Abstract
Methyl isocyanate (CH3NCO) is a recently identified interstellar molecule giving rise to many detected lines. Interestingly, its delayed identification was due not to weak lines but to a very complex rotational spectrum. To date, the only published laboratory transitions for this molecule are those between rotational energy levels with K ≤ 3. In the present work, Stark-modulation spectroscopy was used to record the room-temperature rotational spectrum of CH3NCO in the spectral region from 32 to 90 GHz. Observation of characteristic Stark effects, measured at specific low-voltage modulation conditions, and 14N nuclear quadrupole hyperfine structure allowed unambiguous assignment of rotational transitions up to K = 10. These newly assigned transitions were subsequently followed up to 364 GHz with the aid of Loomis–Wood-type displays. Since there are no reports on astrophysical detection of 13C isotopic species, first laboratory measurements between 50 and 300 GHz have also been performed for CH3N13CO and 13CH3NCO isotopologues. A comprehensive spectral analysis undertaken in this work made it possible to extend the knowledge of the rotational spectrum of CH3NCO to more than 2500 new transitions. Furthermore, more than 1200 lines were identified and analyzed for each of the isotopologues. The extensive line lists and sets of molecular parameters reported in this work provide the basis for further astrophysical searches of CH3NCO.
Export citation and abstract BibTeX RIS
1. Introduction
The reported detection of methyl isocyanate (CH3NCO) on the frozen surface of comet 67P/Churyumov–Gerasimenko (Goesmann et al. 2015) provided the impulse for its detection in the interstellar medium (ISM). Even though that cometary identification is nowadays called into question (Altwegg et al. 2017), CH3NCO is now considered to be a well-established interstellar molecule, with the first reported detections toward Sgr B2(N) (Halfen et al. 2015) and Orion KL (Cernicharo et al. 2016) molecular clouds. Later on, Belloche et al. (2017) found some additional transitions in hot molecular core Sgr B2(N2). Recently, CH3NCO has also been observed in the low-mass solar-type protostar IRAS 16293–2422 (Ligterink et al. 2017; Martin-Domenech et al. 2017). Relying on these detections, several laboratory experiments have been undertaken in order to unveil the astrochemical origin (Ligterink et al. 2017, 2018) and survival probability of CH3NCO in interstellar and cometary ices (Maté et al. 2018). Finally, CH3NCO has also been incorporated into astrochemical models (Belloche et al. 2017; Martin-Domenech et al. 2017; Majumdar et al. 2018; Quénard et al. 2018).
Inspection of the current lists of detected transitions reveals that the most significant number of CH3NCO lines has been found in the millimeter-wave survey of Orion KL (Cernicharo et al. 2016). However, all of those 399 lines belong to K ≤ 3 transitions, simply because confident assignment of K > 3 transitions was not available at the time. Furthermore, astrophysical detection of 13C isotopic species could not be attempted, also for lack of suitable laboratory data. The identification of 13C isotopologues of detected molecules is of special importance in astrophysics since it provides information on abundance ratio of 12C over 13C isotopes in the ISM. The abundance of CH3NCO in Orion KL has been found to be only a factor of 10 below those of HNCO and CH3CN (Cernicharo et al. 2016), which makes this molecule a significant potential contributor to so-called U-lines. The large number of 8000 U-lines out of 16,000 lines detected in the line survey of Orion KL (Tercero et al. 2010) was reduced on successive investigations, yet CH3NCO accounted for the strongest outstanding U-lines at the time of its detection (Cernicharo et al. 2016). Hence, K > 3 transitions of the parent species and transitions of the 13C isotopic species are also expected to be good candidates for future detections. The fact that there were no attempts to detect these lines is due to the lack of suitable laboratory data arising largely from the difficulties in dealing with the rotational spectrum of the CH3NCO molecule.
The pioneer microwave measurements performed by Curl et al. (1963), Lett & Flygare (1967), and later by Koput (1984, 1986, 1988) have demonstrated that CH3NCO displays a spectrum with practically irregular distribution of a-type R-branch transitions of comparable intensities. This spectral complexity has been attributed to the presence of two large-amplitude motions in the molecule: the internal rotation of the methyl group with a very low barrier (V3 = 21 cm−1) and a low-frequency CNC bending motion (νb). A detailed analysis carried out by Koput (1986) made it possible to unambiguously assign the rotational transitions in numerous low-lying internal rotation states (described by the quantum number m) in the ground state () and the two lowest excited states of the CNC bending mode (
). The quantum number assignment was based on characteristic line patterns observed in the Stark-modulation spectrum. That work served as an excellent starting point for the sequence of laboratory studies (Kisiel et al. 2010, 2015; Cernicharo et al. 2016) in which the original assignment made by Koput (J'' ≤ 3, K ≤ 3) was extended up to 364 GHz (J'' = 41). This resulted in a list of 1269 confidently assigned laboratory lines and detection of CH3NCO toward Orion KL (Cernicharo et al. 2016).
The present work has been conducted in two different directions: on the one hand, to extend the knowledge of the laboratory rotational spectrum of parent CH3NCO, and on the other hand, to provide first spectroscopic information on the CH3N13CO and 13CH3NCO isotopic species. A combination of microwave Stark-modulation and frequency-modulation millimeter-wave spectroscopy was used for this purpose. The observation of characteristic Stark effects measured at specific low-voltage conditions, specific nuclear quadrupole hyperfine structure, and the use of a graphical Loomis–Wood-type plot approach was fundamental for assignment of rotational transitions. An extensive set of new laboratory lines for the parent species and the two 13C isotopic species is provided in this work. This should facilitate further identifications of CH3NCO in space.
2. Experimental Details
2.1. Chemical Synthesis
The parent species of CH3NCO has been prepared as previously reported (Maté et al. 2017). The syntheses of Han et al. (1989) have been modified to prepare CH3N13CO and 13CH3NCO.
CH3N13CO: Sodium azide (1.63 g, 25 mmol, 2 equiv.) and dry diethylene glycol dibutyl ether (15 ml) were introduced into a three-necked flask equipped with a nitrogen inlet, a reflux condenser, a magnetic stirring bar, and a dropping funnel. The suspension was heated at 80°C–95°C, and acetyl-1-13C chloride (1g, 13 mmol, 1 equiv.) was added dropwise. The suspension was stirred at this temperature for 1 hr. After cooling to room temperature, the flask was connected to a vacuum line (0.1 mbar) equipped with two traps. The first one was immersed in a bath cooled at –30°C to remove high-boiling compounds. The low-boiling CH3N13CO was selectively condensed in the second trap immersed in a liquid nitrogen bath. Yield: 392 mg, 52%. 1H NMR (CDCl3, 400 MHz) δ 3.02 (d, 3JCH = 4.9 Hz, CH3). 13C NMR (CDCl3, 100 MHz) δ 28.1 (d, 2JCC = 5.1 Hz, CH3), 121.6 (s brd, NCO).
13CH3NCO: Silver cyanate (2g, 13.3 mmol), dry diethylene glycol dibutyl ether (3 mL), and iodomethane-13C (1g, 7.0 mmol) were mixed together in a cell equipped with a magnetic stirring bar and a stopcock. The cell was immersed in a liquid nitrogen bath and degassed. The mixture was heated under stirring at 90°C for 20 hr. The cell was then connected to a vacuum line (0.1 mbar) equipped with two traps. Following the same procedure as mentioned above, low-boiling 13CH3NCO was selectively condensed in the second trap immersed in a liquid nitrogen bath. Yield: 243 mg (60%). 1H NMR (CDCl3, 400 MHz) δ 3.01 (d, 1JCH = 143.1 Hz, CH3). 13C NMR (CDCl3, 100 MHz) δ 28.1 (d, 1JCH = 143.1 Hz, 13CH3), 121.4 (s brd, NCO).
2.2. Rotational Spectra
A Stark-modulation spectrometer, whose design has been described previously (Kolesniková et al. 2017), was used to acquire the Stark spectra of the parent and 13C isotopic species between 32 and 90 GHz. To cover this region, an Agilent synthesizer (≤20 GHz) was used in combination with an additional frequency doubler, tripler, or quadrupler as a frequency source. The absorption lines were modulated with a 33.3 kHz zero-based square-wave voltage, and phase-sensitive detection by means of a lock-in amplifier was employed. Modulation voltages of 10 and 150 V, corresponding to electric field strengths of 21 and 319 V cm−1, were applied.
Frequency-modulation spectra of the 13C isotopic species above 50 GHz were recorded using the millimeter and submillimeter-wave spectrometer in Valladolid, which has been described in Daly et al. (2014). Agilent synthesizer and a set of amplifier-multiplier chains WR15.0, WR10.0, WR6.5, and WR9.0 (VDI, Inc) in combination with an additional frequency doubler and tripler (WR4.3, WR2.8, VDI, Inc.) were used to reach frequencies up to 300 GHz. The synthesizer frequency was modulated at f = 10.2 kHz, with modulation depth ranging from 30 to 40 kHz. A detection system composed of either Schottky diodes or broadband quasi-optical detector (VDI, Inc) was completed by a 2f demodulation using a phase-sensitive lock-in amplifier. All spectra were recorded in 1 GHz sections in both directions (a single acquisition cycle) and averaged. The archival data in the 117–364 GHz frequency region (Cernicharo et al. 2016) recorded with the Fast Scanning Submillimeter Spectroscopic Technique (FASSST; Petkie et al. 1997; Medvedev et al. 2004) have also been used for the parent species and for some highest-frequency lines of the 13C species visible in natural abundance. The spectra from the various spectrometers were combined into a single spectrum and further processed using the AABS package (Kisiel et al. 2005, 2012). The uncertainty of center frequency measurement on the recorded second-derivative line shapes was estimated to be better than 50 kHz. In the experiments, the samples of CH3NCO were kept at room temperature, typically at a pressure of 20 μbar (15 mTorr).
3. Analysis and Results
3.1. Parent Species
Considerable progress in the assignment of higher-K transitions was made upon measurement of the Stark spectrum of CH3NCO at the low modulation voltage of 10 V. We were able to build on the classification of Stark patterns made by Koput (1984). The frequency spread of the expected Stark pattern for this near-prolate molecule is expected to be directly proportional to K. At low modulation voltage, K = 0 transitions disappear, while the target K > 3 transitions should give rise to Stark patterns with the broadest frequency spans and in the form of equidistant combs of resolved Stark components. Under the phase detection used in the present Stark-modulation method, these have the form of negative patterns of Stark lines on both sides of the field-free line, which is displayed in the positive direction. A portion of the spectrum in the region of the rotational transition is shown in Figure 1. This part of the spectrum is removed from the clutter
lines for different internal rotation m states and different CCN bending mode vb states centered on 43,600 MHz. Inspection of isolated, already-assigned lines in this spectrum showed relative invariance of the Stark patterns on m and vb, as visible in Figure 1. Comparison of the visible line patterns allowed confident identification of the line at 42,731 MHz in Figure 1 as corresponding to K = 4.
Figure 1. Top panel: CH3NCO molecule and a section of the low-voltage, Stark-modulation spectrum (10 V) in the region of the rotational transition. Bottom panel and inset: detail of the spectrum showing a characteristic Stark effect and line shape asymmetry for isolated transitions. While K = 1 and K = 2 transitions are not well modulated, clear fast first-order Stark lobes (see the text) can be observed for the K = 3 transitions, and the increase of the Stark lobe span with K allows easy identification of the K = 4 transition. Furthermore, the line shape of the K = 4 transition is affected by the nuclear quadrupole hyperfine structure, which is in agreement with predictions in Figure 2.
Download figure:
Standard image High-resolution imageAnother useful feature, observed in the lowest-J rotational spectra of CH3NCO, is the nuclear quadrupole hyperfine structure due to the presence in the molecule of a single 14N nucleus. At the current resolution of the Stark spectra in Figure 1, this gives rise to a noticeable asymmetry in the line shapes for K = J'' transitions (as for the K = 4 line in Figure 1). As shown in Figure 2, these doublets can be better resolved in the frequency-modulated spectra not only for K = J'' but also for K = J'' − 1 transitions. The frequency splitting in the doublet is measurable and is compared in Figure 2 with predictions based on the most recent hyperfine splitting constants for CH3NCO (Cernicharo et al. 2016). The hyperfine patterns thus provide independent confirmation of the assignments based on the Stark patterns. The behavior of the Stark and hyperfine patterns as a function of K and J'' for lines that are crucial to the higher-K assignment is further illustrated in Figure 3.
