Abstract
We present the results of our Atacama Large Millimeter Array HCN J = 3–2 and HCO+ J = 3–2 line observations of a uniformly selected sample (>25) of nearby ultraluminous infrared galaxies (ULIRGs) at z < 0.15. The emission of these dense molecular gas tracers and continuum are spatially resolved in the majority of observed ULIRGs for the first time with achieved synthesized beam sizes of ≲02 or ≲500 pc. In most ULIRGs, the HCN-to-HCO+ J = 3–2 flux ratios in the nuclear regions within the beam size are systematically higher than those in the spatially extended regions. The elevated nuclear HCN J = 3–2 emission could be related to (a) luminous buried active galactic nuclei, (b) the high molecular gas density and temperature in ULIRG's nuclei, and/or (c) mechanical heating by spatially compact nuclear outflows. A small fraction of the observed ULIRGs display higher HCN-to-HCO+ J = 3–2 flux ratios in localized off-nuclear regions than those of the nuclei, which may be due to mechanical heating by spatially extended outflows. The observed nearby ULIRGs are generally rich in dense (>105 cm−3) molecular gas, with an estimated mass of >109 M⊙ within the nuclear (a few kpc) regions, and dense gas can dominate the total molecular mass there. We find a low detection rate (<20%) regarding the possible signature of a vibrationally excited (v2 = 1f) HCN J = 3–2 emission line in the vicinity of the bright HCO+ J = 3–2 line that may be due, in part, to the large molecular line widths of ULIRGs.
Export citation and abstract BibTeX RIS
1. Introduction
Ultraluminous infrared galaxies (ULIRGs) emit very strong infrared (IR) radiation, with infrared (8–1000 μm) luminosities of LIR > 1012 L⊙. ULIRGs are mostly found in gas-rich major galaxy mergers in the nearby universe at z < 0.15 (e.g., Sanders et al. 1988; Clements et al. 1996; Murphy et al. 1996; Duc et al. 1997). The large observed infrared luminosities indicate the presence of luminous energy sources; however, these sources may be hidden and/or surrounded by dust. Numerical simulations of gas-rich major galaxy mergers predict not only that active star formation (a starburst) is triggered but also that existing supermassive black holes (SMBHs) can achieve high mass accretion rates and become luminous active galactic nuclei (AGNs); both are likely to occur in heavily dust-obscured regions (Hopkins et al. 2006). It is important to test this widely accepted scenario observationally if we are to understand the nature of the ULIRG population in the local universe (Sanders et al. 1988; Alexander & Hickox 2012).
For this purpose, it is necessary to conduct observations using wavelengths of low dust extinction, given that the ULIRG energy sources, particularly AGNs, are buried deep in the nuclear gas and dust. Dust extinction effects are much reduced in the infrared and hard X-ray range, compared to the optical range. Spectroscopic investigations of such buried AGNs in nearby ULIRGs have been undertaken using an infrared range of 2.5–35 μm (e.g., Genzel et al. 1998; Imanishi & Dudley 2000; Soifer et al. 2002; Imanishi et al. 2006a, 2007a, 2008, 2010a, 2010b; Imanishi 2006, 2009; Armus et al. 2007; Nardini et al. 2008, 2009, 2010; Veilleux et al. 2009; Lee et al. 2012; Ichikawa et al. 2014; Alonso-Herrero et al. 2016) and a hard X-ray range of >10 keV (e.g., Teng et al. 2015; Iwasawa et al. 2017; Oda et al. 2017; Ricci et al. 2017). This is because AGNs and starbursts display different relative strengths of polycyclic aromatic hydrocarbon emission features and dust continuum emission in the infrared and also have different hard X-ray to bolometric luminosity ratios. In fact, these observations have revealed optically elusive, but intrinsically luminous, buried AGNs in many nearby ULIRGs. However, there remains a large fraction of nearby ULIRGs for which no clear AGN signature has been detected in infrared and hard X-ray surveys. It remains unclear whether the nondetection of AGN signatures in these sources is due to the fact that (a) AGNs are not sufficiently luminous (i.e., mass accretion rates onto SMBHs are not so high) to be detectable relative to strong starbursts, or (b) luminous AGNs are present but are too highly obscured to be detectable, even in the infrared and X-ray range. Observations at wavelengths of even stronger dust/gas penetration power may reveal extremely deeply buried AGNs (τ > 1 at infrared 2.5–35 μm and X-ray ∼10 keV) in nearby ULIRGs.
(Sub)millimeter wavelengths at 0.3–3.6 mm (84–950 GHz) are of particular interest in this regard. If the Galactic extinction curve by gas and dust is assumed, the extinction is a factor of >30 smaller than those at infrared ∼20 μm and X-ray ∼10 keV (Hildebrand 1983). With the Atacama Large Millimeter Array (ALMA), it is now possible to conduct high-sensitivity and high-spatial-resolution observations of nearby ULIRGs in this potentially powerful (sub)millimeter wavelength range. As the energy-generation mechanisms are different between a starburst and an AGN (nuclear fusion versus a mass-accreting SMBH, respectively), the physical/chemical effects to the surrounding molecular gas are expected to differ between the two energy sources. Rotational J-transition lines of many molecules reside in the (sub)millimeter wavelength range, and multiple molecular line flux ratios can differ depending on the primary energy sources. In fact, (sub)millimeter molecular rotational J-transition line flux ratios have been used to separate AGNs from starbursts in nearby optically identified luminous AGNs and starburst galaxies (e.g., Kohno 2005; Krips et al. 2008). (Sub)millimeter molecular line observations have been applied also to a limited number of nearby ULIRGs, using pre-ALMA observing facilities and the early phase of ALMA. Some fraction of ULIRGs have been diagnosed to contain optically elusive, but infrared-detectable, luminous buried AGNs. A trend was found that such ULIRGs displayed different molecular rotational J-transition line flux ratios from starburst-dominated galaxies, although a small fraction of infrared-classified starbursts showed similar molecular line flux ratios to AGN-important galaxies (e.g., Imanishi et al. 2004, 2006b, 2007b, 2009, 2016c, 2018; Costagliola et al. 2011; Imanishi & Nakanishi 2014; Privon et al. 2015; Izumi et al. 2016). Compared to old single dish telescope data, the trend has been seen more clearly in recent interferometric data, most likely because (a) only nuclear molecular gas significantly affected by luminous AGNs is selectively probed (with reduced contaminations from spatially extended starburst-dominated emission), and (b) multiple molecular line data were taken simultaneously (which minimized possible systematic uncertainties in molecular line flux ratios).
Based on sensitive ALMA studies, a few candidates of optically/infrared/X-ray-elusive but (sub)millimeter-detectable extremely deeply buried AGNs have been found (Imanishi et al. 2016c, 2018). This has been accomplished (a) by finding molecular rotational J-transition line flux ratios (at the vibrational ground level; v = 0) that are usually seen in AGN-important galaxies and (b) by detecting molecular J-transition lines at a vibrationally excited (v2 = 1, l = 1f; hereafter v2 = 1f) level. The latter detection can be the signature of a luminous buried AGN, particularly if the vibrationally excited (v2 = 1f) molecular line luminosity (relative to vibrational ground (v = 0) molecular line and/or infrared luminosity), is significantly higher than those seen in active star-forming regions (Aalto et al. 2015a, 2015b; Imanishi et al. 2016c, 2018). This is because (a) molecular vibrational excitation is most likely to be caused by mid-infrared (5–25 μm) radiative pumping (Sakamoto et al. 2010), and (b) the mid-infrared to bolometric luminosity ratio in an AGN can be much higher than a starburst, due to AGN-heated hot dust emission. However, these molecular line observations of nearby ULIRGs have only been conducted for arbitrarily selected interesting sources with known luminous AGN signatures at other wavelengths and/or some other unique observational properties. Target selection has been non-uniform, hampering any statistical discussion about the nature of the nearby ULIRG population in general. Specifically, it is still unclear (a) whether ULIRGs with infrared-identified luminous buried AGNs consistently show molecular line flux ratios expected for AGN-important galaxies and (b) what fraction of nearby ULIRGs contain infrared/X-ray-elusive, but (sub)millimeter-detectable, extremely deeply buried AGNs (τ > 1 at 2.5–35 μm and ∼10 keV). It is a natural next step to apply the powerful (sub)millimeter molecular line method to an extended number of carefully and uniformly selected nearby ULIRG samples, using the highly sensitive ALMA.
In this paper, we present the results of ALMA molecular line observations of an increasing number of nearby ULIRGs. Molecular line and continuum emission were spatially resolved in the majority of observed ULIRGs, allowing for the first time detailed and systematic investigations of the internal spatial variation of molecular line flux ratios in a statistically significant number of the nearby ULIRG population. Throughout this paper, we adopt H0 = 71 km s−1 Mpc−1, ΩM = 0.27, and ΩΛ = 0.73. Unless otherwise stated, "molecular line flux ratio" refers to the "rotational J-transition line flux ratio at the vibrational ground level (v = 0)."
2. Selected Targets and Molecular Lines
Our targets are nearby ULIRGs in the Infrared Astronomical Satellite (IRAS) 1 Jy sample (Kim & Sanders 1998). Optical spectroscopic classifications (Veilleux et al. 1999; Yuan et al. 2010), as well as infrared spectroscopic energy diagnostics (a) at 5–35 μm using Spitzer InfraRed Spectrograph (IRS; e.g., Imanishi et al. 2007a, 2010a; Nardini et al. 2008, 2009, 2010; Imanishi 2009; Veilleux et al. 2009) and (b) at 2.5–5 μm using AKARI Infrared Camera (IRC; e.g., Imanishi et al. 2008, 2010b), have extensively been conducted for this sample. We selected ULIRGs classified optically as Low Ionization Nuclear Emission-line Regions (LINERs) or H ii regions (i.e., no obvious optical AGN signature; Veilleux et al. 1999) at z < 0.15 and at decl. <+20° to be best observable from the ALMA site in Chile. Our primary goal was to unveil optically and/or infrared/X-ray-elusive, but intrinsically luminous, buried AGNs in nearby ULIRGs, based on the proposed powerful (sub)millimeter approach.
In nearby ULIRGs, which are mostly gas-rich major galaxy mergers, molecular gas is concentrated in the nuclear regions (e.g., Downes & Solomon 1998; Evans et al. 2002; Pereira-Santaella et al. 2018), and gas density becomes high there (Scoville et al. 2015). Observations using dense gas tracers are crucial to understanding the physical properties of molecular gas in nearby ULIRGs (Gao & Solomon 2004a, 2004b). HCN and HCO+ rotational J-transition lines are better probes of such dense molecular gas, due to larger dipole moments, than the widely used bright CO low-J (J = 1–0 or J = 2–1) transition lines (Shirley 2015). Furthermore, the trend of higher HCN-to-HCO+ line flux ratios in AGNs than in starbursts have been argued mostly in recent high-quality interferometric data (where HCN and HCO+ lines were observed simultaneously), at J = 1–0 (Kohno 2005; Imanishi et al. 2006b, 2007b, 2009; Krips et al. 2008), J = 3–2 (Krips et al. 2008; Imanishi et al. 2016a, 2016c), and J = 4–3 (Izumi et al. 2013, 2015, 2016; Garcia-Burillo et al. 2014; Imanishi & Nakanishi 2014; Imanishi et al. 2018). Thus, observing both HCN and HCO+ lines can be an effective way to disentangle the energetic role of AGNs and starbursts.
Our next step was to select ULIRGs with modestly bright dense molecular emission lines from the nuclear regions, where the effects of the putative AGNs to molecular line flux ratios are expected to be strong. Regarding the nuclear buried energy sources of ULIRGs, dense molecular emission line luminosity is expected to correlate with infrared dust emission luminosity (Gao & Solomon 2004a, 2004b), regardless of whether the energy sources are stars and/or AGNs, because both dense molecular gas and spatially coexisting dust are heated and produce dense molecular line and infrared dust continuum emission, respectively. Although the infrared luminosities of ULIRGs are usually derived from IRAS's large-aperture (>60'') measurements (Table 1), the bulk of the infrared luminosities in nearby ULIRGs originate in nuclear (<a few kpc) regions, with small contributions from spatially extended (>3 kpc) star formation in the host galaxies (Soifer et al. 2000; Diaz-Santos et al. 2010; Imanishi et al. 2011). Namely, ULIRGs with high IRAS-based infrared fluxes strongly suggest high nuclear infrared dust continuum emission fluxes and thereby high nuclear dense molecular emission line fluxes (Imanishi & Nakanishi 2014).
