. The fourth equality again follows from the definition of conditional expectation. The sixth and seventh (last two) equalities solve the outer integral.
In the fifth equality we have used the result for the expected age when the process starts at time u and time between events is exponentially distributed. The time duration t − TN(t) follows an exponential distribution with rate λ but can only take values on the interval . Therefore, the expected time since the previous resurfacing event, conditional on the time of the first resurfacing event occurring at time u, can be calculated as
.
Hence, the expected age of grid element i of the ocean world is
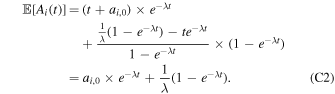
Note that when , Equation (C2) simplifies to
. Therefore, when the resurfacing rate is the reciprocal of the initial age of the grid element, the resurfacing rate is not a time-dependent function of the average surface age.
Moreover, for equal-area grid elements of an ocean world, Equation (C1) becomes
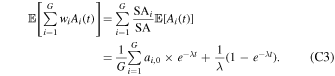
Finally, when all grid elements are assumed to have the same age at the beginning of the resurfacing processes, a0, Equation (C3) simplifies to
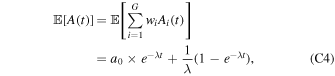
as shown in expression (3).
Footnotes
- 1
The period of biological exploration refers to the time necessary for robotic missions to determine whether biological systems occur on a potentially habitable planetary body (NRC 2012). This was enacted by the NASA Office of Planetary Protection (OPP) in 2019 via a Memorandum of Understanding between the Jet Propulsion Laboratory and NASA OPP.
- 2
- 3
If the average observed age of Europa is not relatively constant over significant timescales, then λ values higher than
yr−1 are at least mathematically possible.
- 4
This is documented in a Memorandum of Understanding between the Jet Propulsion Laboratory and NASA OPP, "Europa Clipper Disposal Targets," dated 2021 June 30.
- 5
See McCoy et al. (2020) for further discussion as to how Europa Clipper demonstrated compliance with planetary protection requirements.
- 6
Specifically, we use the result that, for any event, A, and any set of mutually exclusive nonempty events Bj , j an integer,
, where
.