Figure 2. Line shapes for the higher-K transitions at the lowest possible values of J'' illustrating the characteristic, asymmetric doubling arising from the partially resolved nuclear quadrupole hyperfine structure. (a) Comparison of the resolution in Stark and frequency-modulated (FM) spectra. (b) Illustration of the relatively constant, resolvable doubling visible in FM spectra for the J'' = K lines, which decreases rapidly for J'' = K + 1 transitions. (c) Calculated values of the splitting to compare with the experimental values indicated in the previous panel.
Download figure:
Standard image High-resolution imageFigure 3. Diagram of Stark line shapes for some K = J'', K = J'' − 1, and K = J'' − 2 transitions showing the increasing frequency span of the Stark lobe pattern on increasing K for a given value of J'', while for a given K the width of the Stark pattern and the hyperfine splitting decrease rapidly with increasing J''.
Download figure:
Standard image High-resolution imageThe application of these criteria and step-by-step inspection of spectral regions corresponding to higher-J transitions then allowed the identifications of K = 5–10 transitions. The availability of two different criteria was especially useful in the denser portions of the spectra, where the characteristic Stark lobes could not be clearly distinguished owing to overlaps with those of neighboring lines. Final confirmation of the K assignments was made simply by the absence of the corresponding lines in the spectral region for J < K. Once the K > 3 transitions were successfully assigned in the low-frequency Stark spectra for lowest J, their higher-J counterparts could be easily located in the higher-frequency spectra (up to 364 GHz) measured in our previous work (Cernicharo et al. 2016). Graphical Loomis–Wood-type plotting techniques as built into the AABS package (Kisiel et al. 2005, 2012) proved invaluable for this purpose. The J dependence of frequencies for a given set of K, m, and vb quantum number values is a smooth function so that such transition sequences are readily identifiable.
Koput (1986) has shown that the global fitting of the rotational spectrum of CH3NCO to experimental accuracy is an extremely challenging task. For this reason, the experimental spectrum has been broken down in our previous work into more than 220 (K, m, ) sequences of correlated lines, with each sequence analyzed separately (see Cernicharo et al. 2016, for details). The same pragmatic approach is employed in this work. Each of the identified K > 3 sequences was thus fitted to a suitable linear-rotor-type J(J + 1) power series expansion for the rotational energies
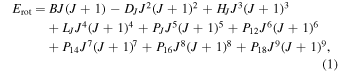
where the lengths of the centrifugal distortion expansions were established empirically. In cases of partially resolved nuclear quadrupole hyperfine structure, such as those in Figure 2, an intensity-weighed frequency average has been the measured frequency used in the fitting procedure. The determined linear-rotor-type parameters are listed in Table 1, while the experimental frequencies are collected in Table 2. It has to be noted that while the assignment of the K quantum numbers is confident, the assignment of m quantum numbers is rather uncertain, although a general decrease of intensity with increasing m may be expected. For this reason in Table 1 we list the sequences in order of decreasing intensity for each K and adopt a modified naming convention in relation to that used in Cernicharo et al. (2016). For example, for K = 4 transitions, the labeling of corresponding sequences is as follows: K4a, K4b, K4c, etc., where a, b, c are assigned in the order of decreasing relative intensity at room temperature (a strongest, b second strongest, etc.). Many sequences for K = 4 and 5 consist of degenerate lower-J parts, separating into two distinct series at high J. In such cases L and U letters are added (e.g., K4aL, K4aU) in order to distinguish between the components separating to lower and upper frequency, respectively. In some cases a line sequence displayed a J dependence of transition frequencies that was smooth but sufficiently nonlinear to fall outside the tractability as a whole with Equation (1). This necessitated splitting the sequence into two parts (with prefixes V and X), such as for the strongest K = 4 sequence in Table 1 reproduced by parts V129 and X129.
Table 1. Parameters of the Linear-rotor-type, J(J + 1) Power Series Fitted to the Assigned K > 3 Line Sequences of the Parent Species
Ka | Nameb | Irelc | Nind | Nreje | σwf | Bg (MHz) | DJ (kHz) | HJ (Hz) | LJ (mHz) | PJ (μHz) | P12 (nHz) | P14 (pHz) | P16 (fHz) | P18 (aHz) |
---|---|---|---|---|---|---|---|---|---|---|---|---|---|---|
K = 4 | ||||||||||||||
K4aL | V129: | 10 | 0 | 3.159 | 3830.2(86) | −8.74(32) × 103 | −1.295(64) × 105 | 1.287(81) × 106 | −8.59(65) × 106 | 3.79(33) × 107 | −1.05(10) × 108 | 1.69(19) × 108 | −1.17(14) × 108 | |
X129: | 0.758 | 27 | 0 | 4.944 | 4200.20(73) | −121.8(37) | −305.(10) | 404.(18) | -343.(19) | 194.(13) | −70.0(58) | 14.6(13) | −1.34(14) | |
K4aU | V130: | 10 | 0 | 3.764 | 3834.(10) | −8.62(38) × 103 | −1.269(77) × 105 | 1.255(96) × 106 | −8.33(77) × 106 | 3.66(39) × 107 | −1.02(12) × 108 | 1.62(22) × 108 | −1.12(17) × 108 | |
X130: | 0.759 | 27 | 0 | 4.904 | 4200.15(72) | −122.1(37) | −306.(10) | 405.(18) | -345.(19) | 195.(13) | −70.4(58) | 14.7(13) | −1.35(14) | |
K4bL | V102: | 9 | 0 | 0.0 | 4746.97 | 24.017 × 103 | 6.163 × 105 | −11.571 × 106 | 131.94 × 106 | −97.67 × 107 | 45.165 × 108 | −1.1835 × 1010 | 1.34 × 1010 | |
X102: | 0.734 | 29 | 0 | 2.597 | 4232.88 (10) | −7.18 (67) | 18.5 (23) | −40.4 (47) | 46.3 (60) | −32.9 (46) | 14.4 (22) | −3.53(59) | 0.373(66) | |
K4bU | V103: | 9 | 0 | 0.254 | 4746.93 ) | 24.014 × 103 | 6.152 × 105 | −11.568 × 106 | 131.91 × 106 | −97.63 × 107 | 45.145 × 108 | −1.1829 × 1010 | 1.34 × 1010 | |
X103: | 0.737 | 29 | 0 | 1.349 | 4232.152(74) | −11.43(46) | 5.7 (15) | −16.9 (30) | 19.2 (36) | −13.2 (28) | 5.6 (13) | −1.36(34) | 0.141(38) | |
K4c | V004: | 0.727 | 29 | 6 | 0.869 | 4346.5608(20) | 1.536(10) | 0.044(22) | −0.085(20) | 0.0428(67) | ⋯ | ⋯ | ⋯ | ⋯ |
K4dL | V141: | 0.694 | 37 | 0 | 1.304 | 4405.4265(92) | 41.59(12) | 61.43(83) | −84.5(30) | 96.7(62) | −81.1(79) | 45.0(59) | −14.7(24) | 2.12(42) |
K4dU | V140: | 0.714 | 37 | 0 | 0.914 | 4405.4441(68) | 41.936(98) | 64.27(67) | −96.4(25) | 124.3(55) | −118.0(74) | 73.7(58) | −26.7(25) | 4.23(46) |
K4e | V051: | 0.547 | 37 | 0 | 1.228 | 4389.1854(20) | 2.0690(67) | −0.1645(92) | 0.1082(55) | −0.0216(12) | ⋯ | ⋯ | ⋯ | ⋯ |
K4f | V108: | 0.493 | 30 | 5 | 0.947 | 4365.1008(16) | 1.9297(57) | −0.0254(79) | 0.0123(47) | −0.0031(10) | ⋯ | ⋯ | ⋯ | ⋯ |
K = 5 | ||||||||||||||
K5a | V022: | 0.988 | 33 | 3 | 1.016 | 4365.4070(12) | 2.2359(26) | 0.0252(21) | −0.00129(56) | ⋯ | ⋯ | ⋯ | ⋯ | ⋯ |
K5bL | V109: | 0.714 | 31 | 4 | 1.192 | 4306.3355(75) | −43.955(76) | −57.56(37) | 81.49(96) | −89.8(15) | 73.7(14) | −39.45(76) | 11.69(23) | −1.438(29) |
K5bU | V110: | 0.701 | 31 | 3 | 0.932 | 4306.3197(62) | −44.176(67) | −58.88(34) | 85.54(96) | −96.9(16) | 81.2(15) | −43.93(90) | 13.11(28) | −1.623(38) |
K5c | V001: | 0.676 | 35 | 0 | 1.240 | 4350.4222(15) | 1.4947(31) | 0.0136(25) | 0.00635(66) | ⋯ | ⋯ | ⋯ | ⋯ | ⋯ |
K5d | V092: | 0.573 | 36 | 1 | 1.030 | 4341.4381(58) | 6.558(55) | −2.24(25) | 1.08(61) | −4.27(89) | 5.22(78) | −3.97(40) | 1.58(11) | −0.239(13) |
K5e | V062: | 0.441 | 34 | 0 | 1.260 | 4399.9696(15) | 2.2318(32) | −0.0084(25) | −0.00105(68) | ⋯ | ⋯ | ⋯ | ⋯ | ⋯ |
K5f | V049: | 0.427 | 26 | 1 | 1.282 | 4387.7577(25) | 2.0469(83) | −0.085(12) | 0.0435(70) | −0.0082(15) | ⋯ | ⋯ | ⋯ | ⋯ |
K5g | V122: | 0.336 | 29 | 0 | 1.201 | 4355.8543(52) | −2.796(35) | 1.06(11) | 1.02(17) | 0.73(15) | −0.998(64) | 0.226(11) | ⋯ | ⋯ |
K5h | V087: | 0.269 | 32 | 1 | 0.993 | 4354.3392(12) | 1.6359(25) | −0.0057(21) | 0.00323(56) | ⋯ | ⋯ | ⋯ | ⋯ | ⋯ |
K5iL | V238: | 0.179 | 29 | 0 | 0.833 | 4356.1661(32) | −0.481(20) | 0.319(55) | 0.698(80) | −0.082(63) | −0.097(25) | 0.0204(40) | ⋯ | ⋯ |
K5iU | V239: | 0.172 | 30 | 0 | 0.916 | 4356.1725(35) | −0.424(22) | 0.525(62) | 0.350(89) | 0.210(68) | −0.213(27) | 0.0382(42) | ⋯ | ⋯ |