Table 1. Basic Properties of the Observed Ultraluminous Infrared Galaxies
Object | Redshifta | dL | Scale | f12 | f25 | f60 | f100 | log LIR | log LFIR | Optical | IR-AGN |
---|---|---|---|---|---|---|---|---|---|---|---|
(Mpc) | (kpc/'') | (Jy) | (Jy) | (Jy) | (Jy) | (L⊙) | (L⊙) | Class | Fraction [%] | ||
(1) | (2) | (3) | (4) | (5) | (6) | (7) | (8) | (9) | (10) | (11) | (12) |
IRAS 00188−0856 | 0.1285 (0.128) | 596 | 2.3 | <0.12 | 0.37 | 2.59 | 3.40 | 12.4 | 12.3 | LINER | 35 ± 4 |
IRAS 03250+1606 | 0.1286 (0.129) | 596 | 2.3 | <0.10 | <0.15 | 1.38 | 1.77 | 12.1 | 12.0 | LINER | <0.2 |
IRAS 04103−2838 | 0.118 | 543 | 2.1 | 0.08 | 0.54 | 1.82 | 1.71 | 12.2 | 12.0 | LINER | 5.4 ± 0.8 |
IRAS 09039+0503 | 0.1257b | 578 | 2.2 | 0.07 | 0.12 | 1.48 | 2.06 | 12.1 | 12.0 | LINER |
![]() |
IRAS 10378+1108 | 0.1365 (0.136) | 636 | 2.4 | <0.11 | 0.24 | 2.28 | 1.82 | 12.3 | 12.2 | LINER | 14 ± 2 |
IRAS 10485−1447 | 0.133 | 619 | 2.3 | <0.11 | 0.25 | 1.73 | 1.66 | 12.2 | 12.1 | LINER | 5.7 ± 1.0 |
IRAS 11095−0238 | 0.1066c | 484 | 1.9 | 0.06 | 0.42 | 3.25 | 2.53 | 12.3 | 12.1 | LINER | 60 ± 4 |
IRAS 13335−2612 | 0.125d | 578 | 2.2 | <0.13 | <0.14 | 1.40 | 2.10 | 12.1 | 12.0 | LINER | <0.03 |
IRAS 14348−1447 | 0.083e | 373 | 1.5 | 0.07 | 0.49 | 6.87 | 7.07 | 12.3 | 12.3 | LINER | 3.9 ± 1.1 |
IRAS 16090−0139 | 0.1334 (0.134) | 621 | 2.4 | 0.09 | 0.26 | 3.61 | 4.87 | 12.6 | 12.5 | LINER | 24 ± 3 |
IRAS 21329−2346 | 0.125 | 578 | 2.2 | 0.05 | 0.12 | 1.65 | 2.22 | 12.2 | 12.1 | LINER |
![]() |
IRAS 23234+0946 | 0.128 | 593 | 2.3 | <0.06 | 0.08 | 1.56 | 2.11 | 12.1 | 12.1 | LINER |
![]() |
IRAS 00091−0738 | 0.118 | 543 | 2.1 | <0.07 | 0.22 | 2.63 | 2.52 | 12.3 | 12.2 | H ii | 58 ± 6 |
IRAS 00456−2904 | 0.110 | 504 | 2.0 | <0.08 | 0.14 | 2.60 | 3.38 | 12.2 | 12.1 | H ii | <0.05 |
IRAS 01004−2237 | 0.118 | 543 | 2.1 | 0.11 | 0.66 | 2.29 | 1.79 | 12.3 | 12.1 | H ii | 50 ± 4 |
IRAS 01166−0844 | 0.1172 (0.118) | 539 | 2.1 | 0.07 | 0.17 | 1.74 | 1.42 | 12.1 | 12.0 | H ii |
![]() |
IRAS 01298−0744 | 0.1368 (0.136) | 638 | 2.4 | <0.12 | 0.19 | 2.47 | 2.08 | 12.4 | 12.3 | H ii |
![]() |
IRAS 01569−2939 | 0.1402 (0.141) | 655 | 2.5 | <0.11 | 0.14 | 1.73 | 1.51 | 12.3 | 12.1 | H ii | 18 ± 3 |
IRAS 02411+0353 | 0.144 | 676 | 2.5 | <0.08 | 0.22 | 1.37 | 1.95 | 12.3 | 12.1 | H ii | <0.9 |
IRAS 10190+1322 | 0.0762 (0.077) | 341 | 1.4 | <0.07 | 0.38 | 3.33 | 5.57 | 12.0 | 12.0 | H ii | <0.02 |
IRAS 11506+1331 | 0.127 | 588 | 2.2 | <0.10 | 0.19 | 2.58 | 3.32 | 12.4 | 12.3 | H ii |
![]() |
IRAS 13509+0442 | 0.1365 (0.136) | 636 | 2.4 | 0.10 | <0.23 | 1.56 | 2.53 | 12.3 | 12.2 | H ii | <0.03 |
IRAS 22206−2715 | 0.132 | 614 | 2.3 | <0.10 | <0.16 | 1.75 | 2.33 | 12.2 | 12.1 | H ii | <0.5 |
IRAS 12112+0305f | 0.0730 | 326 | 1.4 | 0.12 | 0.51 | 8.50 | 9.98 | 12.3 | 12.3 | LINER | <0.7 |
IRAS 20414−1651f | 0.0870 (0.086) | 392 | 1.6 | <0.65 | 0.35 | 4.36 | 5.25 | 12.3 | 12.1 | H ii | <0.1 |
IRAS 22491−1808f | 0.0776 (0.076) | 347 | 1.5 | 0.05 | 0.55 | 5.44 | 4.45 | 12.2 | 12.1 | H ii | <0.07 |
Notes. Column (1): object name. Column (2): redshift. Column (3): luminosity distance (in Mpc). Column (4): physical scale (in kpc arcsec−1). Columns (5)–(8): f12, f25, f60, and f100 are IRAS fluxes at 12 μm, 25 μm, 60 μm, and 100 μm, respectively, taken from Kim & Sanders (1998). Column (9): decimal logarithm of infrared (8–1000 μm) luminosity in units of solar luminosity (L⊙), calculated with LIR = 2.1 × 1039 × D(Mpc)2 × (13.48 × f12 + 5.16 × f25 + 2.58 × f60 + f100) (erg s−1) (Sanders 1996). Column (10): decimal logarithm of far-infrared (40–500 μm) luminosity in units of solar luminosity (L⊙), calculated with LFIR = 2.1 × 1039 × D(Mpc)2 × (2.58 × f60 + f100) (erg s−1) (Sanders 1996). Column (11): optical spectroscopic classification by Veilleux et al. (1999). "LINER" and "H ii" refer to LINER and H ii-region, respectively. Column (12): infrared spectroscopically estimated bolometric contribution of AGNs (in %) by Nardini et al. (2010).
aFor some fraction of sources, the observed peak frequencies of the detected HCN J = 3–2 and HCO+ J = 3–2 emission lines in our ALMA spectra are significantly offset from the expected frequencies from optically derived redshifts (Kim & Sanders 1998). For them, our ALMA molecular line redshifts are adopted, and the optically derived redshifts are shown in parentheses. bThis is the redshift of the southwest nucleus. The redshift of the northeast nucleus is z = 0.125. cThis is the redshift of the northeast nucleus. The redshift of the southwest nucleus is z = 0.106. dThis is the redshift of the north nucleus. The redshift of the south nucleus is z = 0.1257. eThis is the redshift of the southwest nucleus. The redshift of the northeast nucleus is z = 0.0825. fObserved in ALMA Cycle 2 or 3 and presented by Imanishi et al. (2016c).Download table as: ASCIITypeset image
Next, we determined which rotational J-transition lines should be observed. Historically, HCN and HCO+ J = 1–0 lines have long been used to diagnose energy sources (e.g., Imanishi et al. 2004, 2006b, 2007b, 2009; Kohno 2005; Krips et al. 2008; Costagliola et al. 2011; Privon et al. 2015). However, using ALMA, the J = 1–0 lines can be observed only for nearby sources at z < 0.05; above this redshift, the lines are redshifted beyond the frequency coverage of band 3 (84–116 GHz), the longest wavelength band of the current ALMA. Many interesting nearby ULIRGs in the IRAS 1 Jy sample are at z > 0.05 (Kim & Sanders 1998).
Higher J-transition lines at higher frequencies (shorter wavelengths) can be covered with ALMA for higher-redshift sources. More importantly, higher J-transition lines probe denser and warmer molecular gas, due to the higher critical density and excitation energy level, than lower J-transition lines (Shirley 2015). Given that dense, warm molecular gas locates preferentially in the nuclear regions of galaxies, molecular emission line flux ratios at higher J should be more sensitive to the properties of molecular gas in close vicinity to the ULIRG's nuclear primary energy sources (<a few kpc), with small contaminations from low-density and cool molecular gas in the spatially extended (>3 kpc) host galaxies. Given that the J = 2–1 lines of HCN and HCO+ for nearby sources at z < 0.15 were not observable before ALMA Cycle 4 (due to the unavailability of band 5 (163–211 GHz) at that time), we started our dense molecular line observations of nearby ULIRGs at J = 4–3 (Imanishi & Nakanishi 2014; Izumi et al. 2016; Imanishi et al. 2018) and J = 3–2 (Imanishi et al. 2016c). We decided to expand our HCN and HCO+ line survey of nearby ULIRGs at J = 3–2 in band 6 (211–275 GHz) for the following reasons. First, due to fewer requirements for weather conditions in band 6, the chance of program execution is higher than that in band 7 (275–373 GHz) where J = 4–3 lines of HCN and HCO+ are observable for nearby sources. Second, at J = 3–2, in addition to the widely used HCN and HCO+ rotational J-transition lines at the vibrational ground level (v = 0), we can simultaneously cover the vibrationally excited (v2 = 1f) emission lines of both HCN and HCO+ in ALMA band 6, which can provide additional signatures of luminous buried AGNs surrounded by a large column density of dense molecular gas and dust (Section 1).
Assuming a fixed HCN J = 3–2 to J = 1–0 flux ratio, we ordered ULIRGs in the IRAS 1 Jy sample at z < 0.15 and decl. <+20° from the highest infrared flux. We selected 26 sources with infrared fluxes above a certain threshold, which are summarized in Table 1. Of these, HCN J = 3–2 and HCO+ J = 3–2 emission line data of three ULIRGs (IRAS 12112+0305, IRAS 20414−1651, and IRAS 22491−1808) have been obtained during ALMA Cycle 2 or 3 (Imanishi et al. 2016c). We thus observed the remaining 23 ULIRGs in Cycle 5. These 26 ULIRGs were selected solely based on the infrared flux (or expected nuclear HCN J = 3–2 emission line flux). This flux-limited sample was complete and unbiased with respect to the energetic importance of optically elusive, but infrared-detected, buried AGNs.
3. Observations and Data Analyses
Our HCN J = 3–2 and HCO+ J = 3–2 observations of 23 ULIRGs in band 6 (211–275 GHz) were conducted using our ALMA Cycle 5 program 2017.1.00057.S (PI = M. Imanishi). The observation details are summarized in Table 2. Observations of the majority of the ULIRGs were conducted in 2017 December with the longest baseline of >2500 m, excluding IRAS 21329−2346 and IRAS 22206−2715, whose observations were made in 2018 April with the ∼500 m longest baseline. We adopted the widest 1.875 GHz width mode and three spectral windows to cover HCN J = 3–2 (νrest = 265.886 GHz), HCO+ J = 3–2 (νrest = 267.558 GHz) and HCN v2 = 1f J = 3–2 (νrest = 267.199 GHz), and HCO+ v2 = 1f J = 3–2 (νrest = 268.689 GHz) lines simultaneously in each of the windows.
Table 2. Log of Our ALMA Cycle 5 Observations
Object | Date | Antenna | Baseline | Integration | Calibrator | ||
---|---|---|---|---|---|---|---|
(UT) | Number | (m) | (minutes) | Bandpass | Flux | Phase | |
(1) | (2) | (3) | (4) | (5) | (6) | (7) | (8) |
IRAS 00188−0856 | 2017 Dec 17 | 45 | 15–2517 | 36 | J0006−0623 | J0006−0623 | J0017−0512 |
IRAS 03250+1606 | 2017 Dec 10 | 46 | 15–3321 | 39 | J0238+1636 | J0238+1636 | J0309+1029 |
IRAS 04103−2838 | 2017 Dec 9 | 44 | 15–3083 | 15 | J0522−3627 | J0522−3627 | J0348−2749 |
IRAS 09039+0503 | 2017 Dec 23 | 48 | 15–2517 | 22 | J0854+2006 | J0854+2006 | J0914+0245 |
IRAS 10378+1108 | 2017 Dec 17 | 45 | 15–2517 | 28 | J1058+0133 | J1058+0133 | J1025+1253 |
IRAS 10485−1447 | 2017 Dec 11 | 44 | 15–3321 | 18 | J1037−2934 | J1037−2934 | J1048−1909 |
IRAS 11095−0238 | 2017 Dec 11 | 44 | 15–3321 | 12 | J1229+0203 | J1229+0203 | J1058+0133 |
IRAS 13335−2612 | 2017 Dec 23 | 42 | 15–2517 | 21 | J1337−1257 | J1337−1257 | J1351−2912 |
IRAS 14348−1447 | 2017 Dec 12 | 44 | 15–3321 | 8 | J1517−2422 | J1517−2422 | J1445−1629 |
IRAS 16090−0139 | 2017 Dec 16 | 43 | 15–2517 | 8 | J1517−2422 | J1517−2422 | J1557−0001 |
IRAS 21329−2346 | 2018 Apr 12 | 44 | 15–500 | 21 | J2258−2758 | J2258−2758 | J2101−2933 |
IRAS 23234+0946 | 2017 Dec 10 | 44 | 15–2933 | 36 | J2253+1608 | J2253+1608 | J2327+0940 |
IRAS 00091−0738 | 2017 Dec 16 | 45 | 15–3083 | 16 | J2258−2758 | J2258−2758 | J0006−0623 |
IRAS 00456−2904 | 2017 Dec 16 | 45 | 15–3083 | 12 | J2258−2758 | J2258−2758 | J0038−2459 |
IRAS 01004−2237 | 2017 Dec 16 | 45 | 15–3083 | 12 | J0006−0623 | J0006−0623 | J0117−2111 |
IRAS 01166−0844 | 2017 Dec 9–10 | 45 | 15–3321 | 16 | J0006−0623 | J0006−0623 | J0116−1136 |
IRAS 01298−0744 | 2017 Dec 10 | 45 | 15–3321 | 24 | J0006−0623 | J0006−0623 | J0141−0928 |
IRAS 01569−2939 | 2017 Dec 10 | 46 | 15–3321 | 21 | J0006−0623 | J0006−0623 | J0120−2701 |
IRAS 02411+0353 | 2017 Dec 9 | 47 | 15–3321 | 22 | J0238+1636 | J0238+1636 | J0239+0416 |
IRAS 10190+1322 | 2017 Dec 23 | 45 | 15–2517 | 9 | J1058+0133 | J1058+0133 | J1025+1253 |
IRAS 11506+1331 | 2017 Dec 15 | 45 | 15–2517 | 20 | J1229+0203 | J1229+0203 | J1157+1638 |
IRAS 13509+0442 | 2017 Dec 17 | 44 | 15–2517 | 26 | J1256−0547 | J1256−0547 | J1359+0159 |
IRAS 22206−2715 | 2018 Apr 12 | 44 | 15–500 | 25 | J2148+0657 | J2148+0657 | J2258−2758 |
IRAS 12112+0305a | 2015 May 14 | 37 | 23–558 | 5 | J1058+0133 | Ganymede | J1229+0203 |
IRAS 20414−1651a | 2016 Mar 26 | 38 | 15–460 | 11 | J1924−2914 | Pallas | J2011−1546 |
IRAS 22491−1808a | 2015 May 24 | 34 | 21–539 | 5 | J2258−2758 | Titan | J2056−4714 |
Notes. Column (1): object name. Column (2): observation date in UT. Column (3): number of antennas used for observations. Column (4): baseline length in meters. Minimum and maximum baseline lengths are shown. Column (5): net on source integration time in minutes. Columns (6), (7), and (8): bandpass, flux, and phase calibrator for the target source, respectively.
aObserved in ALMA Cycle 2 or 3.Download table as: ASCIITypeset image
We started our data reduction from calibrated data provided by ALMA, using the Common Astronomy Software Applications (CASA, https://casa.nrao.edu). Based on channels that did not show obvious emission and/or absorption lines, we determined the continuum level and subtracted it using the CASA task "uvcontsub." Then we applied the "clean" task (Briggs weighting; robust = 0.5 and gain = 0.1) for the continuum-only and continuum-subtracted molecular line data to create maps. The final velocity resolution was ∼20 km s−1. The pixel scales were 002 pixel−1 for the majority of the observed ULIRGs with a final synthesized beam size of 0
1–0
2 (150–530 pc), except IRAS 21329−2346 and IRAS 22206−2715, for which 0
04 pixel−1 was adopted due to the larger (0
6–0
8 or 1.3–1.7 kpc) synthesized beam sizes. According to the ALMA Cycle 5 Proposer's Guide, the maximum recoverable scale (MRS) is 1''–3'' (1.4–7.5 kpc) for sources with 0
1–0
2 synthesized beam sizes and 7''–9'' (15–21 kpc) for the two sources with 0
6–0
8 synthesized beam sizes. Diffuse continuum and molecular line emission with a spatial scale larger than the MRS can be missed in our ALMA data. The absolute flux calibration uncertainty of band 6 data is expected to be <10% in ALMA Cycle 5.