K = 6 | ||||||||||||||
K6a | V009: | 1.000 | 32 | 3 | 1.291 | 4353.4867(16) | 0.5959(33) | −0.0358(26) | 0.02590(70) | ⋯ | ⋯ | ⋯ | ⋯ | ⋯ |
K6b | V036: | 0.834 | 34 | 0 | 0.849 | 4374.6209(10) | 2.0963(21) | 0.0021(17) | 0.00123(45) | ⋯ | ⋯ | ⋯ | ⋯ | ⋯ |
K6c | V011: | 0.592 | 32 | 2 | 0.874 | 4355.09118(70) | 1.89726(79) | −0.00678(26) | ⋯ | ⋯ | ⋯ | ⋯ | ⋯ | |
K6d | V003: | 0.507 | 34 | 0 | 1.036 | 4347.7439(26) | 0.378(12) | 0.335(25) | −0.152(25) | −0.110(11) | 0.0335(21) | ⋯ | ⋯ | ⋯ |
K6e | V233: | 0.492 | 27 | 0 | 0.967 | 4412.075(14) | −5.10(20) | 13.6(13) | 9.3(52) | 88.(11) | −286.(16) | 311.(13) | −152.0(59) | 28.8(11) |
K6f | V048: | 0.344 | 31 | 2 | 0.942 | 4386.55941(80) | 2.06674(93) | 0.00262(32) | ⋯ | ⋯ | ⋯ | ⋯ | ⋯ | ⋯ |
K6g | V184: | 0.312 | 24 | 3 | 1.206 | 4411.8773(63) | 1.884(55) | −0.46(21) | 1.09(44) | −1.49(50) | 1.03(28) | −0.284(64) | ⋯ | ⋯ |
K6h | V145: | 0.311 | 29 | 3 | 1.121 | 4388.1291(45) | 2.313(27) | 0.091(78) | −0.24(11) | 0.287(90) | −0.160(35) | 0.0314(56) | ⋯ | ⋯ |
K6i | V061: | 0.227 | 27 | 8 | 1.208 | 4399.4906(12) | 2.1265(13) | 0.00638(43) | ⋯ | ⋯ | ⋯ | ⋯ | ⋯ | ⋯ |
K6j | V064: | 0.146 | 32 | 2 | 0.805 | 4401.17868(65) | 2.13354(74) | 0.00560(24) | ⋯ | ⋯ | ⋯ | ⋯ | ⋯ | ⋯ |
K6k | V078: | 0.099 | 30 | 1 | 1.069 | 4426.54770(94) | 2.2099(10) | 0.00665(34) | ⋯ | ⋯ | ⋯ | ⋯ | ⋯ | ⋯ |
K = 7 | ||||||||||||||
K7a | V013: | 0.892 | 31 | 3 | 1.108 | 4357.7248(14) | 1.6500(30) | −0.0152(23) | 0.00193(62) | ⋯ | ⋯ | ⋯ | ⋯ | ⋯ |
K7b | V123: | 0.388 | 30 | 2 | 1.280 | 4364.1594(10) | 1.8941(12) | −0.00551(40) | ⋯ | ⋯ | ⋯ | ⋯ | ⋯ | ⋯ |
K7c | V084: | 0.342 | 27 | 4 | 0.839 | 4360.3493(12) | 0.8838(24) | −0.1255(19) | 0.01255(49) | ⋯ | ⋯ | ⋯ | ⋯ | ⋯ |
K7d | V063: | 0.278 | 31 | 1 | 0.875 | 4400.6256(28) | 2.318(13) | 0.153(27) | −0.008(28) | −0.061(13) | 0.0192(26) | ⋯ | ⋯ | ⋯ |
K7e | V060: | 0.245 | 30 | 1 | 1.140 | 4398.34077(98) | 2.1058(11) | 0.00548(36) | ⋯ | ⋯ | ⋯ | ⋯ | ⋯ | ⋯ |
K7f | V077: | 0.229 | 32 | 0 | 0.734 | 4425.4453(10) | 2.1866(20) | 0.0030(15) | 0.00108(41) | ⋯ | ⋯ | ⋯ | ⋯ | ⋯ |
K7g | V069: | 0.166 | 33 | 1 | 1.114 | 4411.64576(89) | 2.1514(10) | 0.00692(33) | ⋯ | ⋯ | ⋯ | ⋯ | ⋯ | ⋯ |
K = 8 | ||||||||||||||
K8a | V021: | 0.682 | 26 | 2 | 0.960 | 4364.1271(15) | 1.9735(30) | −0.0069(23) | −0.00255(61) | ⋯ | ⋯ | ⋯ | ⋯ | ⋯ |
K8b | V059: | 0.491 | 31 | 2 | 1.242 | 4397.4177(17) | 2.1423(34) | 0.0089(27) | −0.00519(71) | ⋯ | ⋯ | ⋯ | ⋯ | ⋯ |
K8c | V174: | 0.380 | 31 | 1 | 1.303 | 4389.7174(45) | 1.802(19) | 0.453(38) | −0.382(36) | 0.417(17) | −0.0919(30) | ⋯ | ⋯ | ⋯ |
K8d | V194: | 0.296 | 18 | 1 | 1.154 | 4361.0443(33) | 0.6630(93) | 0.029(11) | 0.0788(68) | −0.0091(14) | ⋯ | ⋯ | ⋯ | ⋯ |
K = 9 | ||||||||||||||
K9a | V165: | 0.495 | 28 | 3 | 1.097 | 4373.1956(44) | −1.075(18) | 0.213(35) | 0.059(33) | 0.085(15) | −0.0336(27) | |||
K9b | V068: | 0.375 | 29 | 2 | 0.917 | 4409.55603(80) | 2.13686(88) | 0.00221(29) | ⋯ | ⋯ | ⋯ | ⋯ | ⋯ | ⋯ |
K9c | V152: | 0.261 | 25 | 1 | 0.829 | 4377.4805(12) | 1.6836(26) | −0.0156(20) | 0.00197(53) | ⋯ | ⋯ | ⋯ | ⋯ | ⋯ |
K9d | V035: | 0.282 | 27 | 2 | 1.073 | 4373.4274(16) | 2.0711(32) | 0.0191(25) | 0.00245(65) | ⋯ | ⋯ | ⋯ | ⋯ | ⋯ |
K9e | V034: | 0.146 | 25 | 3 | 1.331 | 4372.0337(12) | 2.0403(14) | 0.00960(48) | ⋯ | ⋯ | ⋯ | ⋯ | ⋯ | ⋯ |
K9f | V200: | 0.105 | 26 | 3 | 1.262 | 4396.09603(60) | 2.01705(26) | ⋯ | ⋯ | ⋯ | ⋯ | ⋯ | ⋯ | ⋯ |
K = 10 | ||||||||||||||
K10a | V027: | 0.393 | 27 | 3 | 1.196 | 4367.2143(19) | 1.4495(38) | 0.0095(30) | 0.00302(79) | ⋯ | ⋯ | ⋯ | ⋯ | ⋯ |
K10b | V074: | 0.241 | 27 | 2 | 1.062 | 4422.1124(10) | 2.1495(11) | 0.00574(38) | ⋯ | ⋯ | ⋯ | ⋯ | ⋯ | ⋯ |
K10c | V173: | 0.188 | 29 | 0 | 1.425 | 4392.41226(90) | 1.98881(28) | ⋯ | ⋯ | ⋯ | ⋯ | ⋯ | ⋯ | ⋯ |
Notes.
aThe notation of individual K sequences as described in the text following Equation (1). bThe identifier of the line sequence established in Cernicharo et al. (2016). cAutomatically assigned intensity of the sequence relative to the strongest sequence in the spectrum. dThe number of lines in the linear fit. eThe number of confidently assigned lines that were perturbed and were rejected from the fit. fUnitless (weighted) deviation of the effective rotor fit. gThe values of the parameters resulting from the effective linear-rotor fit.Table 2. Line List of K > 3 Rotational Transitions for CH3NCO Compiled from Experimental Frequencies (MHz) Assigned on the Basis of Stark-modulation Spectroscopy and Nuclear Quadrupole Hyperfine Structure
K = 4 | ||||||||||
---|---|---|---|---|---|---|---|---|---|---|
J' | J'' | K4aLa | K4aU | K4bL | K4bU | K4c | K4dL | K4dU | K4e | K4f |
5 | 4 | ⋯b | ⋯b | 42731.292c | 42731.292c | 43464.849c | 44034.686c | 44034.686c | 43890.896c | 43650.061c |
6 | 5 | ⋯b | ⋯b | 50964.759 | 50964.759 | 52157.325c | 52831.945c | 52831.945c | 52668.393c | 52379.568c |
7 | 6 | 58339.391 | 58339.391 | 59350.121 | 59350.121 | 60849.700 | 61624.560 | 61624.560 | 61445.688 | 61108.737 |
8 | 7 | 66984.159 | 66984.159 | 67783.970 | 67783.970 | 69541.847 | 70412.420 | 70412.420 | 70222.650 | 69837.656 |
9 | 8 | 75576.792 | 75576.792 | 76238.725 | 76238.725 | 78233.684 | 79195.280 | 79195.280 | 78999.235 | 78566.221 |
10 | 9 | 84141.319 | 84141.319 | 84705.058 | 84705.058 | 86925.110 | 87973.153 | 87973.153 | 87775.326 | 87294.305 |
Sequence | V129+X129 | V130+X130 | V102+X102 | V103+X103 | V004 | V141+X141 | V140+X140 | V051 | V108 |
Notes. All frequencies (unless otherwise marked) are experimental measurements with estimated uncertainty of 0.05 MHz.
aThe sequence notation as specified in the text. bTransition for which there is no experimental value and the extrapolation from a polynomial fit is not reliable. cIntensity-weighted average of the hyperfine doublet. (This table is available in its entirety in FITS format.)Download table as: ASCIITypeset image
Apart from the K > 3 transitions, a comprehensive investigation of the Stark spectra made it possible to assign also some K ≤ 3 transitions in the vb = 0 state of the parent isotopic species, such as K = −2, m = 1 or K = −1, m = 3L, that were missing in our preceding paper and that are expected to result in identifiable spectral features in the interstellar line surveys. Attempts were made to locate also K = −2, m = −2; K = −2, m = 3L; and K = −2, m = 3U sequences of transitions; however, they seem to be strongly perturbed. For completeness, K ≤ 3 transition sequences in the higher-energy m = 4, −5, and 6 internal rotor states of vb = 0, assigned by Koput (1986), were also extended into the millimeter-wave region. Ultimately, several K ≤ 3 sequences of transitions could also be identified in vb = 1 and vb = 2 excited vibrational states as shown in Figure 4. For all these K < 3 transitions we follow the notation from Cernicharo et al. (2016). In the case of the m = 0 state, for which asymmetric rotor notation Ka and Kc would normally be more appropriate, we use the notation K = nL, nU to distinguish between lower (Ka + Kc = J + 1) and upper (Ka + Kc = J) frequency sequences for the same value of Ka = n. Analogously, we use the notation m = 3L and 3U to distinguish between the two nearly degenerate m = 3 substates giving rise to lower and upper frequency transition sequences, respectively. Although in the foreseeable future astrophysical detection of lines corresponding to these higher vibrational energy states appears more challenging, it cannot be completely discounted as shown recently by detection of several lines of CH3NCO in vb = 1 toward Sgr B2(N2) (Belloche et al. 2017). The sets of parameters of the linear-rotor-type, J(J + 1) power series for all these additionally assigned K ≤ 3 sequences are given in Table 3, and the experimental frequencies are gathered in Table 4 . Finally, the JPL catalog line list for these sequences is provided in Table 5.
Figure 4. Typical Stark pattern (10 V modulation voltage) observed for the m = 0, Ka = 2 asymmetry doublet in the ground state vb = 0 and two successive excitations of the CNC bending mode vb = 1 and vb = 2. The irregular frequency progression of the three marked doublets is indicative of the anharmonicity/perturbations in the CNC bending motion.