4. Results
Figure 1 and Table 3 show the continuum maps and detailed continuum emission properties, respectively. Significant continuum emission was detected from all sources, except IRAS 10485−1447 and IRAS 02411+0353. Multiple continuum emission components are clearly seen in the five ULIRGs IRAS 04103−2838 (Figure 1(c)), IRAS 09039+0503 (Figure 1(d)), IRAS 11095−0238 (Figure 1(f)), IRAS 13335−2612 (Figure 1(g)), and IRAS 14348−1447 (Figure 1(h)). Other specific comments on the continuum emission for several selected sources are provided in Appendices A and D.
Download figure:
Standard image High-resolution imageFigure 1. Continuum emission maps of ULIRGs with >3σ detection. The abscissa and ordinate are R.A. and decl. in International Celestial Reference System (ICRS), respectively. The black contours are 10σ, 20σ, and 30σ for IRAS 00188−0856; 4σ, 8σ, and 12σ for IRAS 03250+1606; 3σ and 4σ for IRAS 04103−2838; 4σ, 8σ, and 16σ for IRAS 09039+0503; 10σ, 20σ, and 30σ for IRAS 10378+1108; 4σ, 10σ, and 16σ for IRAS 11095−0238; 6σ and 20σ for IRAS 13335−2612; 4σ, 12σ, and 20σ for IRAS 14348−1447; 4σ, 8σ, and 16σ for IRAS 16090−0139; 3σ, 6σ, 12σ, and 18σ for IRAS 21329−2346; 4σ, 6σ, 12σ, and 18σ for IRAS 23234+0946; 12σ, 24σ, and 48σ for IRAS 00091−0738; 6σ, 14σ, and 22σ for IRAS 00456−2904; 4σ, 8σ, and 12σ for IRAS 01004−2237; 5σ, 10σ, and 15σ for IRAS 01166−0844 SE; 6σ, 18σ, and 54σ for IRAS 01298−0744; 4σ, 8σ, and 16σ for IRAS 01569−2939; 5σ, 10σ, and 15σ for IRAS 10190+1322; 4σ, 7σ, and 10σ for IRAS 11506+1331; 4σ, 8σ, and 12σ for IRAS 13509+0442; 7σ, 15σ, and 23σ for IRAS 22206−2715; 3σ, 10σ, 20σ, and 40σ for IRAS 12112+0305; 5σ, 15σ, 25σ, and 35σ for IRAS 20414−1651; and 3σ, 5σ, 10σ, and 15σ for IRAS 22491−1808. For IRAS 04103−2838 (Figure 1(c)) and IRAS 11095−0238 (Figure 1(f)), the white contours of the HCN J = 3–2 emission line (3σ, 5σ, and 7σ in the moment 0 map in Figure 3(e)) and HCO+ J = 3–2 emission line (4σ, 6σ, and 9σ in the moment 0 map in Figure 3(l)) are plotted together, respectively, to highlight the significant positional offset between the continuum and dense molecular line emission. IRAS 10485−1447 and IRAS 02411+0353 are not shown, as there was no significant (>3σ) continuum emission. The 1σ rms noise level in each source is tabulated in Table 3 (column 5). Beam sizes are shown as filled circles in the lower-left region.
Download figure:
Standard image High-resolution imageTable 3. Continuum Emission
Object | Frequency | Peak Flux | Peak Coordinate | rms | Synthesized Beam |
---|---|---|---|---|---|
(GHz) | (mJy/beam) | (R.A., Decl.) ICRS | (mJy/beam) | ('' × '') (°) | |
(1) | (2) | (3) | (4) | (5) | (6) |
IRAS 00188−0856 | 234.7–239.7 | 1.4 (35σ) | (00 21 26.514, −08 39 26.01) | 0.040 | 0.18 × 0.13 (−79°) |
IRAS 03250+1606 | 234.6–239.6 | 0.41 (14σ) | (03 27 49.812, +16 16 59.31) | 0.029 | 0.13 × 0.10 (−28°) |
IRAS 04103−2838 N | 236.9–241.8 | 0.21 (4.4σ) | (04 12 19.445, −28 30 25.00) | 0.047 | 0.11 × 0.10 (73°) |
IRAS 04103−2838 S | 236.9–241.8 | 0.18 (3.9σ) | (04 12 19.445, −28 30 25.14) | 0.047 | 0.11 × 0.10 (73°) |
IRAS 04103−2838 NE | 236.9–241.8 | 0.18 (3.9σ) | (04 12 19.440, −28 30 24.90) | 0.047 | 0.11 × 0.10 (73°) |
IRAS 09039+0503 SW | 235.4–240.3 | 0.83 (19σ) | (09 06 34.027, +04 51 27.43) | 0.044 | 0.18 × 0.14 (77°) |
IRAS 09039+0503 NE | 235.4–240.3 | 0.46 (10σ) | (09 06 34.045, +04 51 27.87) | 0.044 | 0.18 × 0.14 (77°) |
IRAS 10378+1108 | 233.0–238.0 | 1.5 (33σ) | (10 40 29.170, +10 53 18.15) | 0.045 | 0.17 × 0.15 (−81°) |
IRAS 10485−1447 | 233.7–238.6 | <0.14 (<3σ)a | (10 51 03.67, −15 03 19.8)a | 0.044 | 0.13 × 0.10 (−78°) |
IRAS 11095−0238 NE | 239.4–244.3 | 1.2 (18σ) | (11 12 03.383, −02 54 22.96) | 0.066 | 0.13 × 0.12 (−83°) |
IRAS 11095−0238 SW | 239.4–244.3 | 0.43 (6.5σ) | (11 12 03.357, −02 54 23.30) | 0.066 | 0.13 × 0.12 (−83°) |
IRAS 13335−2612 N | 235.4–240.3 | 1.2 (26σ) | (13 36 22.290, −26 27 32.78) | 0.048 | 0.16 × 0.12 (−87°) |
IRAS 13335−2612 S | 235.4–240.3 | 0.37 (7.8σ) | (13 36 22.297, −26 27 34.38) | 0.048 | 0.16 × 0.12 (−87°) |
IRAS 14348−1447 SW | 244.5–249.5 | 1.4 (21σ) | (14 37 38.281, −15 00 24.21) | 0.070 | 0.14 × 0.10 (−56°) |
IRAS 14348−1447 NE | 244.5–249.5 | 0.99 (14σ) | (14 37 38.397, −15 00 21.27) | 0.070 | 0.14 × 0.10 (−56°) |
IRAS 16090−0139 | 233.6–238.5 | 1.4 (20σ) | (16 11 40.422, −01 47 06.34) | 0.070 | 0.17 × 0.15 (−83°) |
IRAS 21329−2346 | 235.4–240.2 | 1.4 (21σ) | (21 35 45.861, −23 32 35.35) | 0.071 | 0.75 × 0.59 (87°) |
IRAS 23234+0946 | 234.7–239.7 | 0.45 (20σ) | (23 25 56.142, +10 02 49.61) | 0.023 | 0.15 × 0.13 (33°) |
IRAS 00091−0738 | 236.8–241.8 | 5.5 (59σ) | (00 11 43.273, −07 22 07.35) | 0.094 | 0.18 × 0.13 (−79°) |
IRAS 00456−2904 | 238.5–243.4 | 1.2 (25σ) | (00 48 06.774, −28 48 18.52) | 0.050 | 0.16 × 0.12 (−73°) |
IRAS 01004−2237 | 236.9–241.8 | 0.64 (14σ) | (01 02 49.992, −22 21 57.28) | 0.047 | 0.17 × 0.12 (−71°) |
IRAS 01166−0844 SE | 236.8–241.8 | 0.68 (17σ) | (01 19 07.856, −08 29 12.03) | 0.041 | 0.12 × 0.092 (−71°) |
IRAS 01298−0744 | 233.0–238.0 | 2.4 (58σ) | (01 32 21.413, −07 29 08.34) | 0.042 | 0.15 × 0.12 (−55°) |
IRAS 01569−2939 | 232.2–237.2 | 0.70 (17σ) | (01 59 13.792, −29 24 35.03) | 0.040 | 0.11 × 0.11 (−70°) |
IRAS 02411+0353 | 231.5–236.4 | <0.13 (<3σ) | (02 43 46.032, +04 06 37.93)b | 0.041 | 0.13 × 0.10 (−50°) |
IRAS 10190+1322 | 246.1–251.0 | 1.3 (16σ) | (10 21 42.753, +13 06 55.60) | 0.081 | 0.17 × 0.15 (70°) |
IRAS 11506+1331 | 234.9–239.8 | 0.58 (12σ) | (11 53 14.230, +13 14 27.70) | 0.049 | 0.20 × 0.14 (−63°) |
IRAS 13509+0442 | 233.1–238.0 | 0.56 (12σ) | (13 53 31.566, +04 28 04.83) | 0.045 | 0.22 × 0.16 (26°) |
IRAS 22206−2715 | 234.0–238.9 | 1.7 (24σ) | (22 23 28.900, −27 00 02.84) | 0.069 | 0.75 × 0.60 (88°) |
IRAS 12112+0305 NEc | 246.8–251.3 | 8.3 (46σ) | (12 13 46.057, +02 48 41.49) | 0.18 | 0.73 × 0.55 (66°) |
IRAS 12112+0305 SWc | 246.8–251.3 | 0.80 (4σ) | (12 13 45.944, +02 48 39.09) | 0.19 | 0.73 × 0.55 (66°) |
IRAS 20414−1651c | 243.9–248.6 | 5.2 (38σ) | (20 44 18.165, −16 40 16.84) | 0.14 | 0.76 × 0.66 (−89°) |
IRAS 22491−1808c | 246.1–250.7 | 4.7 (19σ) | (22 51 49.349, −17 52 24.16) | 0.25 | 0.92 × 0.59 (67°) |
Notes. Column (1): object name. Column (2): frequency range (in GHz) used for continuum extraction. Frequencies of obvious emission lines and absorption features are removed. Column (3): flux (in mJy beam−1) at the emission peak. Value at the highest flux pixel (002–0
04 pixel−1) is extracted. The detection significance relative to the rms noise is shown in parentheses. Possible systematic uncertainties, coming from the absolute flux calibration ambiguity in individual ALMA observation and choice of frequency range for the continuum level determination, are not included. Column (4): coordinate of the continuum emission peak in ICRS. Column (5): rms noise level (1σ) (in mJy beam−1), derived from the standard deviation of sky signals in each continuum map. Column (6): synthesized beam (in arcsec × arcsec) and position angle (in degrees). The position angle is 0° along the north–south direction and increases in the counterclockwise direction.

Download table as: ASCIITypeset image
Figure 2 (first and third columns) displays the continuum-subtracted full frequency coverage spectra at the continuum peak position within the synthesized beam size. Gaussian fits for the detected HCN J = 3–2 and HCO+ J = 3–2 emission lines were applied, after removing data points possibly affected by other emission and/or absorption features, and fitting results are listed in Tables 4 and 5, respectively. The best Gaussian fits are shown in the Appendix B. Figure 3 shows integrated intensity (moment 0) maps of the HCN J = 3–2 and HCO+ J = 3–2 emission lines by summing velocity channels with significant line signals. The peak flux values of the HCN J = 3–2 and HCO+ J = 3–2 emission lines in the moment 0 maps are summarized in Tables 4 and 5, respectively. These values roughly agree with the Gaussian fit estimates (columns 2 and 8 in Tables 4 and 5). For the HCN J = 3–2 and HCO+ J = 3–2 emission line fluxes, Gaussian fit estimates will be used in our discussion. For the HCO+ J = 3–2 emission line flux estimates, possible contamination from the nearby vibrationally excited (v2 = 1f) HCN J = 3–2 emission lines cannot be completely removed. However, their contributions should be negligible given much smaller fluxes than the HCO+ J = 3–2 emission line fluxes (see Section 5.2).
Download figure:
Standard image High-resolution imageDownload figure:
Standard image High-resolution imageFigure 2. ALMA full frequency coverage spectra of the observed ULIRGs. First and third columns: spectra at the continuum peak position within the beam size. For IRAS 04103–2838 (Figure 2(c)), the spectrum is extracted at the peak position of both the HCN J = 3 − 2 and HCO + J = 3 − 2 emission lines, which is significantly displaced from the continuum peak position (Figure 1(c)). Second and fourth columns: spectra within a 1'' diameter circular aperture around the continuum peak position. For IRAS 09039 + 0503 (Figure 2(d')) and IRAS 11095−0238 (Figure 2(h'), spatially integrated spectra with a 15 diameter circular aperture are shown, which include emission from multiple components. A 1
5 (IRAS 21329−2346 and IRAS 22206−2715 and IRAS 20414−1651) or 2'' (IRAS 12112+0305 northeast and southwest and IRAS 22491−1808) diameter circular aperture is used for sources with large synthesized beam sizes (0
5–0
9). Spatially integrated spectra are not shown for IRAS 10485−1447 and IRAS 02411−0353, because molecular emission lines are too faint to be detectable, due to increased noise in wider aperture spectra.
Download figure:
Standard image High-resolution imageDownload figure:
Standard image High-resolution imageDownload figure:
Standard image High-resolution imageFigure 3. Integrated intensity (moment 0) maps of the HCN J = 3–2 and HCO+ J = 3–2 emission lines of the observed ULIRGs with >3σ detection. The abscissa and ordinate are R.A. and decl. in ICRS, respectively. The contours are 5σ, 10σ, and 20σ for IRAS 00188−0856 HCN J = 3–2; 5σ, 10σ, and 15σ for IRAS 00188−0856 HCO+ J = 3–2; 4σ and 9σ for IRAS 03250+1606 HCN J = 3–2; 5σ and 8σ for IRAS 03250+1606 HCO+ J = 3–2; 3σ, 5σ, and 7σ for IRAS 04103−2838 HCN J = 3–2; 3σ and 6σ for IRAS 04103−2838 HCO+ J = 3–2; 4σ, 8σ, and 12σ for IRAS 09039+0503 HCN J = 3–2; 4σ, 6σ, and 8σ for IRAS 09039+0503 HCO+ J = 3–2; 6σ, 12σ, and 24σ for IRAS 10378+1108 HCN J = 3–2; 5σ, 10σ, and 20σ for IRAS 10378+1108 HCO+ J = 3–2; 4σ, 8σ, and 12σ for IRAS 11095−0238 HCN J = 3–2; 4σ, 6σ, and 9σ for IRAS 11095−0238 HCO+ J = 3–2; 4σ and 8σ for IRAS 13335−2612 N HCN J = 3–2; 4σ and 7σ for IRAS 13335−2612 N HCO+ J = 3–2; 3σ and 4σ for IRAS 13335−2612 S HCN J = 3–2; 3σ for IRAS 13335−2612 S HCO+ J = 3–2; 6σ, 12σ, and 24σ for IRAS 14348−1447 SW HCN J = 3–2; 5σ, 9σ, and 13σ for IRAS 14348−1447 SW HCO+ J = 3–2; 4σ, 8σ, and 12σ for IRAS 14348−1447 NE HCN J = 3–2; 4σ and 6σ for IRAS 14348−1447 NE HCO+ J = 3–2; 4σ, 8σ, 16σ, and 24σ for IRAS 16090−0139 HCN J = 3–2; 4σ, 8σ, and 16σ for IRAS 16090−0139 HCO+ J = 3–2; 4σ, 9σ, and 14σ for IRAS 21329−2346 HCN J = 3–2; 5σ, 10σ, and 15σ for IRAS 21329−2346 HCO+ J = 3–2; 5σ and 9σ for IRAS 23234+0946 HCN J = 3–2; 4σ and 9σ for IRAS 23234+0946 HCO+ J = 3–2; 4σ, 10σ, and 16σ for IRAS 00091−0738 HCN J = 3–2; 5σ, 9σ, and 13σ for IRAS 00091−0738 HCO+ J = 3–2; 4σ, 8σ, and 16σ for IRAS 00456−2904 HCN J = 3–2; 4σ, 8σ, and 12σ for IRAS 00456−2904 HCO+ J = 3–2; 4σ, 9σ, and 14σ for IRAS 01004−2237 HCN J = 3–2; 4σ, 9σ, and 14σ for IRAS 01004−2237 HCO+ J = 3–2; 5σ, 10σ, and 15σ for IRAS 01166−0844 SE HCN J = 3–2; 5σ, 7σ, and 9σ for IRAS 01166−0844 SE HCO+ J = 3–2; 5σ, 11σ, and 17σ for IRAS 01298−0744 HCN J = 3–2; 4σ, 8σ, and 12σ for IRAS 01298−0744 HCO+ J = 3–2; 5σ, 10σ, and 15σ for IRAS 01569−2939 HCN J = 3–2; 5σ, 9σ, and 13σ for IRAS 01569−2939 HCO+ J = 3–2; 2.5σ and 3.3σ for IRAS 02411+0353 HCN J = 3–2; 2.8σ and 3.2σ for IRAS 02411+0353 HCO+ J = 3–2; 4σ, 8σ, and 12σ for IRAS 10190+1322 HCN J = 3–2; 4σ, 7σ, and 10σ for IRAS 10190+1322 HCO+ J = 3–2; 4σ, 6σ, and 8σ for IRAS 11506+1331 HCN J = 3–2; 4σ, 7σ, and 10σ for IRAS 11506+1331 HCO+ J = 3–2; 3σ and 6σ for IRAS 13509+0442 HCN J = 3–2; 3σ, 5σ, and 7σ for IRAS 13509+0442 HCO+ J = 3–2; 5σ, 12σ, and 19σ for IRAS 22206−2715 HCN J = 3–2; 4σ, 10σ, and 16σ for IRAS 22206−2715 HCO+ J = 3–2; 3σ, 5σ, 10σ, and 20σ for IRAS 12112+0305 NE HCN J = 3–2; 3σ, 5σ, 10σ, and 15σ for IRAS 12112+0305 NE HCO+ J = 3–2; 3σ, and 4σ for IRAS 12112+0305 SW HCN J = 3–2; 4σ, 5σ, and 6σ for IRAS 12112+0305 SW HCO+ J = 3–2; 5σ, 10σ, and 20σ for IRAS 20414−1651 HCN J = 3–2; 4σ, 8σ, and 12σ for IRAS 20414−1651 HCO+ J = 3–2; 5σ, 10σ, and 20σ for IRAS 22491−1808 HCN J = 3–2; and 5σ and 10σ for IRAS 22491−1808 HCO+ J = 3–2. IRAS 10485−1447 is not shown because of no molecular line detection. The 1σ rms noise level in each source is tabulated in Tables 4 and 5 (column 3). Beam sizes are shown as filled circles in the lower-left region. No cutoff in the signal-to-noise ratio is applied.
Download figure:
Standard image High-resolution imageTable 4. Observed Properties of the HCN J = 3–2 Emission Line at the Nuclear Peak Position
Object | Integrated Intensity (Moment 0) Map | Gaussian Fit | |||||
---|---|---|---|---|---|---|---|
Peak | rms | Beam | Velocity | Peak | FWHM | Flux | |
(Jy beam−1 km s−1) | ('' × '') (°) | (km s−1) | (mJy) | (km s−1) | (Jy km s−1) | ||
(1) | (2) | (3) | (4) | (5) | (6) | (7) | (8) |
IRAS 00188−0856 | 1.6 (25σ) | 0.065 | 0.18 × 0.13 (−79°) | 38544 ± 6 | 5.5 ± 0.2 | 355 ± 13 | 1.9 ± 0.1 |
IRAS 03250+1606 | 0.56 (11σ) | 0.049 | 0.13 × 0.10 (−28°) | 38572 ± 26 | 1.3 ± 0.1 | 526 ± 60 | 0.63 ± 0.09 |
IRAS 04103−2838a | 0.44 (7.3σ) | 0.060 | 0.11 × 0.10 (73°) | 35339 ± 26 | 1.4 ± 0.2 | 407 ± 69 | 0.53 ± 0.12 |
IRAS 09039+0503 SW | 0.86 (13σ) | 0.067 | 0.19 × 0.14 (78°) | 37685 ± 16 | 2.4 ± 0.2 | 419 ± 41 | 0.96 ± 0.12 |
IRAS 09039+0503 NE | 0.67 (10σ) | 0.067 | 0.19 × 0.14 (78°) | 37528 ± 12 | 2.6 ± 0.3 | 236 ± 45 | 0.57 ± 0.12 |
IRAS 10378+1108 | 1.9 (26σ) | 0.074 | 0.17 × 0.15 (−83°) | 40946 ± 6 | 5.8 ± 0.2 | 411 ± 12 | 2.2 ± 0.1 |
IRAS 10485−1447 | <0.24 (<3σ)b | 0.078 | 0.13 × 0.10 (−78°) | ⋯ | ⋯ | ⋯ | ⋯ |
IRAS 11095−0238 NE | 1.7 (14σ) | 0.11 | 0.13 × 0.12 (−60°) | 31956 ± 10 | 4.1 ± 0.2 | 437 ± 29 | 1.7 ± 0.1 |
IRAS 11095−0238 SW | <0.35(<3σ) | 0.11 | 0.13 × 0.12 (−60°) | 31770 ± 40 | 0.9 ± 0.3 | 301 ± 206 | 0.27 ± 0.21 |
IRAS 13335−2612 N | 0.82 (8.7σ) | 0.094 | 0.16 × 0.13 (−86°) | 37298 ± 61, 37806 ± 46 | 1.4 ± 0.1, 1.1 ± 0.3 | 517 ± 148, 299 ± 99 | 0.96 ± 0.24 |
IRAS 13335−2612 S | 0.40 (4.6σ) | 0.087 | 0.16 × 0.13 (−86°) | 37725 ± 21 | 1.1 ± 0.2 | 301 ± 49 | 0.33 ± 0.07 |
IRAS 14348−1447 SW | 1.9 (26σ) | 0.072 | 0.14 × 0.11 (−60°) | 24847 ± 6 | 6.7 ± 0.2 | 317 ± 13 | 2.1 ± 0.1 |
IRAS 14348−1447 NE | 0.86 (12σ) | 0.069 | 0.14 × 0.11 (−60°) | 24534 ± 16, 24803 ± 11 | 1.8 ± 0.5, 3.1 ± 0.3 | 162 ± 64, 229 ± 24 | 0.98 ± 0.17 |
IRAS 16090−0139 | 2.7 (26σ) | 0.10 | 0.17 × 0.17 (−53°) | 40033 ± 8 | 6.3 ± 0.2 | 539 ± 17 | 3.5 ± 0.2 |
IRAS 21329−2346 | 1.5 (15σ) | 0.098 | 0.76 × 0.60 (87°) | 37396 ± 26, 37719 ± 31 | 3.6 ± 0.3, 2.8 ± 0.4 | 284 ± 61, 266 ± 61 | 1.7 ± 0.3 |
IRAS 23234+0946 | 0.39 (10σ) | 0.039 | 0.16 × 0.13 (33°) | 38146 ± 25, 38511 ± 18 | 0.79 ± 0.12, 1.0 ± 0.1 | 223 ± 61, 267 ± 47 | 0.43 ± 0.08 |
IRAS 00091−0738 | 2.8 (17σ) | 0.17 | 0.18 × 0.13 (−78°) | 35145 ± 10, 35643 ± 20 | 5.6 ± 0.4, 3.4 ± 0.4 | 262 ± 27, 444 ± 88 | 2.8 ± 0.4 |
IRAS 00456−2904 | 1.5 (20σ) | 0.076 | 0.16 × 0.12 (−75°) | 32944 ± 6 | 6.3 ± 0.2 | 284 ± 12 | 1.7 ± 0.1 |
IRAS 01004−2237 | 0.82 (15σ) | 0.055 | 0.17 × 0.12 (−70°) | 35366 ± 7 | 4.7 ± 0.5 | 201 ± 22 | 0.89 ± 0.13 |
IRAS 01166−0844 SE | 1.1 (16σ) | 0.067 | 0.12 × 0.093 (−60°) | 35126 ± 15 | 2.6 ± 0.2 | 476 ± 36 | 1.2 ± 0.1 |
IRAS 01298−0744 | 1.3 (19σ) | 0.072 | 0.15 × 0.12 (−55°) | 41028 ± 14 | 2.6 ± 0.1 | 621 ± 47 | 1.5 ± 0.1 |
IRAS 01569−2939 | 1.1 (16σ) | 0.069 | 0.11 × 0.11 (−69°) | 41865 ± 21, 42277 ± 17 | 2.0 ± 0.2, 2.3 ± 0.2 | 351 ± 49, 268 ± 43 | 1.2 ± 0.2 |
IRAS 02411+0353 | 0.22 (3.5σ) | 0.064 | 0.13 × 0.11 (−50°) | 42990 ± 179 | 0.39 ± 0.11 | 715 (fix) | 0.26 ± 0.08 |
IRAS 10190+1322 | 1.2 (12σ) | 0.096 | 0.18 × 0.15 (72°) | 22856 ± 10 | 3.3 ± 0.2 | 379 ± 25 | 1.2 ± 0.1 |
IRAS 11506+1331 | 0.43 (9.0σ) | 0.047 | 0.20 × 0.14 (−63°) | 38129 ± 14 | 2.0 ± 0.2 | 235 ± 34 | 0.44 ± 0.08 |
IRAS 13509+0442 | 0.30 (7.0σ) | 0.044 | 0.22 × 0.16 (24°) | 40978 ± 12 | 1.8 ± 0.2 | 203 ± 28 | 0.35 ± 0.06 |
IRAS 22206−2715 | 2.2 (20σ) | 0.11 | 0.75 × 0.60 (88°) | 39490 ± 6 | 6.3 ± 0.2 | 413 ± 14 | 2.4 ± 0.1 |
IRAS 12112+0305 NEc | 8.3 (28σ) | 0.30 | 0.73 × 0.54 (66°) | 21661 ± 18, 21958 ± 20 | 15.0 ± 1.4, 14.6 ± 1.2 | 283 ± 34, 273 ± 46 | 8.1 ± 1.2 |
IRAS 12112+0305 SWc | 0.72 (4.2σ) | 0.17 | 0.73 × 0.54 (66°) | 21970 ± 34 | 2.0 ± 0.5 | 323 ± 75 | 0.64 ± 0.22 |
IRAS 20414−1651c | 3.9 (26σ) | 0.15 | 0.76 × 0.66 (−88°) | 25829 ± 11, 26216 ± 12 | 7.4 ± 0.5, 8.2 ± 0.4 | 250 ± 24, 309 ± 28 | 4.3 ± 0.3 |
IRAS 22491−1808c | 7.3 (26σ) | 0.28 | 0.95 × 0.60 (65°) | 23309 ± 6 | 18.0 ± 0.5 | 458 ± 13 | 8.1 ± 0.3 |
Notes. Column (1): object name. Column (2): integrated intensity of the HCN J = 3–2 emission line (νrest = 265.886 GHz) (in Jy beam−1 km s−1) at the emission peak. Detection significance relative to the rms noise (1σ) in the moment 0 map is shown in parentheses. Possible systematic uncertainty is not included. Column (3): rms noise (1σ) level in the moment 0 map (in Jy beam−1 km s−1), derived from the standard deviation of sky signals in each moment 0 map. Column (4): beam size (in arcsec×arcsec) and position angle (in degrees). Position angle is 0° along the north–south direction and increases counterclockwise. Columns (5)–(8): Gaussian fit of the HCN J = 3–2 emission line in the spectrum at the continuum peak position, within the beam size. For faint emission lines, we fixed some parameters to the best fit or fiducial values. Column (5): optical local standard of rest (LSR) velocity (vopt) of emission line peak (in km s−1). Column (6): peak flux (in mJy). Column (7): observed full width at half maximum (FWHM) (in km s−1). Column (8): flux (in Jy km s−1). For the flux estimate, the observed FWHM (in km s−1) in column 7 is divided by (1 + z) to obtain the intrinsic FWHM (in km s−1).