Download figure:
Standard image High-resolution imageTable 3. Parameters of the Linear-rotor-type, J(J + 1) Power Series Fitted to the Additionally Assigned K ≤ 3 Line Sequences of the Parent Species
Ka | Nameb | Irelc | Nind | Nreje | σwf | B (MHz) | DJ (kHz) | HJ (Hz) | LJ (mHz) | PJ (μHz) | P12 (nHz) | P14 (pHz) | P16 (fHz) | P18 (aHz) |
---|---|---|---|---|---|---|---|---|---|---|---|---|---|---|
![]() |
||||||||||||||
−2a | V179: | 0.579 | 16 | 1 | 0.494 | 4350.3325(51) | −78.80(10) | −44.0(10) | −238.0(46) | 526.(10) | −356.5(84) | ⋯ | ⋯ | ⋯ |
−2b | X179: | 0.789 | 22 | 0 | 1.016 | 4344.71(97) | −125.0(46) | −253.(12) | 309.(20) | −260.(21) | 149.(14) | −55.4(64) | 12.1(15) | −1.16(16) |
![]() ![]() ![]() |
||||||||||||||
−1 | V138: | 0.663 | 34 | 5 | 1.577 | 4377.4365(70) | −10.872(77) | 3.73(40) | −27.1(11) | 24.8(17) | −20.7(16) | 13.66(90) | −4.81(27) | 0.658(34) |
![]() |
||||||||||||||
−1 | V164: | 0.502 | 36 | 3 | 1.259 | 4372.7579(28) | −0.421(13) | 0.001(29) | 0.560(30) | −0.246(14) | 0.0193(27) | ⋯ | ⋯ | ⋯ |
1 | V160: | 0.312 | 29 | 1 | 1.076 | 4372.9957(46) | 1.714(48) | −1.04(23) | 2.72(56) | −4.08(74) | 3.13(48) | −0.97(12) | ⋯ | ⋯ |
−2 | V229: | 0.461 | 26 | 0 | 1.183 | 4407.0035(81) | −23.20(14) | 87.6(12) | −344.4(54) | 243.(13) | 145.(18) | −259.(13) | 93.4(41) | ⋯ |
2 | V158: | 0.209 | 34 | 5 | 1.213 | 4379.0786(10) | 2.0825(11) | −0.00046(37) | ⋯ | ⋯ | ⋯ | ⋯ | ⋯ | ⋯ |
2' | V159: | 0.209 | 34 | 5 | 1.404 | 4379.0839(17) | 2.1043(38) | 0.0111(33) | −0.01225(93) | ⋯ | ⋯ | ⋯ | ⋯ | ⋯ |
![]() |
||||||||||||||
−1 | V045: | 0.252 | 34 | 2 | 0.854 | 4384.15287(41) | 2.11044(22) | ⋯ | ⋯ | ⋯ | ⋯ | ⋯ | ⋯ | ⋯ |
2 | V193: | 0.413 | 37 | 2 | 0.994 | 4388.1800(34) | −2.184(29) | 1.09(11) | −0.28(23) | 1.74(27) | −1.84(17) | 0.608(61) | −0.0661(86) | ⋯ |
−2 | V056: | 0.199 | 38 | 1 | 0.961 | 4393.35137(72) | 2.17888(83) | 0.00628(28) | ⋯ | ⋯ | ⋯ | ⋯ | ⋯ | ⋯ |
![]() |
||||||||||||||
0 | V175: | 0.377 | 35 | 3 | 1.248 | 4387.7938(21) | 2.1700(70) | 0.0974(97) | −0.0479(58) | 0.0092(13) | ⋯ | ⋯ | ⋯ | ⋯ |
−1 | V136: | 0.458 | 35 | 4 | 1.089 | 4383.39713(85) | 1.89120(97) | 0.00489(33) | ⋯ | ⋯ | ⋯ | ⋯ | ⋯ | ⋯ |
1 | V058: | 0.314 | 40 | 0 | 1.023 | 4395.72730(75) | 2.19786(86) | 0.00391(29) | ⋯ | ⋯ | ⋯ | ⋯ | ⋯ | ⋯ |
−2 | V147: | 0.533 | 37 | 2 | 1.054 | 4386.2913(12) | 0.8694(25) | 0.0705(20) | 0.02113(54) | ⋯ | ⋯ | ⋯ | ⋯ | ⋯ |
![]() |
||||||||||||||
2L | V090: | 0.356 | 36 | 3 | 1.077 | 4342.2441(23) | 3.281(11) | 0.144(24) | −0.118(24) | 0.062(11) | −0.0124(22) | ⋯ | ⋯ | ⋯ |
2U | V088: | 0.385 | 38 | 1 | 1.054 | 4342.2228(12) | −2.0956(25) | −0.1958(20) | −0.02294(53) | ⋯ | ⋯ | ⋯ | ⋯ | ⋯ |
3L | V104: | 0.339 | 32 | 5 | 0.852 | 4282.6194(34) | 13.690(30) | 7.53(11) | 2.05(21) | −4.58(22) | 2.59(13) | −0.707(44) | 0.0790(58) | ⋯ |
3U | V105: | 0.325 | 31 | 6 | 0.991 | 4282.6294(41) | 13.818(39) | 8.01(14) | 1.27(27) | −3.85(28) | 2.20(17) | −0.593(55) | 0.0653(72) | ⋯ |
![]() |
||||||||||||||
0a | V177: | 21 | 0 | 0.774 | 4353.3169(60) | 122.30(14) | 37.3(16) | 429.6(88) | −1143.(25) | 1306.(37) | −593.(21) | ⋯ | ⋯ | |
0b | X177: | 0.359 | 15 | 0 | 0.703 | 4357.44(27) | 164.3(13) | 264.3(34) | −288.8(52) | 202.9(47) | −83.0(22) | 15.02(45) | ⋯ | ⋯ |
−1 | V099: | 0.379 | 39 | 2 | 1.147 | 4294.0094(36) | −19.616(29) | −19.36(11) | 14.63(21) | −8.80(24) | 3.63(15) | −0.883(50) | 0.0942(66) | ⋯ |
![]() |
||||||||||||||
0 | V120: | 0.345 | 38 | 2 | 0.838 | 4355.3770(22) | 0.539(15) | 0.556(44) | −1.554(66) | 0.086(52) | 0.190(21) | −0.0384(33) | ⋯ | ⋯ |
−1 | V015: | 0.270 | 32 | 4 | 0.938 | 4357.25912(76) | 1.43704(90) | −0.00953(30) | ⋯ | ⋯ | ⋯ | ⋯ | ⋯ | ⋯ |
![]() |
||||||||||||||
0 | V095: | 0.187 | 41 | 1 | 0.740 | 4330.7106(23) | 6.917(19) | 0.415(70) | −0.42(13) | 0.50(15) | −0.316(96) | 0.102(31) | −0.0134(42) | ⋯ |
1L | V128: | 0.176 | 38 | 2 | 1.180 | 4294.05621(88) | 3.05470(94) | 0.07787(30) | ⋯ | ⋯ | ⋯ | ⋯ | ⋯ | ⋯ |
1U | V217: | 0.156 | 31 | 2 | 1.139 | 4375.67247(93) | 4.1429(10) | 0.03510(37) | ⋯ | ⋯ | ⋯ | ⋯ | ⋯ | ⋯ |
2L | V091: | 0.156 | 31 | 4 | 1.263 | 4343.4842(16) | 3.1549(33) | 0.0395(26) | −0.00193(70) | ⋯ | ⋯ | ⋯ | ⋯ | ⋯ |
2U | V113: | 0.172 | 33 | 4 | 1.091 | 4343.4743(13) | −1.0207(27) | −0.1855(22) | −0.01014(59) | ⋯ | ⋯ | ⋯ | ⋯ | ⋯ |
Notes.
aThe value of the Ka or K quantum number following the notation described in Cernicharo et al. (2016). bThe identifier of the line sequence established in Cernicharo et al. (2016). cAutomatically assigned intensity of the sequence relative to the strongest sequence in the spectrum. dThe number of lines in the linear fit. eThe number of confidently assigned lines that were perturbed and were rejected from the fit. fUnitless (weighted) deviation of the fit.Download table as: ASCIITypeset image
Table 4. Line List of Rotational Transitions for CH3NCO Compiled from Experimental Frequencies (MHz) for Additional K ≤ 3 Line Sequences Assigned on the Basis of Continuity from the Assignment Reached by Koput (1986) for J'' ≤ 3
![]() |
|||||
---|---|---|---|---|---|
J' | J'' | K = −1a | K = 1 | K = −2 | K = 2 |
2 | 1 | 17491.620b | 17491.620b | ⋯ | ⋯ |
3 | 2 | 26236.710b | 26237.850b | 26444.570b | 26274.290b |
4 | 3 | 34982.163 | 34983.529 | 35262.419 | 35032.080 |
5 | 4 | 43727.872 | 43729.167 | 44083.027 | 43789.693 |
6 | 5 | 52473.515 | 52474.398 | 52907.508 | 52547.163 |
7 | 6 | 61219.189c | 61219.500c | 61736.631 | 61304.239 |
8 | 7 | 69965.011 | 69964.211 | 70571.424 | 70060.972 |
Sequencea | V164 | V160 | V229 | V158 |
Notes. All frequencies (unless otherwise marked) are hyperfine-free experimental measurements with estimated uncertainty of 0.05 MHz.
aThe vibrational energies, K quantum number, and sequence notations are the same as in Cernicharo et al. (2016). bTaken from Koput (1986). cCalculated from a J(J + 1) power series fit to the sequence (mainly due to line blending). (This table is available in its entirety in FITS format.)Download table as: ASCIITypeset image
Table 5. JPL Catalog Line List for Additional K ≤ 3 Transitions of CH3NCO
Frequency | Error | Log(Int)a | DRb | Elow | guppc | TAGd | QNFMTe | QN'f | QN''g | ||||||||
---|---|---|---|---|---|---|---|---|---|---|---|---|---|---|---|---|---|
(MHz) | (MHz) | (cm−1) | J' |
![]() |
![]() |
![]() |
![]() |
![]() |
![]() |
![]() |
![]() |
![]() |
|||||
61299.229 | 0.0500 | −5.1774 | 3 | 89.9688 | 15 | 01 | 505 | 7 | −1 | 0 | 3 | 0 | 6 | −1 | 0 | 3 | 0 |
70061.612 | 0.0500 | −5.0059 | 3 | 92.0119 | 17 | 01 | 505 | 8 | −1 | 0 | 3 | 0 | 7 | −1 | 0 | 3 | 0 |
78825.997 | 0.0500 | −4.8562 | 3 | 94.3469 | 19 | 01 | 505 | 9 | −1 | 0 | 3 | 0 | 8 | −1 | 0 | 3 | 0 |
87592.559 | 0.0500 | −4.7237 | 3 | 96.9736 | 21 | 01 | 505 | 10 | −1 | 0 | 3 | 0 | 9 | −1 | 0 | 3 | 0 |
96361.445 | 0.0500 | −4.6051 | 3 | 99.8922 | 23 | 01 | 505 | 11 | −1 | 0 | 3 | 0 | 10 | −1 | 0 | 3 | 0 |
105132.553 | 0.0500 | −4.4982 | 3 | 103.1026 | 25 | 01 | 505 | 12 | −1 | 0 | 3 | 0 | 11 | −1 | 0 | 3 | 0 |
Notes. The key parameters used in the evaluation of this table, μ = μa = 2.882 D, T = 300 K, and Qvr = 138,369, are defined in Sections A.1 and A.2 of Cernicharo et al. (2016).
aBase-10 logarithm of the integrated intensity at 300 K in units of nm2 MHz. bDegrees of freedom in the rotational partition function. cUpper-state degeneracy. dSpecies tag or molecular identifier. eFormat of the quantum numbers. fQuantum numbers for the upper state. Ka and Kc quantum numbers are defined only for the m = 0 state. For m > 0: Ka = K and Kc = 0. gQuantum numbers for the lower state. Ka and Kc quantum numbers are defined only for the m = 0 state. For m > 0: Ka = K and Kc = 0.Only a portion of this table is shown here to demonstrate its form and content. A machine-readable version of the full table is available.