aHCN J = 3–2 emission line peak at (04 12 19.448, −28 30 24.92)ICRS. bNo emission with >3σ is detected within 0
Download table as: ASCIITypeset image
Table 5. Observed Properties of the HCO+ J = 3–2 Emission Line at the Nuclear Peak Position
Object | Integrated Intensity (Moment 0) Map | Gaussian Fit | |||||
---|---|---|---|---|---|---|---|
Peak | rms | Beam | Velocity | Peak | FWHM | Flux | |
(Jy beam−1 km s−1) | ('' × '') (°) | (km s−1) | (mJy) | (km s−1) | (Jy km s−1) | ||
(1) | (2) | (3) | (4) | (5) | (6) | (7) | (8) |
IRAS 00188−0856 | 0.86 (15σ) | 0.056 | 0.18 × 0.13 (−79°) | 38538 ± 10 | 3.1 ± 0.2 | 345 ± 23 | 1.0 ± 0.1 |
IRAS 03250+1606 | 0.40 (8.5σ) | 0.047 | 0.13 × 0.10 (−28°) | 38558 ± 29 | 0.98 ± 0.11 | 535 ± 59 | 0.49 ± 0.08 |
IRAS 04103−2838a | 0.40 (6.4σ) | 0.062 | 0.11 × 0.10 (74°) | 35346 ± 18 | 1.6 ± 0.2 | 300 ± 48 | 0.46 ± 0.09 |
IRAS 09039+0503 SW | 0.46 (7.4σ) | 0.062 | 0.18 × 0.14 (80°) | 37721 ± 23 | 1.4 ± 0.2 | 413 ± 58 | 0.56 ± 0.10 |
IRAS 09039+0503 NE | 0.55 (8.9σ) | 0.062 | 0.18 × 0.14 (80°) | 37555 ± 12 | 2.1 ± 0.2 | 257 ± 32 | 0.51 ± 0.08 |
IRAS 10378+1108 | 1.8 (24σ) | 0.075 | 0.17 × 0.15 (−81°) | 40948 ± 7 | 5.3 ± 0.2 | 399 ± 17 | 2.0 ± 0.1 |
IRAS 10485−1447 | <0.23 (<3σ)b | 0.076 | 0.13 × 0.10 (−76°) | ⋯ | ⋯ | ⋯ | ⋯ |
IRAS 11095−0238 NE | 0.89 (10σ) | 0.087 | 0.13 × 0.12 (−63°) | 32017 ± 26 | 2.1 ± 0.2 | 467 ± 68 | 0.92 ± 0.16 |
IRAS 11095−0238 SW | 0.41 (4.8σ) | 0.087 | 0.13 × 0.12(−63°) | 31813 ± 49 | 1.0 ± 0.2 | 468 ± 124 | 0.47 ± 0.15 |
IRAS 13335−2612 N | 0.58 (7.4σ) | 0.079 | 0.16 × 0.13 (−87°) | 37229 ± 56, 37713 ± 30 | 0.87 ± 0.18, 1.1 ± 0.2 | 307 ± 64, 366 ± 70 | 0.62 ± 0.12 |
IRAS 13335−2612 S | 0.25 (3.1σ) | 0.079 | 0.16 × 0.13 (−87°) | 37718 ± 31 | 0.78 ± 0.19 | 235 ± 77 | 0.17 ± 0.07 |
IRAS 14348−1447 SW | 1.2 (14σ) | 0.091 | 0.15 × 0.12 (−55°) | 24863 ± 7 | 4.4 ± 0.2 | 318 ± 16 | 1.4 ± 0.1 |
IRAS 14348−1447 NE | 0.61 (7.2σ) | 0.085 | 0.14 × 0.11 (−57°) | 24550 ± 34, 24814 (fix) | 1.4 ± 0.5, 2.0 (fix) | 148 (fix), 231 (fix) | 0.65 ± 0.07 |
IRAS 16090−0139 | 2.1 (18σ) | 0.12 | 0.17 × 0.17 (−66°) | 40024 ± 16 | 4.6 ± 0.2 | 560 ± 37 | 2.7 ± 0.2 |
IRAS 21329−2346 | 1.6 (16σ) | 0.095 | 0.75 × 0.59 (87°) | 37388 ± 12, 37723 ± 13 | 3.8 ± 0.2, 3.3 ± 0.2 | 283 ± 29, 242 ± 30 | 1.8 ± 0.2 |
IRAS 23234+0946 | 0.37 (9.8σ) | 0.038 | 0.16 × 0.13 (33°) | 38408 ± 27 | 0.84 ± 0.08 | 545 ± 69 | 0.43 ± 0.07 |
IRAS 00091−0738 | 1.5 (15σ) | 0.10 | 0.19 × 0.13 (−80°) | 35151 ± 11, 35762 ± 17 | 3.1 ± 0.4, 3.4 ± 0.3 | 172 ± 21, 378 ± 36 | 1.7 ± 0.2 |
IRAS 00456−2904 | 0.76 (14σ) | 0.056 | 0.16 × 0.12 (−74°) | 32938 ± 7 | 3.4 ± 0.2 | 266 ± 16 | 0.87 ± 0.07 |
IRAS 01004−2237 | 0.82 (15σ) | 0.054 | 0.17 × 0.12 (−72°) | 35364 ± 6 | 5.0 ± 0.3 | 195 ± 12 | 0.93 ± 0.08 |
IRAS 01166−0844 SE | 0.71 (10σ) | 0.071 | 0.12 × 0.095 (−61°) | 35185 ± 25 | 1.8 ± 0.2 | 430 ± 60 | 0.75 ± 0.13 |
IRAS 01298−0744 | 1.1 (14σ) | 0.077 | 0.15 × 0.12 (−55°) | 41057 ± 21 | 2.0 ± 0.1 | 634 ± 57 | 1.2 ± 0.1 |
IRAS 01569−2939 | 1.1 (14σ) | 0.078 | 0.11 × 0.11 (−60°) | 41790 ± 34, 42279 ± 42 | 1.6 ± 0.2, 1.7 ± 0.1 | 369 ± 79, 471 ± 94 | 1.3 ± 0.2 |
IRAS 02411+0353 | 0.18 (3.4σ) | 0.052 | 0.13 × 0.10 (−50°) | 43162 ± 111 | 0.42 ± 0.29 | 633 (fix) | 0.25 ± 0.17 |
IRAS 10190+1322 | 1.0 (11σ) | 0.094 | 0.17 × 0.15 (69°) | 22845 ± 17 | 2.8 ± 0.2 | 396 ± 37 | 1.1 ± 0.1 |
IRAS 11506+1331 | 0.53 (10σ) | 0.052 | 0.20 × 0.14 (−64°) | 38128 ± 12 | 2.4 ± 0.2 | 258 ± 23 | 0.58 ± 0.07 |
IRAS 13509+0442 | 0.30 (7.5σ) | 0.041 | 0.22 × 0.16 (26°) | 40944 ± 17 | 1.5 ± 0.2 | 231 ± 42 | 0.33 ± 0.08 |
IRAS 22206−2715 | 1.5 (16σ) | 0.091 | 0.75 × 0.60 (87°) | 39500 ± 9 | 4.6 ± 0.2 | 398 ± 19 | 1.7 ± 0.1 |
IRAS 12112+0305 NEc | 4.5 (17σ) | 0.26 | 0.76 × 0.62 (68°) | 21665 ± 7, 21979 ± 6 | 11.1 ± 0.7, 12.4 ± 0.8 | 185 ± 18, 208 ± 20 | 4.6 ± 0.4 |
IRAS 12112+0305 SWc | 1.4 (6.6σ) | 0.21 | 0.76 × 0.62 (62°) | 21888 ± 17, 22071 ± 16 | 4.5 ± 0.5, 4.3 ± 0.8 | 186 ± 37, 125 ± 33 | 1.4 ± 0.3 |
IRAS 20414−1651c | 2.6 (13σ) | 0.21 | 0.76 × 0.67 (−88°) | 25834 ± 12, 26259 ± 12 | 5.8 ± 0.5, 6.3 ± 0.5 | 235 ± 31, 273 ± 32 | 3.0 ± 0.3 |
IRAS 22491−1808c | 3.5 (14σ) | 0.25 | 0.95 × 0.60 (66°) | 23255 ± 11 | 9.7 ± 0.5 | 371 ± 30 | 3.6 ± 0.3 |
Notes. Column (1): object name. Column (2): integrated intensity of the HCO+ J = 3–2 emission line (νrest = 267.558 GHz) (in Jy beam−1 km s−1) at the emission peak. Detection significance relative to the rms noise (1σ) in the moment 0 map is shown in parentheses. Possible systematic uncertainty is not included. Column (3): rms noise (1σ) level in the moment 0 map (in Jy beam−1 km s−1), derived from the standard deviation of sky signals in each moment 0 map. Column (4): Beam size (in arcsec×arcsec) and position angle (in degree). Position angle is 0° along the north–south direction and increases counterclockwise. Columns (5)–(8): Gaussian fit of the HCO+ J = 3–2 emission line in the spectrum at the continuum peak position, within the beam size. For faint emission lines, we fixed some parameters to the best fit or fiducial values. Column (5): optical LSR velocity (vopt) of emission line peak (in km s−1). Column (6): peak flux (in mJy). Column (7): observed FWHM (in km s−1). Column (8): flux (in Jy km s−1).
aHCO+ J = 3–2 emission line peak at (04 12 19.448, −28 30 24.92)ICRS. bNo emission with >3σ is detected within 0
Download table as: ASCIITypeset image
Intensity-weighted mean velocity (moment 1) and intensity-weighted velocity dispersion (moment 2) maps for HCN J = 3–2 and HCO+ J = 3–2 emission lines are shown in the Appendix C for selected ULIRGs with bright molecular lines.
Table 6 tabulates the estimated intrinsic emission sizes of the ALMA-detected continuum, HCN J = 3–2, and HCO+ J =3–2 lines after deconvolution, using the CASA task "imfit." These values will be used for the comparison of the spatial extents of molecular line and continuum emission, with respect to beam sizes. For most of the sources observed with small beam sizes (01–0
2 or 150–530 pc in physical scale), the deconvolved intrinsic emission sizes are significantly larger than the beam sizes, suggesting that the emission is spatially resolved. We extracted spectra within 1'' (1.4–2.5 kpc) diameter circular apertures around the continuum peak positions, which are displayed in Figure 2 (second and fourth columns). This aperture size was adopted, as ULIRG's continuum and dense molecular line emission are usually concentrated in compact nuclear (<a few kpc) regions (e.g., Wilson et al. 2008; Imanishi & Nakanishi 2014; Scoville et al. 2015; Tunnard et al. 2015; Imanishi et al. 2016c, 2018; Martin et al. 2016; Barcos-Munoz et al. 2017, 2018; Manohar & Scoville 2017; Sakamoto et al. 2017; Sliwa & Downes 2017). IRAS 09039+0503 (Figures 3(g) and (h)) and IRAS 11095−0238 (Figures 3(k) and (l)) show double nuclear molecular line emission with the separation of ∼0
5, for which we created spatially integrated spectra with 1
5 diameter circular apertures centered at the middle point of the two nuclei, to include emission from both nuclei (Figures 2(d') and (h')). In general, the molecular line peak fluxes are higher in these spatially integrated spectra than the peak-position beam-sized spectra (Figure 2, first and third columns), due to the contribution from spatially resolved (a few 100 pc to a few kpc) nuclear emission components.
Table 6. Emission Size after Deconvolution
Object | Continuum | HCN J = 3–2 | HCO+ J = 3–2 | Beam Size |
---|---|---|---|---|
(mas × mas (°)) | (mas × mas (°)) | (mas × mas (°)) | (mas × mas (°)) | |
(1) | (2) | (3) | (4) | (5) |
IRAS 00188−0856 | 248 ± 19 × 150 ± 11 (93 ± 6) | 332 ± 29 × 177 ± 16 (91 ± 5) | 352 ± 38 × 218 ± 24 (87 ± 10) | 180 × 130 (−79) |
IRAS 03250+1606 | 314 ± 40 × 284 ± 39 (4 ± 52) | 226 ± 32 × 186 ± 28 (136 ± 76) | 440 ± 86 × 254 ± 53 (2 ± 16) | 130 × 100 (−28) |
IRAS 04103−2838 | 380 ± 112 × 105 ± 54 (161 ± 7) | 165 ± 52 × 120 ± 50 (52 ± 40) | 284 ± 71 × 123 ± 38 (88 ± 11) | 110 × 100 (73) |
IRAS 09039+0503 SW | 254 ± 54 × 156 ± 53 (29 ± 29) | 401 ± 85 × 305 ± 71 (57 ± 45) | 705 ± 117 × 467 ± 83 (17 ± 18) | 180 × 140 (77) |
IRAS 09039+0503 NE | 587 ± 143 × 398 ± 104 (40 ± 29) | 387 ± 86 × 311 ± 77 (52 ± 41) | 541 ± 90 × 400 ± 71 (42 ± 55) | 180 × 140 (77) |
IRAS 10378+1108 | 137 ± 22 × 130 ± 25 (49 ± 84) | 220 ± 22 × 162 ± 22 (32 ± 17) | 263 ± 24 × 163 ± 21 (42 ± 9) | 170 × 150 (−81) |
IRAS 11095−0238 NW | 208 ± 43 × 159 ± 35 (2 ± 33) | 224 ± 39 × 165 ± 33 (12 ± 25) | 495 ± 86 × 205 ± 41 (52 ± 7) | 130 × 120 (−83) |
IRAS 13335−2612 N | 137 ± 17 × 44 ± 18 (89 ± 6) | 278 ± 56 × 178 ± 46 (58 ± 31) | 242 ± 60 × 224 ± 64(69 ± 83) | 160 × 120 (−87) |
IRAS 13335−2612 S | 292 ± 71 × 152 ± 40 (93 ± 18) | 247 ± 99 × 67 ± 61 (133 ± 29) | 269 ± 125 × 183 ± 124 (85 ± 52) | 160 × 120 (−87) |
IRAS 14348−1447 SW | 166 ± 26 × 148 ± 24 (119 ± 89) | 222 ± 32 × 204 ± 35 (10 ± 66) | 326 ± 45 × 273 ± 42 (35 ± 40) | 140 × 100 (−56) |
IRAS 14348−1447 NE | 259 ± 47 × 212 ± 45 (160 ± 49) | 335 ± 44 × 200 ± 29 (89 ± 12) | 357 ± 73 × 242 ± 56 (34 ± 24) | 140 × 100 (−56) |
IRAS 16090−0139 | 301 ± 39 × 260 ± 35 (170 ± 39) | 285 ± 20 × 203 ± 17 (268 ± 9) | 389 ± 35 × 264 ± 25 (30 ± 9) | 170 × 150 (−83) |
IRAS 21329−2346 | 429 ± 76 × 303 ± 127 (142 ± 34) | 436 ± 89 × 387 ± 135 (170 ± 66) | 568 ± 62 × 371 ± 112 (178 ± 61) | 750 × 590 (87) |
IRAS 23234+0946 | 303 ± 40 × 277 ± 37 (148 ± 88) | 413 ± 68 × 278 ± 47 (23 ± 22) | 427 ± 67 × 273 ± 44 (24 ± 16) | 150 × 130 (33) |
IRAS 00091−0738 | 96 ± 17 × 60 ± 15 (85 ± 22) | 205 ± 28 × 132 ± 22 (82 ± 18) | 170 ± 39 × 127 ± 37 (89 ± 89) | 180 × 130 (−79) |
IRAS 00456−2904 | 232 ± 34 × 189 ± 35 (38 ± 40) | 240 ± 25 × 188 ± 26 (28 ± 25) | 336 ± 44 × 240 ± 37 (56 ± 22) | 160 × 120 (−73) |
IRAS 01004−2237 | 163 ± 40 × 117 ± 38 (89 ± 72) | 158 ± 31 × 135 ± 28 (112 ± 82) | 149 ± 35 × 86 ± 58 (60 ± 28) | 170 × 120 (−71) |
IRAS 01166−0844 SE | 119 ± 28 × 82 ± 34 (51 ± 43) | 169 ± 32 × 101 ± 22 (107 ± 22) | 194 ± 49 × 152 ± 43 (109 ± 63) | 120 × 90 (−60) |
IRAS 01298−0744 | 82 ± 11 × 71 ± 10 (122 ± 69) | 132 ± 21 × 87 ± 17 (125 ± 30) | 200 ± 36 × 167 ± 36 (89 ± 54) | 150 × 120 (−55) |
IRAS 01569−2939 | 109 ± 20 × 61 ± 23 (119 ± 17) | 200 ± 28 × 169 ± 24 (148 ± 33) | 260 ± 35 × 212 ± 30 (19 ± 26) | 110 × 110 (70) |
IRAS 10190+1322 | 489 ± 47 × 267 ± 27 (42 ± 6) | 450 ± 44 × 309 ± 31 (53 ± 11) | 539 ± 50 × 264 ± 26 (47 ± 5) | 170 × 150 (70) |
IRAS 11506+1331 | 481 ± 49 × 263 ± 35 (46 ± 7) | 282 ± 62 × 197 ± 61 (65 ± 37) | 318 ± 66 × 250 ± 64 (10 ± 48) | 200 × 140 (−63) |
IRAS 13509+0442 | 475 ± 56 × 366 ± 46 (54 ± 30) | 480 ± 82 × 377 ± 71 (71 ± 31) | 496 ± 81 × 423 ± 76 (117 ± 53) | 220 × 160 (23) |
IRAS 22206−2715 | 310 ± 88 × 140 ± 128 (165 ± 70) | 401 ± 97 × 204 ± 148 (160 ± 61) | 457 ± 104 × 217 ± 143 (146 ± 25) | 750 × 600 (88) |
IRAS 12112+0305 NEa | 250 ± 40 × 230 ± 40 (85 ± 84) | 360 ± 30 × 140 ± 60 (155 ± 13) | 370 ± 90 × 150 ± 140 (128 ± 27) | 730 × 550 (66) |
IRAS 20414−1651a | 400 ± 40 × 320 ± 50 (168 ± 25) | 470 ± 60 × 300 ± 110 (178 ± 59) | 520 ± 120 × 410 ± 160 (105 ± 82) | 760 × 660 (−89) |
IRAS 22491−1808a | <430 × <110 (—) | 210 ± 100 × 150 ± 80 (10 ± 64) | could not deconvolve | 920 × 590 (67) |
Notes. Column (1): object name. Columns (2), (3), and (4): intrinsic emission size (in mas) along the major and minor axis, after deconvolution, using the CASA task "imfit," for the continuum, HCN J = 3–2, and HCO+ J = 3–2, respectively. The position angle (in degrees) is shown in parentheses. Column (5): synthesized beam size for the continuum data shown for comparison, because it is difficult to constrain the intrinsic emission size much smaller than the beam size. The size (in mas) along the major and minor axis, and the position angle (in degrees, in parentheses) are shown.
aTaken from Imanishi et al. (2016c).Download table as: ASCIITypeset image
For IRAS 21329−2346, IRAS 22206−2715, IRAS 12112+0305, IRAS 20414−1651, and IRAS 22491−1808 that were observed with larger beam sizes (05–0
9 or 0.9–1.7 kpc), we created spatially integrated spectra with 1
5 or 2'' diameter circular apertures (Figures 2(o'), (aa'), (ab'), (ac'), (ad'), and (ae')). For these five ULIRGs, the flux increase from the peak position within the beam size (Tables 4 and 5) to the integrated area (Table 7), is relatively modest (<20%–30%) compared to other ULIRGs observed with small 0
1–0
2 synthesized beam sizes, as expected, because the bulk of molecular line fluxes in these five ULIRGs have already been covered in the peak-position beam-sized spectra.
Table 7. Gaussian Fit Fluxes of HCN J = 3–2 and HCO+ J = 3–2 Emission Lines in Spatially Integrated Spectra
Object | Gaussian Fit (HCN J = 3–2) | Gaussian Fit (HCO+ J = 3–2) | ||||||
---|---|---|---|---|---|---|---|---|
Velocity | Peak | FWHM | Flux | Velocity | Peak | FWHM | Flux | |
(km s−1) | (mJy) | (km s−1) | (Jy km s−1) | (km s−1) | (mJy) | (km s−1) | (Jy km s−1) | |
(1) | (2) | (3) | (4) | (5) | (6) | (7) | (8) | (9) |
IRAS 00188−0856 | 38548 ± 6 | 19 ± 1 | 339 ± 14 | 6.1 ± 0.3 | 38514 ± 10 | 12 ± 1 | 319 ± 20 | 3.5 ± 0.3 |
IRAS 03250+1606 | 38620 ± 28 | 5.8 ± 0.6 | 540 ± 54 | 2.9 ± 0.4 | 38652 ± 26 | 6.8 ± 0.6 | 520 ± 58 | 3.3 ± 0.5 |
IRAS 04103−2838 | 35327 ± 86 | 4.9 ± 1.9 | 382 (fix) | 1.8 ± 0.7 | 35267 ± 57 | 7.0 ± 1.8 | 315 (fix) | 2.1 ± 0.5 |
IRAS 09039+0503 (1![]() |
37583 ± 12 | 19 ± 1 | 376 ± 29 | 6.8 ± 0.7 | 37569 ± 11 | 19 ± 1 | 384 ± 26 | 7.0 ± 1.2 |
IRAS 10378+1108 | 40911 ± 9 | 16 ± 1 | 384 ± 24 | 5.8 ± 0.5 | 40895 ± 9 | 16 ± 1 | 378 ± 23 | 5.7 ± 0.4 |
IRAS 11095−0238 (1![]() |
31949 ± 40 | 14 ± 2 | 458 ± 114 | 6.1 ± 1.9 | 31903 ± 31 | 14 ± 2 | 397 ± 86 | 5.5 ± 1.5 |
IRAS 13335−2612 N | 37486 ± 52 | 4.3 ± 0.6 | 785 ± 136 | 3.2 ± 0.7 | 37471 ± 64 | 3.3 ± 0.7 | 646 ± 122 | 2.0 ± 0.6 |
IRAS 13335−2612 S | 37718 ± 62 | 2.8 ± 1.0 | 338 (fix) | 0.89 ± 0.33 | 37681 ± 79 | 1.9 ± 0.5 | 460 (fix) | 0.81 ± 0.22 |
IRAS 14348−1447 SW | 24850 ± 10 | 24 ± 1 | 378 ± 27 | 8.8 ± 0.8 | 24842 ± 10 | 28 ± 2 | 302 ± 21 | 8.3 ± 0.8 |
IRAS 14348−1447 NE | 24691 ± 22 | 12 ± 1 | 415 ± 58 | 5.1 ± 0.9 | 24710 ± 31 | 10 ± 2 | 346 ± 68 | 3.4 ± 0.9 |
IRAS 16090−0139 | 40054 ± 13 | 21 ± 1 | 534 ± 31 | 11 ± 1 | 40090 ± 15 | 22 ± 1 | 576 ± 38 | 12 ± 1 |
IRAS 21329−2346 (1![]() |
37523 ± 13 | 4.1 ± 0.2 | 544 ± 30 | 2.1 ± 0.2 | 37548 ± 15 | 4.9 ± 0.3 | 523 ± 37 | 2.4 ± 0.2 |
IRAS 23234+0946 | 38389 ± 21 | 4.2 ± 0.3 | 585 ± 43 | 2.3 ± 0.2 | 38411 ± 23 | 4.7 ± 0.4 | 531 ± 43 | 2.3 ± 0.3 |
IRAS 00091−0738 | 35181 ± 15, 35604 ± 21 | 13 ± 1, 10 ± 1 | 283, 283 (fix) | 6.1 ± 0.4 | 35168 ± 29, 35727 ± 29 | 5.7 ± 0.9, 8.9 ± 0.9 | 283, 283 (fix) | 3.9 ± 0.4 |
IRAS 00456−2904 | 32931 ± 10 | 19 ± 1 | 299 ± 21 | 5.5 ± 0.5 | 32924 ± 12 | 16 ± 1 | 287 ± 60 | 4.4 ± 1.0 |
IRAS 01004−2237 | 35387 ± 32 | 7.8 ± 1.3 | 383 ± 83 | 2.8 ± 0.8 | 35359 ± 27 | 7.5 ± 1.0 | 381 (fix) | 2.7 ± 0.4 |
IRAS 01166−0844 SE | 35141 ± 40 | 8.9 ± 1.1 | 384 (fix) | 3.3 ± 0.4 | 35123 ± 34 | 7.9 ± 1.3 | 385 ± 72 | 2.9 ± 0.7 |
IRAS 01298−0744 | 40978 ± 50 | 5.3 ± 0.8 | 634 ± 117 | 3.1 ± 0.8 | 41031 ± 45 | 5.9 ± 0.8 | 662 ± 115 | 3.7 ± 0.8 |
IRAS 01569−2939 | 41994 ± 43 | 6.3 ± 0.8 | 643 ± 108 | 3.8 ± 0.8 | 42006 ± 32 | 8.5 ± 0.7 | 731 ± 79 | 5.8 ± 0.8 |
IRAS 10190+1322 | 22807 ± 15 | 18 ± 1 | 429 ± 32 | 7.7 ± 0.8 | 22835 ± 19 | 16 ± 1 | 444 ± 36 | 7.0 ± 0.8 |
IRAS 11506+1331 | 38104 ± 20 | 6.9 ± 1.3 | 245 ± 54 | 1.6 ± 0.5 | 38127 ± 15 | 9.8 ± 1.4 | 252 ± 35 | 2.3 ± 0.5 |
IRAS 13509+0442 | 40928 ± 15 | 7.1 ± 0.8 | 267 ± 31 | 1.8 ± 0.3 | 40907 ± 12 | 8.8 ± 0.8 | 248 ± 30 | 2.0 ± 0.3 |
IRAS 22206−2715 (1![]() |
39484 ± 6 | 7.4 ± 0.2 | 403 ± 15 | 2.8 ± 0.1 | 39503 ± 8 | 5.5 ± 0.2 | 386 ± 19 | 2.0 ± 0.1 |
IRAS 12112+0305 NE (2'') | 21833 ± 11 | 21 ± 1 | 520 ± 23 | 11 ± 1 | 21674 ± 9, 21956 ± 9 | 13 ± 2, 16 ± 1 | 159 ± 25, 201 ± 26 | 5.3 ± 0.7 |
IRAS 12112+0305 SW (2'') | 21958 ± 34 | 3.5 ± 1.3 | 190 (fix) | 0.67 ± 0.24 | 21958 ± 40 | 3.7 ± 0.9 | 280 (fix) | 1.0 ± 0.3 |
IRAS 20414−1651 (1![]() |
25823 ± 11, 26204 ± 11 | 8.2 ± 0.5, 9.4 ± 0.4 | 237 ± 24, 330 ± 24 | 5.0 ± 0.3 | 25835 ± 11, 26250 ± 12 | 7.1 ± 0.6, 7.4 ± 0.6 | 227 ± 25, 263 ± 31 | 3.5 ± 0.4 |
IRAS 22491−1808 (2'') | 23313 ± 9 | 19 ± 1 | 450 ± 19 | 8.4 ± 0.5 | 23264 ± 15 | 10 ± 1 | 314 ± 39 | 3.3 ± 0.5 |
Note. Column (1): object name. Columns (2)–(5): Gaussian fit of the HCN J = 3–2 emission line (νrest = 265.886 GHz) (in Jy km s−1) in the spectrum within a 1''–2'' diameter circular aperture around the continuum peak position. Column (2): optical LSR velocity (vopt) of the emission line peak (in km s−1). Column (3): peak flux (in mJy). Column (4): observed FWHM (in km s−1). Column (5): flux (in Jy km s−1). Columns (6)–(9): Gaussian fit of the HCO+ J = 3–2 emission line (νrest = 267.558 GHz) (in Jy km s−1) in the spectrum within a 1''–2'' diameter circular aperture around the continuum peak position. Column (6): optical LSR velocity (vopt) of the emission line peak (in km s−1). Column (7): peak flux (in mJy). Column (8): observed FWHM (in km s−1). Column (9): flux (in Jy km s−1).
Download table as: ASCIITypeset image
Figure 4 compares the intrinsic deconvolved size of continuum versus HCN J = 3–2 and HCO+ J = 3–2 emission lines (left) and HCN J = 3–2 versus HCO+ J = 3–2 (right). While the HCN J = 3–2 line emission size is comparable to the continuum emission size (Figure 4, left), the emission size of the HCO+ J = 3–2 line is larger overall than those of the continuum and HCN J = 3–2 line (Figure 4, left and right). The more spatially extended emission for HCO+ J = 3–2 than for HCN J = 3–2 is reasonable, given that the critical density of HCO+ J = 3–2 is a factor of ∼5 smaller than that of HCN J = 3–2 (Shirley 2015). Thus, HCO+ can be collisionally excited to J = 3 to a greater extent than HCN by spatially extended low-density and low-temperature molecular gas (compared to nuclear dense, warm molecular gas).
Figure 4. Comparison of the intrinsic emission size after deconvolution for the continuum, HCN J = 3–2, and HCO+ J = 3–2 for ULIRGs observed with small beam sizes (01–0
2). Both major and minor axis values, as well as their geometric means, are plotted. The thick solid line indicates the same size between the abscissa and ordinate. Left: the abscissa is the continuum emission size (in mas). The ordinate is the size of the HCN J = 3–2 (red squares) or HCO+ J = 3–2 (light blue circles) emission line (in mas). Right: the size of the HCN J = 3–2 (abscissa) and HCO+ J = 3–2 (ordinate) emission lines (in mas).
Download figure:
Standard image High-resolution image5. Discussion
5.1. HCN-to-HCO+ J = 3–2 Flux Ratios
Table 8 tabulates the HCN-to-HCO+ J = 3–2 flux ratios based on Gaussian fit measurements in the nuclear beam-sized spectra and spatially integrated spectra for the 24 ULIRGs with significant dense molecular line detection in both spectra, excluding IRAS 10485−1447 and IRAS 02411−0353. These measurements are compared in Figure 5. The ratios in the beam-sized spectra at the nuclear peak position tend to be higher than those in the spatially integrated spectra, because most objects are located below the thick solid line in Figure 5. This suggests that the HCN-to-HCO+ J = 3–2 flux ratios are generally higher at the compact nuclear cores than in spatially resolved regions.