Download table as: DataTypeset image
A useful overview of the features hindering the solution of the spectroscopic problem for CH3NCO is provided in Figure 5. In this reduced frequency representation based on Equation (1) the sequences are expected to converge at low J to the effective values of B as tabulated in Tables 1 and 3. Only moderate (and uniform) curvature in the vertical direction is expected. A useful reference is provided by the quasi-linear NCNCS molecule (see Figure 8 of Winnewisser et al. 2010). For NCNCS the behavior appears to be less perturbed than that visible in Figure 5, but in that case reproduction of the rotational spectrum to within experimental accuracy has not yet been achieved. In the present case of CH3NCO the situation is considerably more complex since the most important, lowest-K sequences in the lowest internal rotation substates m = 0 and 1 appear to show the most unusual behavior. The m = 0, K = 3 sequence is already significantly shifted to low frequency relative to limited fits made with the standard asymmetric rotor model (as reproduced in the discussion of the 13C species further below). Furthermore, the most intense K = 4 sequence, and thus the first candidate to be assigned to m = 0, is displaced even more to low frequency and is highly nonlinear. It is only at higher K where the sequences are closer to the positions expected from standard asymmetric rotor theory, but their ordering is by no means regular. The behavior of the m = 1 sequences as assigned by Koput (1986), in particular of that for K = 0, is also very challenging. In principle, low barrier methyl group internal rotation is treatable with the RAM36 program of Ilyushin et al. (2010). The program has been very successful in treating toluene, which is a molecule with a very similar energy distribution of internal rotor states to that in CH3NCO, and also displaying a plethora of perturbations between m = 0 and 1 substates (Ilyushin et al. 2017). In fact, RAM36 has already been applied to CH3NCO (Halfen et al. 2015), albeit to a very small data set, which has later been shown to contain some misassignments (Cernicharo et al. 2016). The reported predictions allowed confident assignment in Sagittarius B2 of some conservatively chosen CH3NCO transitions (Halfen et al. 2015) but turned out to be of a very limited character when confronted with the complete laboratory data (Cernicharo et al. 2016). The currently available data set is being used to reach a more comprehensive RAM36 fit. Progress is slow, one of the reasons being that multiple competing parameters of fit are involved. The choice of numerical values of even the leading parameters defining internal rotation for this low barrier case, such as ρ, F, V3, Dab, and Qb (see Lin & Swalen 1959) is already found to be critical. Their values can be precalculated with moderate confidence, but in order to reach spectroscopic accuracy, each such parameter also needs to be supported by an associated centrifugal-type expansion, which is much more poorly understood. Furthermore, the J dependence of the observed line sequences also lacks the sharp resonances that allow precise identification of the positions of interacting vibration–rotation energy levels (as has been the case for toluene; Ilyushin et al. 2017). For the time being we prefer to make the extensive laboratory data available for applications and to cite Curl et al. (1963), who, on being confronted with the CH3NCO spectrum, stated, "There seems to be little value in reporting values of the constants obtained or details of the fits." Nevertheless, the analysis in terms of transition series of the complete rotational spectrum of CH3NCO up to 364 GHz allows approximate estimation of most of the rovibrational energy levels within 400 cm−1 or so from J = 0 of the ground state with Evr ( cm−1) = 0.14462 , where the two numerical constants are, respectively, (B + C)/2 and A − (B + C)/2 (from Cernicharo et al. 2016) and
are the vibrational substate energies from Koput (1986).
Figure 5. Illustration of the complexity underlying the rotational spectrum of CH3NCO as revealed by a reduced frequency plot of identified transition sequences. In this representation transition series for a "well-behaved" molecule are expected to show only relatively small curvature from the vertical, such as on the right side of the figure. This is clearly not the case for many important series. Color-coding: red—the lowest-K ground-state (m = 0) sequences; blue—the lowest- sequences; black—the most intense sequences for each value of K for the currently assigned K > 3 series; green—the remaining currently assigned K > 3 series; gray—other series. The values of K for selected series are indicated.
Download figure:
Standard image High-resolution image3.2. 13C Isotopic Species
Three different spectroscopic techniques were used for the assignment of rotational transitions of CH3N13CO and 13CH3NCO isotopologues. In a first step, their rotational signatures were searched for in natural abundance in the broadband chirped-pulse Fourier transform microwave spectrum (6–18 GHz and 25–26 GHz) acquired in our previous study (Cernicharo et al. 2016). This made possible the assignment of Ka = 0, m = 0, lines solely on the basis of predicted frequency shifts induced by isotopic substitution. In a next step, Stark-modulation spectroscopy on isotopically enriched samples was used to discriminate between transitions with different values of the K quantum number. The assignments of m and
quantum numbers were subsequently transferred from the parent species on the basis of similarities of spectral patterns for corresponding J regions (see Figure 6). Finally, the assigned sequences were extended into the millimeter-wave range. The isocyanate carbon atom is very close to the center of mass of CH3NCO, so that the
spectrum of CH3N13CO is shifted to low frequency by only ∼170 MHz. The effect on the line pattern in Figure 6 is seen to be minimal, so that quantum number assignment is readily transferable from the parent species. For 13CH3NCO the corresponding isotopic frequency shift is ∼1440 MHz, and changes in the line pattern are seen to be more significant. Nevertheless, the key lines are also assignable on the basis of Stark behavior and relative intensity. The experimental line lists for the assigned transition sequences of CH3N13CO and 13CH3NCO with the lowest vibrational energies that are expected to be key in future astrophysical identifications are given in Tables 6 and 7. The labeling of the individual sequences, as well as the quantum number nomenclature, is kept the same as for the parent species. Similarly to the parent species, only Ka = 0, 1, 2, m = 0,
transitions could be fitted together with reasonable terms in Watson's semirigid asymmetric rotor Hamiltonian. Results of such fits are given in Tables 8 and 9. Table 10 then summarizes the spectroscopic parameters for each of the identified line sequences of CH3N13CO and 13CH3NCO obtained from the linear-rotor fits to Equation (1). The similarities in their values to those for the parent species as compared in Table 10 corroborate the correct assignments. The JPL catalog line lists for CH3N13CO and 13CH3NCO that are most directly useful for astrophysical applications are reported in Tables 11 and 12, respectively.
Figure 6. Stark spectra of the region for the parent and the two 13C isotopic species of CH3NCO measured at modulation voltage of 10 V, with frequency axes aligned in a way highlighting the relatively small isotopic variation of the observed spectroscopic patterns. Comparison of the two top spectra gives evidence for the achieved high isotopic purity since no lines of the parent species are visible in the spectrum of CH3N13CO.
Download figure:
Standard image High-resolution imageTable 6. Line List of Rotational Transitions for CH3N13CO Compiled from Experimental Frequencies (MHz) for Line Sequences Assigned on the Basis of CP-FTMW, Stark-modulated, and Frequency-modulated Millimeter-wave Spectra
![]() |
||||||||
---|---|---|---|---|---|---|---|---|
J' | J'' | K = 0a | K = 1L | K = 1U | K = 2L | K = 2U | K = 3L | K = 3U |
6 | 5 | 51853.337 | 51403.416 | 52340.000 | 51905.699 | 51910.851 | 51726.586 | 51726.586 |
7 | 6 | 60492.530 | 59969.439 | 61061.832 | 60555.431 | 60563.705 | 60345.859 | 60345.859 |
8 | 7 | 69130.253 | 68534.899 | 69782.881 | 69204.658 | 69217.015 | 68964.201 | 68964.201 |
9 | 8 | 77766.490 | 77099.721 | 78503.174 | 77853.205 | 77870.697 | 77581.512 | 77581.512 |
10 | 9 | 86400.869b | 85663.778 | 87222.481 | 86501.119 | 86525.020 | 86197.856 | 86197.856 |
11 | 10 | 95033.255b | 94227.186b | 95940.874b | 95148.303b | 95180.269b | 94812.773b | 94812.852b |
12 | 11 | 103663.458b | 102789.647b | 104658.087b | 103794.561b | 103836.065b | 103426.398b | 103426.526b |
Sequencea | V093 | V126 | V131 | V089 | V006 | V096 | V097 |
Notes. All frequencies (unless otherwise marked) are hyperfine-free experimental measurements with estimated uncertainty of 0.05 MHz.
aThe vibrational energies, K quantum number notation, and the labeling of the individual line sequences are the same as for the parent species in Cernicharo et al. (2016). bCalculated from a J(J + 1) power series fit to the sequence (interpolation due to line blending or missing data). (This table is available in its entirety in FITS format.)Download table as: ASCIITypeset image
Table 7. Line List of Rotational Transitions for 13CH3NCO Compiled from Experimental Frequencies (MHz) for Line Sequences Assigned on the Basis of CP-FTMW, Stark-modulated, and Frequency-modulated Millimeter-wave Spectra
![]() |
||||||||
---|---|---|---|---|---|---|---|---|
J' | J'' | K = 0a | K = 1L | K = 1U | K = 2L | K = 2U | K = 3L | K = 3U |
6 | 5 | 50583.272 | 50151.340 | 51050.741 | 50634.955 | 50639.578 | 50482.694 | 50482.694 |
7 | 6 | 59010.994 | 58508.723 | 59557.732 | 59072.857 | 59080.538 | 58894.756 | 58894.756 |
8 | 7 | 67437.329 | 66865.576 | 68064.121 | 67510.342 | 67521.855 | 67306.163 | 67306.163 |
9 | 8 | 75862.078 | 75221.855 | 76569.588 | 75947.215 | 75963.479 | 75716.641 | 75716.641 |
10 | 9 | 84285.279b | 83577.376 | 85074.249 | 84383.466 | 84405.827 | 84126.212 | 84126.212 |
11 | 10 | 92706.543 | 91932.271b | 93577.977b | 92818.970b | 92848.738b | 92534.703b | 92534.775b |
12 | 11 | 101125.795 | 100286.312 | 102080.644 | 101253.657 | 101292.289 | 100942.013 | 100942.013 |
Sequencea | V093 | V126 | V131 | V089 | V006 | V096 | V097 |
Notes. All frequencies (unless otherwise marked) are hyperfine-free experimental measurements with estimated uncertainty of 0.05 MHz.
aThe vibrational energies, K quantum number notation, and the labeling of the individual line sequences are the same as for the parent species in Cernicharo et al. (2016). bCalculated from a J(J + 1) power series fit to the sequence (interpolation due to line blending or missing data). (This table is available in its entirety in FITS format.)Download table as: ASCIITypeset image
Table 8.
Spectroscopic Constants for the Ground States (, m = 0) of CH3N13CO and 13CH3NCO in Comparison with the Parent Species
Constant | Parent Speciesa | CH3N13CO | 13CH3NCO |
---|---|---|---|
A (MHz) | 128435.(19) | 128324.7(39) | 127110.(25) |
B (MHz) | 4414.6182(93) | 4400.1847(12) | 4291.1542(90) |
C (MHz) | 4256.7490(85) | 4243.2158(11) | 4140.4916(82) |
ΔJ (kHz) | 2.3209(11) | 2.30880(17) | 2.22320(57) |
ΔJK (kHz) | −1274.0(13) | −1273.73(12) | −1240.5(14) |
δJ (kHz) | 0.4042(14) | 0.40068(25) | 0.37712(92) |
δK (kHz) | 183.1(43) | [183.1]b | 160.8(41) |
![]() |
−0.00191(47) | [−0.00191]b | [−0.00191]b |
ΦJK (Hz) | −5.39(24) | [−5.39]b | −4.08(33) |
![]() |
−68478.(285) | [−68478.]b | −63001.(300) |
ϕJK (Hz) | −47.6(14) | [−47.61]b | −44.94(78) |
Nlinesc | 201 | 160 | 161 |
σfitd (kHz) | 94.6 | 88.5 | 99.6 |
σwe | 1.89 | 1.77 | 1.99 |
Notes. Numbers in parentheses are standard errors in units of the last digit.
aTaken from Cernicharo et al. (2016). bFixed to the parent species value. cThe number of fitted lines. dStandard deviation of the fit. eUnitless (weighted) deviation of the fit.Download table as: ASCIITypeset image
Table 9.