Figure 5. Comparison of the HCN-to-HCO+ J = 3–2 flux ratio at the nuclear continuum peak position within the beam size (abscissa) and within a 1''–2'' diameter circular aperture (ordinate). The thick solid line indicates the same ratio between the abscissa and ordinate. For ULIRGs observed in ALMA Cycle 5 with 01–0
2 synthesized beam sizes, 1'' diameter circular apertures are employed for the ordinate. These ULIRGs are plotted as filled circles. For ULIRGs observed with larger synthesized beam size (0
5–0
9), 1
5 (IRAS 21329−2346, IRAS 22206−2715, and IRAS 20414−1651) or 2'' (IRAS 12112+0305 northeast and southwest, and IRAS 22491−1808) diameter circular apertures are used, depending on the growth curve of HCN J = 3–2 and HCO+ J = 3–2 molecular line fluxes. These ULIRGs are plotted as filled gray triangles, where IRAS 22206−2715 and IRAS 20414−1651 are almost at the same location. IRAS 09039+0503 and IRAS 11095−0238 display double nuclear molecular emission with ∼0
5 separation (Figures 3(g), (h), (k), and (l)), for which measurements of molecular line fluxes with ≳1'' diameter circular apertures suffer from contamination from the other nucleus in the abscissa. These two ULIRGs are not plotted.
Download figure:
Standard image High-resolution imageTable 8. HCN-to-HCO+ Flux Ratio at J = 3–2
Object | Peak (Beam Size) | Spatially Integrated |
---|---|---|
(1) | (2) | (3) |
IRAS 00188−0856 | 1.9 ± 0.2 | 1.7 ± 0.2 |
IRAS 03250+1606 | 1.3 ± 0.3 | 0.9 ± 0.2 |
IRAS 04103−2838 | 1.2 ± 0.3 | 0.8 ± 0.4 |
IRAS 09039+0503 SW | 1.7 ± 0.4 | 1.0 ± 0.2a |
IRAS 09039+0503 NE | 1.1 ± 0.3 | 1.0 ± 0.2a |
IRAS 10378+1108 | 1.1 ± 0.1 | 1.0 ± 0.1 |
IRAS 11095−0238 NE | 1.9 ± 0.4 | 1.1 ± 0.5a |
IRAS 11095−0238 SW | 0.6 ± 0.5 | 1.1 ± 0.5a |
IRAS 13335−2612 N | 1.5 ± 0.5 | 1.6 ± 0.6 |
IRAS 13335−2612 S | 1.9 ± 0.8 | 1.1 ± 0.5 |
IRAS 14348−1447 SW | 1.5 ± 0.1 | 1.1 ± 0.1 |
IRAS 14348−1447 NE | 1.5 ± 0.3 | 1.5 ± 0.5 |
IRAS 16090−0139 | 1.3 ± 0.1 | 0.9 ± 0.1 |
IRAS 21329−2346 | 0.9 ± 0.2 | 0.9 ± 0.1b |
IRAS 23234+0946 | 1.0 ± 0.2 | 1.0 ± 0.2 |
IRAS 00091−0738 | 1.6 ± 0.3 | 1.6 ± 0.2 |
IRAS 00456−2904 | 2.0 ± 0.2 | 1.2 ± 0.3 |
IRAS 01004−2237 | 1.0 ± 0.2 | 1.0 ± 0.3 |
IRAS 01166−0844 SE | 1.6 ± 0.3 | 1.1 ± 0.3 |
IRAS 01298−0744 | 1.3 ± 0.2 | 0.9 ± 0.3 |
IRAS 01569−2939 | 1.0 ± 0.2 | 0.7 ± 0.2 |
IRAS 10190+1322 | 1.1 ± 0.2 | 1.1 ± 0.2 |
IRAS 11506+1331 | 0.8 ± 0.2 | 0.7 ± 0.2 |
IRAS 13509+0442 | 1.0 ± 0.3 | 0.9 ± 0.2 |
IRAS 22206−2715 | 1.4 ± 0.1 | 1.4 ± 0.1b |
IRAS 12112+0305 NE | 1.8 ± 0.3c | 2.0 ± 0.3d |
IRAS 12112+0305 SW | 0.5 ± 0.2c | 0.7 ± 0.3d |
IRAS 20414−1651 | 1.4 ± 0.2c | 1.4 ± 0.2b |
IRAS 22491−1808 | 2.3 ± 0.2c | 2.6 ± 0.4d |
Notes. Column (1): object name. Column (2): ratio of HCN J = 3–2 to HCO+ J = 3–2 flux (in Jy km s−1) at the nuclear peak position within the beam size. Column (3): ratio of HCN J = 3–2 to HCO+ J = 3–2 flux (in Jy km s−1) within a 1'' diameter circular aperture (unless otherwise noted).
aA 1

Download table as: ASCIITypeset image
To better investigate the enhanced HCN J = 3–2 emission at the compact nuclear cores, we created maps of the ratios of the HCN J = 3–2 to HCO+ J = 3–2 flux (in Jy km s−1) for ULIRGs observed with small (01–0
2) beam sizes (Figure 6). ULIRGs observed with larger beam sizes (0
5–0
9) are not plotted, as we were unable to obtain meaningful information regarding the spatial variation in the HCN-to-HCO+ J = 3–2 flux ratios. If an AGN produces stronger HCN emission than star formation (Section 1), the HCN-to-HCO+ J = 3–2 flux ratios are expected to be higher at the compact nuclear cores where AGNs reside than in spatially resolved off-nuclear regions where star formation is usually energetically dominant. This trend is clearly seen in the nearby well-studied optically identified luminous AGN, NGC 7469 (z = 0.016), in which the HCN-to-HCO+ J = 3–2 flux ratio is significantly higher at the AGN-dominated nuclear core than off-nuclear bright star-forming knots (Figure 6(a)). In Figure 6, HCN-to-HCO+ J = 3–2 flux ratios in nearby ULIRGs are also generally higher in the compact nuclear cores than the spatially resolved regions. AGN effects are a plausible scenario. In fact, an enhanced HCN abundance, relative to HCO+, in molecular gas in the close vicinity of luminous AGNs is observationally suggested (Imanishi et al. 2018; Nakajima et al. 2018; Saito et al. 2018), which can explain the elevated HCN emission of compact nuclear cores.3
Alternatively, the volume number density and temperature of molecular gas are expected to be very high in the nuclei of ULIRGs (Scoville et al. 2015). More HCN collisional excitation by nuclear dense and warm molecular gas than in the spatially resolved regions (Imanishi et al. 2018) can explain the observed HCN J = 3–2 flux enhancement in ULIRG's nuclei as well. ALMA multiple J-transition HCN and/or HCO+ observations (e.g., J = 2–1 and J = 4–3, in addition to J = 3–2) with similar beam sizes (0
1–0
2) are needed to constrain the spatial variation in molecular gas density and temperature, and thereby excitation conditions, in the observed ULIRGs. It is also possible that mechanical heating by shocks caused by spatially compact nuclear outflows (Aalto et al. 2012, 2017; Privon et al. 2017; Barcos-Munoz et al. 2018) contributes much for the observed nuclear molecular line flux ratios. An AGN is a plausible origin for such a compact outflow (Aalto et al. 2012, 2017), but a very compact starburst remains an alternative origin.
Download figure:
Standard image High-resolution imageFigure 6. Ratios of the HCN J = 3–2 to HCO+ J = 3–2 flux (in Jy km s−1) are shown as the images for ULIRGs observed with small (01–0
2) beam sizes. Continuum emission is overplotted as the black contours to indicate the nuclear location. The flux ratio map for the nearby well-studied optically identified luminous AGN, NGC 7469, is provided as a reference (Figure 6(a)), as it clearly shows an elevated HCN-to-HCO+ J = 3–2 flux ratio at the AGN-dominated nucleus, compared to circumnuclear starburst-dominated regions (Imanishi et al. 2016c). The continuum contours are 5σ, 10σ, 20σ, and 30σ (Imanishi et al. 2016c). For ULIRGs, the contour levels are the same as those in Figure 1, except IRAS 00188−0856 (5σ, 10σ, 20σ, and 30σ), IRAS 10378+1108 (3σ, 10σ, 20σ, and 30σ), IRAS 13335−2612 N (6σ, 12σ, and 24σ), IRAS 14348−1447 SW (5σ, 10σ, 15σ, and 20σ), IRAS 14348−1447 NE (5σ, 9σ, and 13σ), IRAS 23234+0946 (6σ, 12σ, and 18σ), and IRAS 11506+1331 (5σ, 8σ, and 11σ). IRAS 10485−1447 and IRAS 02411+0353 are not shown because molecular emission lines are too faint to create meaningful flux ratio maps. Only pixels whose HCO+ J = 3–2 emission line fluxes (i.e., denominator) are above a certain threshold are displayed to prevent the resulting ratio map from being dominated by noise.
Download figure:
Standard image High-resolution imageSeveral ULIRGs show higher HCN-to-HCO+ J = 3–2 flux ratios in off-nuclear local regions than the nuclear cores. Examples include IRAS 23234+0946 and IRAS 01004−2237, in which excess in the HCN-to-HCO+ J = 3–2 flux ratios are found both at the southeastern and northwestern sides of nuclei in Figures 6(l) and (o). Such off-nuclear local regions with enhanced HCN-to-HCO+ J = 3–2 flux ratios are also seen in the western part of the nuclei for IRAS 00188−0856 and IRAS 01298−0744 (Figures 6(b) and (q)). Although molecular line emission is generally fainter in off-nuclear edge regions than in nuclei, in Figure 6 we display only regions with ≳3σ HCO+ J = 3–2 emission line detection in the moment 0 maps in Figure 3. Mechanical heating by spatially extended outflow-related shocks may be responsible for these high HCN-to-HCO+ J = 3–2 flux ratios at off-nuclear regions (Izumi et al. 2013). It is also possible that molecular gas becomes locally dense and warm in off-nuclear regions through merger-induced processes.
Figure 7 is a plot of the nuclear HCN-to-HCO+ J = 3–2 flux ratios as a function of the infrared spectroscopically estimated AGN bolometric contribution (Nardini et al. 2010). ULIRGs with significant infrared-estimated AGN contributions tend to show HCN-to-HCO+ J = 3–2 flux ratios larger than unity, as expected for AGN-important galaxies (Krips et al. 2008; Imanishi et al. 2016c). Not only the infrared-identified AGN-significant ULIRGs but also the majority of ULIRGs with no significant infrared-detected AGNs show HCN J = 3–2 emission stronger than HCO+ J = 3–2 emission. The HCN-to-HCO+ J = 3–2 flux ratios in known starburst-dominated regions (mostly less infrared luminous than ULIRGs) are usually less than unity (Krips et al. 2008; Imanishi et al. 2016c). Plausible scenarios include (a) the presence of infrared-elusive but (sub)millimeter-detectable extremely deeply buried (τ > 1 at infrared 2.5–35 μm) luminous AGNs and their effects to nuclear molecular line fluxes (including both AGN radiative effects and mechanical heating by AGN-related compact outflow shocks) and/or (b) more HCN excitation to J = 3 than in less infrared luminous starburst galaxies due to dense and warm molecular gas in ULIRG's nuclei.
Figure 7. Comparison of infrared spectroscopically estimated AGN bolometric contribution (in %) by Nardini et al. (2010) (abscissa) and HCN-to-HCO+ J = 3–2 flux ratios at the nuclear continuum peak position within the beam size (ordinate) in the observed ULIRGs. ULIRGs observed with small (01–0
2) beam sizes are plotted as red circles. Those observed with large (0
5–0
9) beam sizes are plotted as light blue triangles. For several small-separation (<4'') double-nuclei ULIRGs, while the bolometric contributions of the AGNs based on Spitzer IRS infrared spectra are for both nuclei combined (Nardini et al. 2010), the derived HCN-to-HCO+ flux ratios at J = 3–2 shown in Table 8 (column 2) are for the individual nuclei separately. For IRAS 09039+0503, IRAS 11095−0238, IRAS 13335−2612, IRAS 14348−1447, and IRAS 12112+0305, we plot the HCN-to-HCO+ J = 3–2 flux ratios for the southwest, northeast, north, southwest, and northeast nuclei, respectively, because they are brighter than the other nuclei in the continuum (Figure 1) and thus are judged to dominate the bolometric luminosities.
Download figure:
Standard image High-resolution image5.2. Vibrationally Excited HCN J = 3–2 Emission Lines
Vibrationally excited (v2 = 1f) HCN J = 3–2 emission lines at νrest = 267.199 GHz were detected in several buried-AGN-hosting infrared luminous galaxies and such candidates (Sakamoto et al. 2010; Aalto et al. 2015a, 2015b; Imanishi et al. 2016b; Martin et al. 2016). This emission is believed to be vibrationally excited by infrared radiative pumping by absorbing ∼14 μm infrared photons rather than collisionally excited (Sakamoto et al. 2010; Aalto et al. 2015a). Because an AGN can emit ∼14 μm infrared photons more efficiently than star formation for given bolometric luminosity, due to AGN-heated hot dust thermal radiation, detection of the strong HCN v2 = 1f J = 3–2 emission line can be used to argue for the presence of a luminous AGN, particularly if the HCN v2 = 1f J = 3–2 to HCN J = 3–2 (v = 0) and/or infrared luminosity ratio are significantly larger than those found in pure star-forming regions (Aalto et al. 2015a; Imanishi et al. 2016c, 2017). We thus searched for signatures of the HCN v2 = 1f J = 3–2 emission line.
The detection of the HCN v2 = 1f J = 3–2 emission line is straightforward if the molecular line widths are small—say, <200 km s−1 FWHM—because we can clearly separate the contribution from the nearby strong HCO+ J = 3–2 (v = 0) emission line at νrest = 267.558 GHz (∼400 km s−1 separation; Sakamoto et al. 2010; Aalto et al. 2015a; Imanishi et al. 2016b; Martin et al. 2016). However, the detection is not easy for the majority of ULIRGs whose molecular line widths are usually large. The nuclear beam-sized spectra of IRAS 10378+1108, IRAS 11095−0238 NE, IRAS 14348−1447 SW, and IRAS 16090−0139 show possible signatures of the excess emission on the lower-frequency side of the HCO+ J = 3–2 line emission (Figures 2(f), (h), (l), and (n)), which may be attributable to the contribution from the HCN v2 = 1f J = 3–2 emission line. We compared the emission line profiles of HCN J = 3–2 and HCO+ J = 3–2 in detail, after normalizing peak flux values, and looked for sources that showed significant flux excess at the lower-frequency (high-velocity) side of the HCO+ J = 3–2 line, compared to the HCN J = 3–2 line. IRAS 10378+1108 and IRAS 11095−0238 NE may correspond to such cases (Figure 8). If the excess is due to the vibrationally excited HCN v2 = 1f J = 3–2 emission line, then its estimated fluxes are roughly ∼0.1 (Jy km s−1) for both sources. The HCN v2 = 1f to v = 0 flux ratios at J = 3–2 are ∼0.05, which is as high as an AGN-hosting ULIRG with a clearly detected HCN v2 = 1f J = 3–2 emission line (i.e., IRAS 20551−4250; Imanishi et al. 2016b, 2017), but is smaller than other such ULIRGs (Aalto et al. 2015a; Martin et al. 2016).