Results of Fitting the Spectroscopic Constants of Table 8 for CH3N13CO and 13CH3NCO Ground States (, m = 0)
CH3N13CO | 13CH3NCO | ||||||||||||||||
---|---|---|---|---|---|---|---|---|---|---|---|---|---|---|---|---|---|
J' |
![]() |
![]() |
![]() |
![]() |
![]() |
Obs.a | O. – C.b | Unc.c | J' |
![]() |
![]() |
J'' |
![]() |
![]() |
Obs.a | O. – C.b | Unc.c |
(MHz) | (MHz) | (MHz) | (MHz) | (MHz) | (MHz) | ||||||||||||
1 | 0 | 1 | 0 | 0 | 0 | 8643.400d | 0.008 | 0.050 | 1 | 0 | 1 | 0 | 0 | 0 | 8431.640d | 0.003 | 0.050 |
2 | 0 | 2 | 1 | 0 | 1 | 17286.600d | 0.019 | 0.050 | 2 | 0 | 2 | 1 | 0 | 1 | 16863.100d | 0.015 | 0.050 |
3 | 0 | 3 | 2 | 0 | 2 | 25929.400d | 0.032 | 0.050 | 3 | 0 | 3 | 2 | 0 | 2 | 25294.240d | 0.087 | 0.050 |
6 | 0 | 6 | 5 | 0 | 5 | 51853.337 | 0.039 | 0.050 | 6 | 0 | 6 | 5 | 0 | 5 | 50583.272 | 0.076 | 0.050 |
7 | 0 | 7 | 6 | 0 | 6 | 60492.530 | 0.065 | 0.050 | 7 | 0 | 7 | 6 | 0 | 6 | 59010.994 | 0.131 | 0.050 |
8 | 0 | 8 | 7 | 0 | 7 | 69130.253 | 0.023 | 0.050 | 8 | 0 | 8 | 7 | 0 | 7 | 67437.329 | 0.118 | 0.050 |
Notes.
aExperimentally observed frequency. bObserved minus calculated frequency. cExperimental uncertainty. dHyperfine-free frequency. (This table is available in its entirety in FITS format.)Download table as: ASCIITypeset image
Table 10. Comparison of Parameters of the Linear-rotor-type, J(J + 1) Power Series for the Same Line Sequence for the Parent, CH3N13CO, and 13CH3NCO Species
Species | Ka | Nameb |
![]() |
Nind | Nreje | σwf (MHz) | B (MHz) | DJ (kHz) | HJ (Hz) | LJ (mHz) | PJ (μHz) | P12 (nHz) | P14 (pHz) | P16 (fHz) | P18 (aHz) |
---|---|---|---|---|---|---|---|---|---|---|---|---|---|---|---|
![]() |
|||||||||||||||
CH3NCO | 0 | V093: | 0.843 | 41 | 1 | 0.910 | 4335.6996 (13) | 8.4815 (43) | 0.1971 (58) | 0.0728 (33) | −0.00909 (69) | ⋯ | ⋯ | ⋯ | ⋯ |
CH3N13CO | V093: | 0.997 | 36 | 0 | 0.735 | 4321.7180 (14) | 8.4036 (41) | 0.1946 (52) | 0.0714 (29) | −0.00887 (60) | ⋯ | ⋯ | ⋯ | ⋯ | |
13CH3NCO | V093: | 0.730 | 37 | 0 | 1.243 | 4215.8348 (15) | 7.8872 (29) | 0.1644 (22) | 0.07012 (55) | [−0.00909]g | ⋯ | ⋯ | ⋯ | ⋯ | |
CH3NCO | 1L | V126: | 0.783 | 38 | 2 | 0.967 | 4297.6203 (10) | 3.3744 (21) | 0.1133 (16) | −0.00393 (41) | ⋯ | ⋯ | ⋯ | ⋯ | ⋯ |
CH3N13CO | V126: | 0.898 | 31 | 0 | 0.659 | 4283.85898 (98) | 3.3465 (20) | 0.1100 (16) | −0.00358 (44) | ⋯ | ⋯ | ⋯ | ⋯ | ⋯ | |
13CH3NCO | V126: | 1.000 | 32 | 2 | 0.862 | 4179.5051 (10) | 3.1806 (19) | 0.0960 (14) | −0.00223 (34) | ⋯ | ⋯ | ⋯ | ⋯ | ⋯ | |
CH3NCO | 1U | V131: | 0.786 | 38 | 2 | 1.249 | 4376.1857 (14) | 4.2752 (30) | −0.0053 (24) | 0.00245 (67) | ⋯ | ⋯ | ⋯ | ⋯ | ⋯ |
CH3N13CO | V131: | 0.948 | 31 | 0 | 0.730 | 4361.97551 (67) | 4.24135 (82) | −0.00599 (29) | [0.00245] | ⋯ | ⋯ | ⋯ | ⋯ | ⋯ | |
13CH3NCO | V131: | 0.943 | 28 | 1 | 0.770 | 4254.5199 (11) | 4.0287 (25) | −0.0120 (22) | 0.00598 (64) | ⋯ | ⋯ | ⋯ | ⋯ | ⋯ | |
CH3NCO | 2L | V089: | 0.761 | 38 | 1 | 1.101 | 4339.69609 (82) | 3.28715 (94) | 0.03891 (31) | ⋯ | ⋯ | ⋯ | ⋯ | ⋯ | ⋯ |
CH3N13CO | V089: | 0.760 | 31 | 0 | 0.737 | 4325.71123 (68) | 3.26463 (82) | 0.03850 (30) | ⋯ | ⋯ | ⋯ | ⋯ | ⋯ | ⋯ | |
13CH3NCO | V089: | 0.725 | 33 | 1 | 0.693 | 4219.79574 (53) | 3.11301 (58) | 0.03494 (18) | ⋯ | ⋯ | ⋯ | ⋯ | ⋯ | ⋯ | |
CH3NCO | 2U | V006: | 0.835 | 38 | 1 | 1.046 | 4339.6811 (16) | −2.8764 (56) | −0.1690 (77) | −0.0676 (47) | 0.0079 (10) | ⋯ | ⋯ | ⋯ | ⋯ |
CH3N13CO | V006: | 0.747 | 30 | 1 | 0.742 | 4325.69904 (71) | −2.82860 (86) | −0.16306 (31) | [−0.0676] | [0.0079] | ⋯ | ⋯ | ⋯ | ⋯ | |
13CH3NCO | V006: | 0.917 | 27 | 0 | 0.870 | 4219.7862 (15) | −2.5537 (40) | −0.1440 (43) | −0.0629 (15) | [0.0079] | ⋯ | ⋯ | ⋯ | ⋯ | |
CH3NCO | 3L | V096: | 0.734 | 34 | 5 | 1.123 | 4324.6812 (18) | 5.1738 (58) | −0.2812 (77) | 0.1712 (44) | −0.01590 (94) | ⋯ | ⋯ | ⋯ | ⋯ |
CH3N13CO | V096: | 0.792 | 29 | 0 | 0.897 | 4310.9131 (14) | 5.0840 (30) | −0.2833 (25) | 0.16765 (73) | [−0.01590] | ⋯ | ⋯ | ⋯ | ⋯ | |
13CH3NCO | V096: | 0.492 | 27 | 1 | 1.249 | 4207.1970 (20) | 4.3934 (52) | −0.2735 (54) | 0.1312 (18) | [−0.01590] | ⋯ | ⋯ | ⋯ | ⋯ | |
CH3NCO | 3U | V097: | 0.808 | 35 | 4 | 1.078 | 4324.6762 (18) | 5.1686 (55) | −0.1769 (74) | 0.1479 (43) | −0.01455 (89) | ⋯ | ⋯ | ⋯ | ⋯ |
CH3N13CO | V097: | 0.777 | 29 | 0 | 0.907 | 4310.9126 (14) | 5.0856 (31) | −0.1762 (26) | 0.14395 (74) | [−0.01455] | ⋯ | ⋯ | ⋯ | ⋯ | |
13CH3NCO | V097: | 0.492 | 24 | 3 | 0.950 | 4207.1944 (17) | 4.3939 (43) | −0.1719 (43) | 0.1092 (14) | [−0.01455] | ⋯ | ⋯ | ⋯ | ⋯ | |
![]() |
|||||||||||||||
CH3NCO | 0a | V107: | 0.625 | 22 | 0 | 1.016 | 4357.5774(71) | 132.34(14) | 86.4(14) | 226.1(67) | −665.(17) | 719.(21) | −298.(11) | ⋯ | ⋯ |
CH3N13CO | V107: | 0.935 | 14 | 0 | 0.603 | 4343.4612(83) | 130.81(12) | 85.56(77) | 220.1(24) | −651.3(39) | 709.3(23) | [−298] | ⋯ | ⋯ | |
13CH3NCO | V107: | 0.796 | 13 | 0 | 0.362 | 4236.403(10) | 120.50(20) | 74.5(19) | 194.9(96) | −549.(25) | 571.(33) | −228.(17) | ⋯ | ⋯ | |
CH3NCO | 0b | X107: | 0.990 | 20 | 0 | 1.125 | 4348.7(10) | 116.2(37) | 146.2(74) | −125.4(87) | 71.4(65) | −25.9(29) | 5.39(74) | −0.493(81) | ⋯ |
CH3N13CO | X107: | 0.815 | 18 | 0 | 0.948 | 4334.99(43) | 116.1(14) | 146.0(24) | −125.4(24) | 71.5(14) | −25.91(44) | 5.404(59) | [−0.493] | ⋯ | |
13CH3NCO | X107: | 0.983 | 14 | 0 | 1.169 | 4230.37(47) | 114.4(14) | 144.0(21) | −124.1(18) | 71.01(81) | −25.82(14) | [5.39] | [−0.493] | ⋯ | |
CH3NCO | −1 | V101: | 0.755 | 39 | 1 | 1.000 | 4282.7615(32) | −3.118(26) | 9.39(10) | −10.67(19) | 6.64(21) | −2.59(13) | 0.590(44) | −0.0593(59) | ⋯ |
CH3N13CO | V101: | 0.917 | 31 | 0 | 0.572 | 4269.4950(20) | −3.039(13) | 8.933(42) | −10.023(65) | 6.192(53) | −2.434(22) | 0.5668(37) | [−0.0593] | ⋯ | |
13CH3NCO | V101: | 0.742 | 26 | 0 | 1.171 | 4169.4635(56) | −1.903(49) | 6.60(18) | −6.47(34) | 3.62(35) | −1.43(17) | 0.398(36) | [−0.0593] | ⋯ | |
CH3NCO | 1 | V098: | 0.797 | 36 | 5 | 0.961 | 4284.5720(42) | −35.940(44) | −45.38(21) | 49.98(57) | −45.27(87) | 29.62(81) | −12.71(44) | 3.16(12) | −0.342(15) |
CH3N13CO | V098: | 1.000 | 30 | 0 | 0.683 | 4270.9525(37) | −35.408(36) | −44.29(15) | 48.24(33) | −43.37(41) | 28.35(28) | −12.24(10) | 3.086(16) | [−0.342] | |
13CH3NCO | V098: | 0.881 | 26 | 0 | 0.950 | 4167.8312(70) | −32.204(79) | −39.92(38) | 44.17(97) | −41.1(13) | 27.9(11) | −12.39(47) | 3.150(85) | [−0.342] | |
CH3NCO | 2 | V010: | 0.660 | 31 | 2 | 0.845 | 4350.3415(23) | −2.325(15) | −0.065(46) | −0.290(64) | 0.284(43) | −0.109(11) | ⋯ | ⋯ | ⋯ |
CH3N13CO | V010: | 0.728 | 25 | 5 | 1.293 | 4336.3057(24) | −2.3166(70) | −0.0848(78) | −0.2813(29) | [0.284] | [−0.109] | ⋯ | ⋯ | ⋯ | |
13CH3NCO | V010: | 0.889 | 23 | 4 | 1.004 | 4229.9947(19) | −2.1780(61) | −0.0500(75) | −0.2959(30) | [0.284] | [−0.109] | ⋯ | ⋯ | ⋯ | |
CH3NCO | −3 | V005: | 0.800 | 36 | 2 | 1.153 | 4343.3487(25) | 0.096(11) | 0.288(25) | −0.381(25) | 0.202(12) | −0.0848(22) | ⋯ | ⋯ | ⋯ |
CH3N13CO | V005: | 0.741 | 31 | 0 | 0.944 | 4329.3382(26) | 0.074(13) | 0.171(29) | −0.225(32) | 0.109(17) | −0.0625(34) | ⋯ | ⋯ | ⋯ | |
13CH3NCO | V005: | 0.870 | 26 | 0 | 1.161 | 4223.2217(29) | 0.190(12) | 0.291(22) | −0.402(18) | 0.2363(55) | [−0.0848] | ⋯ | ⋯ | ⋯ | |
CH3NCO | 3 | V012: | 0.642 | 34 | 3 | 0.973 | 4356.1489(11) | 1.6845(24) | −0.0204(19) | 0.00189(52) | ⋯ | ⋯ | ⋯ | ⋯ | ⋯ |
CH3N13CO | V012: | 0.617 | 30 | 0 | 0.865 | 4342.10839(42) | 1.67820(20) | [−0.0204] | [0.00189] | ⋯ | ⋯ | ⋯ | ⋯ | ⋯ | |
13CH3NCO | V012: | 0.726 | 25 | 1 | 0.891 | 4235.6898(10) | 1.6380(15) | −0.01831(70) | [0.00189] | ⋯ | ⋯ | ⋯ | ⋯ | ⋯ | |
![]() |
|||||||||||||||
CH3NCO | 0 | V119: | 0.774 | 36 | 1 | 1.001 | 4356.2099(29) | 2.431(15) | 0.837(33) | −2.676(29) | ⋯ | 0.851(17) | −0.314(10) | 0.0333(20) | ⋯ |
CH3N13CO | V119: | 0.750 | 29 | 0 | 1.012 | 4342.0974(31) | 2.423(12) | 0.793(21) | −2.595(15) | ⋯ | 0.8246(46) | −0.3063(14) | [0.0333] | ⋯ | |
13CH3NCO | V119: | 0.825 | 25 | 0 | 0.948 | 4235.2124(29) | 2.433(16) | 0.570(38) | −2.093(34) | ⋯ | 0.629(16) | −0.2422(64) | [0.0333] | ⋯ | |
CH3NCO | −1 | V008: | 0.708 | 38 | 2 | 1.243 | 4353.2529(11) | 1.0831(14) | ⋯ | 0.00928(84) | −0.00378(31) | ⋯ | ⋯ | ⋯ | ⋯ |
CH3N13CO | V008: | 0.664 | 30 | 1 | 0.520 | 4339.19340(41) | 1.08491(35) | ⋯ | 0.009162(61 | [−0.00378] | ⋯ | ⋯ | ⋯ | ⋯ | |
13CH3NCO | V008: | 0.759 | 26 | 0 | 0.928 | 4232.66949(92) | 1.09494(97) | ⋯ | 0.00852(25) | [−0.00378] | ⋯ | ⋯ | ⋯ | ⋯ | |
CH3NCO | 1 | V180: | 0.807 | 40 | 0 | 3.750 | 4350.771(14) | −40.79(16) | −39.36(81) | −24.5(22) | 74.5(34) | −69.5(32) | 34.7(17) | −9.27(54) | 1.045(69) |
CH3N13CO | V180: | 0.743 | 27 | 1 | 1.061 | 4336.6441(84) | −40.08(10) | −37.91(53) | −26.7(14) | 78.8(24) | −75.3(24) | 39.0(14) | −10.93(48) | 1.301(64) | |
13CH3NCO | V180: | 0.901 | 26 | 0 | 0.872 | 4230.0429(99) | −35.28(13) | −27.23(83) | −41.3(27) | 100.7(56) | −102.8(69) | 60.6(50) | −20.1(20) | 2.89(34) | |
CH3NCO | 2 | V100: | 0.805 | 39 | 0 | 1.677 | 4276.5950(66) | −51.195(68) | −64.24(33) | 63.79(84) | −50.5(12) | 29.3(11) | −11.35(59) | 2.60(17) | −0.262(20) |
CH3N13CO | V100: | 0.972 | 31 | 0 | 0.813 | 4262.8094(60) | −50.410(69) | −62.56(34) | 61.94(90) | −49.7(14) | 29.7(13) | −12.06(75) | 2.92(23) | −0.316(30) | |
13CH3NCO | V100: | 0.749 | 26 | 0 | 0.529 | 4157.4953(48) | −46.639(52) | −53.90(24) | 51.23(59) | −40.60(82) | 24.36(66) | −9.98(28) | 2.432(50) | [−0.262] | |
CH3NCO | −3 | V024: | 0.473 | 35 | 3 | 1.105 | 4366.9265(12) | 1.9129(27) | −0.0098(22) | 0.00672(61) | ⋯ | ⋯ | ⋯ | ⋯ | ⋯ |
CH3N13CO | V024: | 0.586 | 28 | 1 | 0.516 | 4352.85172(51) | 1.90448(61) | −0.00915(22) | [0.00672] | ⋯ | ⋯ | ⋯ | ⋯ | ⋯ | |
13CH3NCO | V024: | 0.612 | 25 | 0 | 0.933 | 4246.1256(19) | 1.8213(48) | −0.0204(50) | 0.0153(17) | ⋯ | ⋯ | ⋯ | ⋯ | ⋯ | |
CH3NCO | 3a | V106: | 0.523 | 21 | 0 | 0.860 | 4373.6038(50) | 110.306(69) | 93.71(41) | −32.2(12) | −11.7(16) | 10.81(88) | ⋯ | ⋯ | ⋯ |
CH3N13CO | V106: | 0.901 | 14 | 0 | 0.761 | 4359.2931(53) | 108.531(50) | 91.21(19) | −30.46(32) | −12.23(19) | [10.81] | ⋯ | ⋯ | ⋯ | |
13CH3NCO | V106: | 0.750 | 15 | 0 | 0.907 | 4251.1541(59) | 98.593(61) | 78.31(23) | −22.26(38) | −14.34(22) | [10.81] | ⋯ | ⋯ | ⋯ | |
CH3NCO | 3b | X106: | 0.884 | 18 | 0 | 1.049 | 4370.20(59) | 103.8(16) | 95.6(24) | −55.9(22) | 20.9(11) | −4.49(32) | 0.438(38) | ⋯ | ⋯ |
CH3N13CO | X106: | 0.915 | 15 | 0 | 0.789 | 4356.21(28) | 103.12(70) | 94.79(92) | −55.57(67) | 20.80(25) | −4.491(38) | [0.438] | ⋯ | ⋯ | |
13CH3NCO | X106: | 0.761 | 12 | 0 | 0.884 | 4250.21(17) | 99.32(38) | 90.73(40) | −53.67(21) | 20.425(44) | [−4.49] | [0.438] | ⋯ | ⋯ | |
![]() ![]() |
|||||||||||||||
CH3NCO | 0 | V017: | 0.562 | 38 | 2 | 1.223 | 4361.0342(19) | 0.6664(66) | 0.0255(94) | 0.0843(57) | −0.0269(12) | ⋯ | ⋯ | ⋯ | ⋯ |
CH3N13CO | V017: | 0.637 | 29 | 1 | 0.708 | 4346.9119(11) | 0.6646(24) | 0.0166(21) | 0.08616(60) | [−0.0269] | ⋯ | ⋯ | ⋯ | ⋯ | |
13CH3NCO | V017: | 0.625 | 23 | 2 | 0.655 | 4239.94822(81) | 0.7203(12) | 0.00654(55) | [0.0843] | [−0.0269] | ⋯ | ⋯ | ⋯ | ⋯ | |
CH3NCO | −1 | V137: | 0.654 | 37 | 2 | 1.852 | 4376.0833(78) | −11.011(83) | 3.34(41) | −25.5(11) | 21.8(17) | −17.3(16) | 11.56(91) | −4.15(27) | 0.576(34) |
CH3N13CO | V137: | 0.773 | 31 | 0 | 1.204 | 4361.8394(91) | −10.80(10) | 3.54(55) | −25.5(15) | 21.7(25) | −16.6(25) | 10.7(15) | −3.69(50) | 0.492(67) | |
13CH3NCO | V137: | 0.711 | 26 | 0 | 0.753 | 4253.8242(60) | −9.695(71) | 2.35(35) | −20.71(93) | 18.5(13) | −15.6(11) | 10.42(54) | −3.82(10) | [0.576] | |
CH3NCO | 1 | V082: | 0.485 | 39 | 0 | 1.398 | 4362.15134(58) | 1.68176(26) | ⋯ | ⋯ | ⋯ | ⋯ | ⋯ | ⋯ | ⋯ |
CH3N13CO | V082: | 0.554 | 30 | 0 | 0.912 | 4348.05955(44) | 1.67676(21) | ⋯ | ⋯ | ⋯ | ⋯ | ⋯ | ⋯ | ⋯ | |
13CH3NCO | V082: | 0.604 | 25 | 0 | 1.053 | 4241.26821(63) | 1.64066(37) | ⋯ | ⋯ | ⋯ | ⋯ | ⋯ | ⋯ | ⋯ | |
CH3NCO | 2 | v030: | 0.399 | 35 | 3 | 1.347 | 4369.5669(10) | 1.9458(12) | −0.00228(41) | ⋯ | ⋯ | ⋯ | ⋯ | ⋯ | ⋯ |
CH3N13CO | V030: | 0.460 | 27 | 0 | 1.060 | 4355.46916(55) | 1.93746(26) | [−0.00228] | ⋯ | ⋯ | ⋯ | ⋯ | ⋯ | ⋯ | |
13CH3NCO | V030: | 0.465 | 23 | 0 | 1.102 | 4248.58309(71) | 1.87900(41) | [−0.00228] | ⋯ | ⋯ | ⋯ | ⋯ | ⋯ | ⋯ | |
CH3NCO | 3 | V040: | 0.634 | 33 | 2 | 1.276 | 4378.65685(60) | 2.06406(30) | ⋯ | ⋯ | ⋯ | ⋯ | ⋯ | ⋯ | ⋯ |
CH3N13CO | V040: | 0.774 | 25 | 3 | 0.635 | 4364.54115(35) | 2.05499(20) | ⋯ | ⋯ | ⋯ | ⋯ | ⋯ | ⋯ | ⋯ | |
13CH3NCO | V040: | 0.691 | 23 | 3 | 0.787 | 4257.49302(52) | 1.98668(35) | ⋯ | ⋯ | ⋯ | ⋯ | ⋯ | ⋯ | ⋯ | |
![]() |
|||||||||||||||
CH3NCO | 0 | V018: | 0.546 | 39 | 2 | 0.925 | 4361.4499(14) | 0.5247(49) | 0.0221(69) | 0.0949(42) | −0.02898(93) | ⋯ | ⋯ | ⋯ | ⋯ |
CH3N13CO | V018: | 0.672 | 30 | 0 | 0.607 | 4347.33135(97) | 0.5270(20) | 0.0145(17) | 0.09635(50) | [−0.02898] | ⋯ | ⋯ | ⋯ | ⋯ | |
13CH3NCO | V018: | 0.682 | 25 | 1 | 0.857 | 4240.3532(10) | 0.5908(15) | 0.00154(67) | [0.0949] | [−0.02898] | ⋯ | ⋯ | ⋯ | ⋯ | |
CH3NCO | 1 | V020: | 0.496 | 36 | 3 | 0.888 | 4363.55034(68) | 1.72074(80) | −0.00110(27) | ⋯ | ⋯ | ⋯ | ⋯ | ⋯ | ⋯ |
CH3N13CO | V020: | 0.583 | 28 | 1 | 0.842 | 4349.45678(41) | 1.71616(20) | [−0.00110] | ⋯ | ⋯ | ⋯ | ⋯ | ⋯ | ⋯ | |
13CH3NCO | V020: | 0.572 | 24 | 2 | 0.674 | 4242.61566(82) | 1.6725(12) | −0.00365(55) | ⋯ | ⋯ | ⋯ | ⋯ | ⋯ | ⋯ | |
CH3NCO | 2 | v029: | 0.406 | 34 | 4 | 1.138 | 4369.57115(94) | 1.9779(10) | 0.00072(36) | ⋯ | ⋯ | ⋯ | ⋯ | ⋯ | ⋯ |
CH3N13CO | V029: | 0.457 | 27 | 0 | 0.769 | 4355.47169(39) | 1.96867(19) | [0.00072] | ⋯ | ⋯ | ⋯ | ⋯ | ⋯ | ⋯ | |
13CH3NCO | V029: | 0.466 | 24 | 0 | 0.791 | 4248.58532(51) | 1.90805(29) | [0.00072] | ⋯ | ⋯ | ⋯ | ⋯ | ⋯ | ⋯ | |
![]() |
|||||||||||||||
CH3NCO | 0 | V025: | 0.420 | 37 | 3 | 1.039 | 4368.7341(11) | 1.5662(25) | 0.0039(21) | 0.00218(57) | ⋯ | ⋯ | ⋯ | ⋯ | ⋯ |
CH3N13CO | V025: | 0.516 | 28 | 0 | 0.658 | 4354.59556(64) | 1.56131(78) | 0.00303(28) | [0.00218] | ⋯ | ⋯ | ⋯ | ⋯ | ⋯ | |
13CH3NCO | V025: | 0.525 | 24 | 2 | 0.864 | 4247.4371(10) | 1.5348(15) | −0.00030(71) | [0.00218] | ⋯ | ⋯ | ⋯ | ⋯ | ⋯ | |
![]() |
|||||||||||||||
CH3NCO | 0 | V142: | 0.300 | 38 | 1 | 0.973 | 4377.7029(11) | 1.8930(23) | −0.0136(19) | 0.00256(51) | ⋯ | ⋯ | ⋯ | ⋯ | ⋯ |
CH3N13CO | V142: | 0.351 | 29 | 0 | 0.838 | 4363.54378(81) | 1.88911(98) | −0.01291(35) | [0.00256] | ⋯ | ⋯ | ⋯ | ⋯ | ⋯ | |
13CH3NCO | V142: | 0.336 | 22 | 0 | 0.958 | 4256.1828(12) | 1.8473(18) | −0.00969(79) | [0.00256] | ⋯ | ⋯ | ⋯ | ⋯ | ⋯ | |
![]() |
|||||||||||||||
CH3NCO | 0 | V094: | 0.395 | 41 | 0 | 0.991 | 4335.3010(15) | 7.8017(47) | 0.2011(63) | 0.0443(37) | −0.00561(76) | ⋯ | ⋯ | ⋯ | ⋯ |
CH3N13CO | V094: | 0.426 | 31 | 0 | 0.985 | 4321.35444(91) | 7.7351(11) | 0.19638(40) | [0.0443] | [−0.00561] | ⋯ | ⋯ | ⋯ | ⋯ | |
13CH3NCO | V094: | 0.330 | 26 | 0 | 1.101 | 4215.5503(20) | 7.2894(49) | 0.1797(49) | 0.0413(16) | [−0.00561] | ⋯ | ⋯ | ⋯ | ⋯ | |
CH3NCO | 1L | V127: | 0.373 | 38 | 2 | 1.037 | 4297.1113(11) | 3.2544(22) | 0.0971(17) | −0.00294(44) | ⋯ | ⋯ | ⋯ | ⋯ | ⋯ |
CH3N13CO | V127: | 0.440 | 30 | 0 | 0.843 | 4283.37801(40) | 3.23616(18) | [0.0971] | [−0.00294] | ⋯ | ⋯ | ⋯ | ⋯ | ⋯ | |
13CH3NCO | V127: | 0.435 | 26 | 1 | 1.333 | 4179.1157(15) | 3.0893(21) | 0.08971(90) | [−0.00294] | ⋯ | ⋯ | ⋯ | ⋯ | ⋯ | |
CH3NCO | 1U | V132: | 0.365 | 36 | 3 | 0.969 | 4377.6225(11) | 4.2356(24) | 0.0116(19) | 0.00223(53) | ⋯ | ⋯ | ⋯ | ⋯ | ⋯ |
CH3N13CO | V132: | 0.432 | 31 | 0 | 1.070 | 4363.45460(50) | 4.20678(24) | [0.0116] | [0.00223] | ⋯ | ⋯ | ⋯ | ⋯ | ⋯ | |
13CH3NCO | V132: | 0.424 | 26 | 0 | 1.195 | 4255.9987(14) | 4.0113(20) | 0.01325(92) | [0.00223] | ⋯ | ⋯ | ⋯ | ⋯ | ⋯ |
Notes.
aThe value of the Ka or K quantum number following the notation described in Cernicharo et al. (2016). bThe identifier of the line sequence as defined in Cernicharo et al. (2016). cIntensity of the sequence relative to the strongest sequence in the spectrum. dThe number of lines in the linear fit. eThe number of confidently assigned lines that were perturbed and were rejected from the fit. fUnitless (weighted) deviation of the fit. gSquare brackets represent the value fixed to that for the parent species.Table 11. JPL Catalog Line List for CH3N13CO
Frequency | Error | Log(Int)a | DRb | Elowc | guppd | TAGe | QNFMTf | QN'g | QN''h | ||||||||
---|---|---|---|---|---|---|---|---|---|---|---|---|---|---|---|---|---|
(MHz) | (MHz) | (cm−1) | J' |
![]() |
![]() |
![]() |
![]() |
J'' |
![]() |
![]() |
m'' |
![]() |
|||||
60492.530 | 0.0500 | −5.0017 | 3 | 6.0737 | 15 | 01 | 505 | 7 | 0 | 7 | 0 | 0 | 6 | 0 | 6 | 0 | 0 |
69130.253 | 0.0500 | −4.8323 | 3 | 8.0980 | 17 | 01 | 505 | 8 | 0 | 8 | 0 | 0 | 7 | 0 | 7 | 0 | 0 |
77766.490 | 0.0500 | −4.6840 | 3 | 10.4114 | 19 | 01 | 505 | 9 | 0 | 9 | 0 | 0 | 8 | 0 | 8 | 0 | 0 |
86400.869 | 0.0500 | −4.5525 | 3 | 13.0138 | 21 | 01 | 505 | 10 | 0 | 10 | 0 | 0 | 9 | 0 | 9 | 0 | 0 |
95033.255 | 0.0500 | −4.4348 | 3 | 15.9051 | 23 | 01 | 505 | 11 | 0 | 11 | 0 | 0 | 10 | 0 | 10 | 0 | 0 |
103663.458 | 0.0500 | −4.3284 | 3 | 19.0854 | 25 | 01 | 505 | 12 | 0 | 12 | 0 | 0 | 11 | 0 | 11 | 0 | 0 |
Notes. The key parameters used in the evaluation of this table, μ = μa = 2.882 D, T = 300 K, and Qvr = 138,369, were taken from the parent species. See Sections A.1 and A.2 in Cernicharo et al. (2016) for details.
aBase-10 logarithm of the integrated intensity at 300 K in units of nm2 MHz. bDegrees of freedom in the rotational partition function. cUpper-state degeneracy. dEnergy of the lower level taken from the parent species from Cernicharo et al. (2016). eSpecies tag or molecular identifier. fFormat of the quantum numbers. gQuantum numbers for the upper state. Ka and Kc quantum numbers are defined only for m = 0 state. For m > 0: Ka = K and Kc = 0. hQuantum numbers for the lower state. Ka and Kc quantum numbers are defined only for m = 0 state. For m > 0: Ka = K and Kc = 0.Only a portion of this table is shown here to demonstrate its form and content. A machine-readable version of the full table is available.
Download table as: DataTypeset image
Table 12. JPL Catalog Line List for 13CH3NCO
Frequency | Error | Log(Int)a | DRb | Elowc | guppd | TAGe | QNFMTf | QN'g | QN''h | ||||||||
---|---|---|---|---|---|---|---|---|---|---|---|---|---|---|---|---|---|
(MHz) | (MHz) | (cm−1) | J' |
![]() |
![]() |
![]() |
![]() |
J'' |
![]() |
![]() |
m'' |
![]() |
|||||
59010.994 | 0.0500 | −5.0017 | 3 | 6.0737 | 15 | 01 | 505 | 7 | 0 | 7 | 0 | 0 | 6 | 0 | 6 | 0 | 0 |
67437.329 | 0.0500 | −4.8323 | 3 | 8.0980 | 17 | 01 | 505 | 8 | 0 | 8 | 0 | 0 | 7 | 0 | 7 | 0 | 0 |
75862.078 | 0.0500 | −4.6840 | 3 | 10.4114 | 19 | 01 | 505 | 9 | 0 | 9 | 0 | 0 | 8 | 0 | 8 | 0 | 0 |
84285.279 | 0.0500 | −4.5525 | 3 | 13.0138 | 21 | 01 | 505 | 10 | 0 | 10 | 0 | 0 | 9 | 0 | 9 | 0 | 0 |
92706.543 | 0.0500 | −4.4348 | 3 | 15.9051 | 23 | 01 | 505 | 11 | 0 | 11 | 0 | 0 | 10 | 0 | 10 | 0 | 0 |
101125.795 | 0.0500 | −4.3284 | 3 | 19.0854 | 25 | 01 | 505 | 12 | 0 | 12 | 0 | 0 | 11 | 0 | 11 | 0 | 0 |
Notes. The key parameters used in the evaluation of this table, μ = μa = 2.882 D, T = 300 K, and Qvr = 138,369, were taken from the parent species. See Sections A.1 and A.2 in Cernicharo et al. (2016) for details.
aBase-10 logarithm of the integrated intensity at 300 K in units of nm2 MHz. bDegrees of freedom in the rotational partition function. cUpper-state degeneracy. dEnergy of the lower level taken from the parent species from Cernicharo et al. (2016). eSpecies tag or molecular identifier. fFormat of the quantum numbers. gQuantum numbers for the upper state. Ka and Kc quantum numbers are defined only for m = 0 state. For m > 0: Ka = K and Kc = 0. hQuantum numbers for the lower state. Ka and Kc quantum numbers are defined only for m = 0 state. For m > 0: Ka = K and Kc = 0.Only a portion of this table is shown here to demonstrate its form and content. A machine-readable version of the full table is available.
Download table as: DataTypeset image
Evaluation of transition intensities is critically dependent on the value used for the vibration–rotation partition function qvr. We tested for the possible isotopic changes to qvr, but found those to be small and for simplicity decided to use the recommended parent species function from Table A.4 of Cernicharo et al. (2016). Isotopic changes result from changes in rotational constants and changes in the vibrational energy distribution, both of which would be most marked for 13CH3NCO. On repeating the assumptions used in evaluating the recommended partition function for the parent species, we find that the 300 K value of qvr would be greater by 3.3% for 13CH3NCO but by less than 0.4% for CH3N13CO. The distribution of internal rotation substates in this very low barrier case is rather close to the free internal rotation limit of (Lin & Swalen 1959). The internal rotation F parameter is estimated to be near 8.3 cm−1 and would be lower by ~0.019 cm −1 in 13CH3NCO and 0.001 cm−1 for CH3N13CO. This would result in only negligible changes in internal rotor energies, which would increase in proportion to m2 and be equal to 0.68 cm−1 for the highest considered m = 6 substate in 13CH3NCO. Finally, anharmonic ab initio calculations show that the fundamental wavenumber of the CNC bending mode decreases by 1.4 cm −1 for 13CH3NCO and 0.4 cm −1 for CH3N13CO. All of these changes are deemed insignificant in relation to the assumptions typically made in partition function evaluation, as discussed in detail in Cernicharo et al. (2016).
4. Conclusions
A detailed rotational study of interstellar CH3NCO is reported on the basis of Stark-modulation and frequency-modulation microwave and millimeter-wave techniques. Around 2500 new transitions were assigned and measured for the parent isotopic species of CH3NCO, extending the coverage of its rotational spectrum up to K = 10. In addition, more than 2400 K ≤ 3 transitions were identified for the CH3N13CO and 13CH3NCO isotopic species. The transition frequencies reported in Tables 2, 4, 6, and 7, as well as in the JPL catalog line lists (Tables 5, 11, and 12), are almost all actual experimental frequencies. Only in exceptional cases of a frequency measurement missing for technical reasons or due to line blending were the power expansion fits from Tables 1, 3, and 10 used to interpolate between the experimentally measured lines. Although some of the expansion fits may show useful predictive behavior outside the present data region, such extrapolations would only be deemed moderately safe for the most well-behaved line sequences. The criteria for this would be the shortest number of expansion terms in fits based on Equation (1) and where the effective value of the DJ parameter is close to the 2 kHz magnitude determined in the actual joint K = 0, 1, 2 fits in Table 8. Significant deviation from these conditions indicates increased curvature of the line sequence resulting from considerable vibration–rotation coupling, and in such cases extrapolation is not recommended. In summary, for the extremely challenging spectroscopic case of the CH3NCO molecule all transitions considered in future interstellar detections should be checked against the actual experimental measured laboratory frequencies. The line lists compiled in this work provide a firm basis for further astrophysical searches of CH3NCO and make it possible to account for a considerable number of additional U-lines in the current sensitive broadband surveys.
The funding from Ministerio de Ciencia e Innovación (Consolider-Ingenio 2010 CSD2009-00038 program "ASTROMOL," CTQ2013-40717-P and CTQ2016-76393-P), Junta de Castilla y León (VA077U16), and European Research Council under the European Union's Seventh Framework Programme (FP/2007-2013)/ERC-2013-SyG, grant agreement No. 610256 NANOCOSMOS, is gratefully acknowledged. E.R.A. thanks Ministerio de Ciencia e Innovación for FPI grant (BES-2014-067776). J.C.G. thanks the Program PCMI (INSU-CNRS) and the Centre National d'Etudes Spatiales (CNES) for funding support. L.K. thanks The Czech Science Foundation (GAČR) for financial support for this work (grant 19-25116Y).