Figure 8. Comparison of the emission line profile of HCN J = 3–2 and HCO+ J = 3–2 in the nuclear beam-sized spectra of IRAS 10378+1108 and IRAS 11095−0238 NE, both of which show possible flux excess attributed to the vibrationally excited (v2 = 1f) HCN J = 3–2 emission line. The abscissa is the velocity in (km s−1) relative to the systemic value. The ordinate is the normalized flux. The expected velocity of the HCN v2 = 1f J = 3–2 line is indicated as a downward arrow.
Download figure:
Standard image High-resolution imageThe detection rate of the HCN v2 = 1f J = 3–2 emission line in ULIRGs observed in ALMA Cycle 5 is at most ∼9% (=2/23). Because IRAS 12112+0305 NE and IRAS 22491−1808, and possibly IRAS 20414−1651, observed in ALMA Cycle 2 or 3, display some signs of the HCN v2 = 1f J = 3–2 emission lines (Imanishi et al. 2016c), the detection rate in the complete sample could be as high as ∼19% (=5/26), which is still low. However, wide molecular emission lines in ULIRGs preclude the identification of the faint HCN v2 = 1f J = 3–2 emission line inside the emission tail of the bright HCO+ J = 3–2 (v = 0) line. The limited signal-to-noise ratios can also hamper the recognition of the HCN v2 = 1f J = 3–2 emission line. The actual fraction of ULIRGs showing the HCN v2 = 1f J = 3–2 emission lines could be higher. It is also possible that emission from the vicinity of a buried AGN is opaque even to the (sub)millimeter continuum (Aalto et al. 2015a), in which case the equivalent width of the HCN v2 = 1f J = 3–2 emission line could be low. If this were the case, it would mean that luminous buried AGNs can be elusive even through (sub)millimeter observations.
The signatures of the HCO+ v2 = 1f J = 3–2 (νrest = 268.689 GHz) emission lines were searched for in the obtained ALMA spectra, but were not detected clearly in any of the observed ULIRGs.
5.3. Molecular Inflow and Outflow
IRAS 00091−0738 (Figure 2(q)) shows double-peaked emission line profiles for HCN J = 3–2 and HCO+ J = 3–2. For HCO+ J = 3–2, because the flux at the central dip is below the continuum level, the double-peaked profile of the HCO+ J = 3–2 emission line cannot be solely due to rotation (Scoville et al. 2017) or self-absorption (Aalto et al. 2015a). Foreground absorption in front of the molecular line-emitting region is required at least for HCO+ J = 3–2. We created an integrated intensity (moment 0) map of this HCO+ J = 3–2 absorption component only (below the continuum level) and confirmed that the peak position of this absorption component spatially agrees with the continuum emission peak position in Table 3, supporting the foreground absorption scenario. IRAS 01004−2237 (Figure 2(s)) also displays an absorption feature at the lower-frequency side of the HCO+ J = 3–2 emission line. This absorption peak position is also spatially coincident with the continuum emission peak position in Table 3 within 1 pixel (002). These absorption features may be related to inflowing and/or outflowing molecular gas.
Molecular outflow signatures in nearby ULIRGs are usually seen as broad emission wings for bright molecular lines, mostly in CO J-transition lines (e.g., Maiolino et al. 2012; Cicone et al. 2014; Garcia-Burillo et al. 2015; Imanishi et al. 2017; Veilleux et al. 2017; Pereira-Santaella et al. 2018). The detection of the broad emission line wings in the HCN and HCO+ lines in nearby ULIRGs has been limited (Aalto et al. 2015b; Privon et al. 2017; Barcos-Munoz et al. 2018), simply due to the fainter emission line fluxes of these dense gas tracers compared to the brightest CO lines. The broad emission wings in HCN J = 3–2 and HCO+ J = 3–2 lines were not clearly seen in any of the observed ULIRGs in this paper (Figure 2).
5.4. Nuclear Dense Molecular Mass
The luminosities of the HCN J = 3–2 and HCO+ J = 3–2 emission lines are summarized in Table 9; Equations (1) and (3) of Solomon & Vanden Bout (2005) were used for the calculations from the estimated fluxes in spatially integrated (1''–2'') spectra (Table 7). These luminosities can be converted into the mass of dense (>105 cm−3) molecular gas within the nuclear (a few kpc) regions of the observed ULIRGs. When molecular gas emission lines are optically thick and thermally excited, i.e., where the excitation temperature Tex equals the gas kinetic temperature Tkin (Tex = Tkin), the molecular line luminosities in units of [K km s−1 pc2] should be comparable with respect to different J-transition lines. The conversion factor from molecular line luminosity to dense molecular gas mass is estimated to be αHCN = 6–40 for HCN J = 1–0 in various starbursts and AGNs (Mdense = 6–40× HCN J = 1–0 luminosity [M⊙ (K km s−1 pc2)−1]) (Gao & Solomon 2004a; Krips et al. 2008; Greve et al. 2009; Leroy et al. 2015). Although the αHCN value has some uncertainty, assuming the lower range of αHCN = 10, the derived dense molecular gas mass from the HCN J = 3–2 emission line luminosity is as high as >109 M⊙ for the observed nearby ULIRGs, except IRAS 10485−1447, IRAS 02411+0353, and IRAS 12112+0305 SW. Because the conversion factor (α) is estimated to be comparable for HCN J = 1–0 and HCO+ J = 1–0 (Leroy et al. 2015, 2017), we obtain similarly a high dense molecular gas mass of >109 M⊙ from the HCO+ J = 3–2 emission line luminosity. When HCN and HCO+ are only subthermally excited at J = 3–2 (Tex < Tkin), the luminosity in units of [K km s−1 pc2] is expected to be higher at J = 1–0 than at J = 3–2, so that the dense molecular gas mass will be even higher. The observed nearby ULIRGs are dense molecular gas rich in the nuclear few kpc regions.
Table 9. Luminosity of the HCN and HCO+ J = 3–2 Emission Lines
Object | HCN J = 3–2 | HCO+ J = 3–2 | HCN J = 3–2 | HCO+ J = 3–2 |
---|---|---|---|---|
105 (L⊙) | 105 (L⊙) | 107 (K km s−1 pc2) | 107 (K km s−1 pc2) | |
(1) | (2) | (3) | (4) | (5) |
IRAS 00188−0856 | 5.3 ± 0.3 | 3.0 ± 0.3 | 88 ± 5 | 50 ± 4 |
IRAS 03250+1606 | 2.6 ± 0.4 | 2.9 ± 0.4 | 42 ± 6 | 48 ± 7 |
IRAS 04103−2838 | 1.3 ± 0.5 | 1.5 ± 0.4 | 22 ± 8 | 25 ± 6 |
IRAS 09039+0503 (1![]() |
5.6 ± 0.6 | 5.8 ± 1.0 | 93 ± 9 | 94 ± 16 |
IRAS 10378+1108 | 5.7 ± 0.5 | 5.7 ± 0.4 | 95 ± 8 | 93 ± 7 |
IRAS 10485−1447 | <0.23a | <0.22a | <3.8a | <3.6a |
IRAS 11095−0238 (1![]() |
3.6 ± 1.1 | 3.2 ± 0.9 | 60 ± 18 | 53 ± 14 |
IRAS 13335−2612 N | 2.6 ± 0.6 | 1.7 ± 0.5 | 44 ± 9 | 27 ± 7 |
IRAS 13335−2612 S | 0.73 ± 0.27 | 0.67 ± 0.18 | 12 ± 5 | 11 ± 3 |
IRAS 14348−1447 SW | 3.1 ± 0.3 | 3.0 ± 0.3 | 52 ± 5 | 49 ± 5 |
IRAS 14348−1447 NE | 1.8 ± 0.3 | 1.2 ± 0.3 | 30 ± 5 | 20 ± 5 |
IRAS 16090−0139 | 9.9 ± 0.7 | 11 ± 1 | 165 ± 12 | 182 ± 15 |
IRAS 21329−2346 (1![]() |
1.7 ± 0.1 | 2.0 ± 0.2 | 29 ± 2 | 32 ± 3 |
IRAS 23234+0946 | 2.0 ± 0.2 | 2.0 ± 0.2 | 33 ± 3 | 33 ± 4 |
IRAS 00091−0738 | 4.5 ± 0.3 | 2.9 ± 0.3 | 75 ± 5 | 47 ± 4 |
IRAS 00456−2904 | 3.5 ± 0.3 | 2.8 ± 0.6 | 58 ± 5 | 46 ± 10 |
IRAS 01004−2237 | 2.1 ± 0.6 | 2.0 ± 0.3 | 34 ± 9 | 32 ± 4 |
IRAS 01166−0844 SE | 2.3 ± 0.3 | 2.1 ± 0.5 | 39 ± 5 | 34 ± 9 |
IRAS 01298−0744 | 3.1 ± 0.8 | 3.7 ± 0.8 | 52 ± 12 | 60 ± 13 |
IRAS 01569−2939 | 3.9 ± 0.8 | 6.0 ± 0.8 | 65 ± 14 | 98 ± 14 |
IRAS 02411+0353 | 0.24 ± 0.07a | 0.20 ± 0.06a | 4.0 ± 1.1a | 3.3 ± 1.0a |
IRAS 10190+1322 | 2.3 ± 0.2 | 2.1 ± 0.3 | 38 ± 4 | 34 ± 4 |
IRAS 11506+1331 | 1.4 ± 0.4 | 2.0 ± 0.4 | 23 ± 7 | 32 ± 6 |
IRAS 13509+0442 | 1.7 ± 0.3 | 2.0 ± 0.3 | 29 ± 5 | 33 ± 5 |
IRAS 22206−2715 (1![]() |
2.6 ± 0.1 | 1.8 ± 0.1 | 43 ± 2 | 30 ± 2 |
IRAS 12112+0305 NE (2'') | 2.9 ± 0.2 | 1.5 ± 0.2 | 48 ± 3 | 24 ± 3 |
IRAS 12112+0305 SW (2'') | 0.18 ± 0.07 | 0.28 ± 0.07 | 3.0 ± 1.1 | 4.6 ± 1.2 |
IRAS 20414−1651 (1![]() |
1.9 ± 0.1 | 1.4 ± 0.1 | 32 ± 2 | 22 ± 2 |
IRAS 22491−1808 (2'') | 2.6 ± 0.1 | 1.0 ± 0.2 | 43 ± 2 | 17 ± 3 |
Notes. Column (1): Object name. Columns (2) and (3): Luminosity of the HCN J = 3–2 and HCO+ J = 3–2 emission lines (in units of L⊙), respectively, derived from the spatially integrated (1''–2'') emission line flux (Table 7). Columns (4) and (5): Luminosity of the HCN J = 3–2 and HCO+ J = 3–2 emission lines (in units of K km s−1 pc2), respectively, derived from the spatially integrated (1''–2'') emission line flux (Table 7).
aBased on the peak value in the integrated intensity (moment 0) map.Download table as: ASCIITypeset image
The total molecular mass within a certain volume is often estimated from CO J = 1–0 and/or CO J = 2–1 emission line luminosities (Bolatto et al. 2013). For IRAS 14348−1447 southwest and northeast and IRAS 12112+0305 northeast and southwest, the total molecular mass in the nuclear regions (using the adopted cosmological parameters in Table 1) are estimated to be 12 × 109 M⊙, 7.4 × 109 M⊙, 11 × 109 M⊙, and 3.6 × 109 M⊙, respectively, from pre-ALMA interferometric CO J = 1–0 observations (Evans et al. 2000, 2002). The dense molecular masses estimated from HCN J = 3–2 data (Table 9, column 4) are >5.2 × 109 M⊙, >3.0 × 109 M⊙, >4.8 × 109 M⊙, and >0.3 × 109 M⊙, respectively, where αHCN = 10 is assumed conservatively and the lower limit means that HCN could be subthermally excited at J = 3–2. With the exception of the faint secondary nucleus, IRAS 12112+0305 SW, dense molecular gas masses can account for the bulk of the nuclear total molecular masses. For IRAS 22491−1808, we can estimate the nuclear total molecular gas mass from the ALMA-measured CO J = 2–1 flux of 57.4 ± 0.1 (Jy km s−1) (Pereira-Santaella et al. 2018) to be ∼6 × 109 M⊙, where the conversion factor from CO luminosity in (K km s−1 pc2) to molecular gas mass with αCO = 1.5 (Evans et al. 2002) and optically thick thermal excitation for CO J = 2–1 (Tex ∼ Tkin) are assumed. The dense molecular mass based on HCN J = 3–2 data (Table 9, column 4) is >4.3 × 109 M⊙, which is as high as the total molecular mass in the IRAS 22491−1808 nucleus. Comparisons of the nuclei of these selected ULIRGs suggest that dense gas is the dominant molecular phase in the nuclei of nearby ULIRGs in general.
5.5. Relation between Dense Molecular Line and Infrared Luminosity
For energy sources surrounded by dense molecular gas and dust as in the nuclear regions of ULIRGs, whatever the energy sources are (either buried AGNs and/or nuclear starbursts), HCN and HCO+ emission line luminosities (=mass tracers of dense molecular gas excited by energy sources) are expected to roughly correlate with infrared luminosity (=mass tracer of infrared continuum-emitting dust heated by energy sources) at a first approximation, because these luminosities become higher for more luminous energy sources (Section 2). Their correlation has been found in Galactic star-forming regions and various types of external galaxies (Gao & Solomon 2004a; Wu et al. 2005; Evans et al. 2006; Gracia-Carpio et al. 2008; Ma et al. 2013; Zhang et al. 2014; Liu et al. 2016; Tan et al. 2018; Imanishi et al. 2018). Figure 9 shows a comparison of the HCN J = 3–2 and HCO+ J = 3–2 emission line luminosities measured with 1''–2'' diameter circular apertures, with IRAS-measured infrared luminosity. As the energetically essential nuclear regions (a few kpc) of nearby ULIRGs are covered (Section 2), the bulk of dense molecular gas emission in individual sources is expected to be included in our ALMA measurements.
Figure 9. Comparison of (a) HCN J = 3–2 and (b) HCO+ J = 3–2 emission line luminosities (in K km s−1 pc2) (abscissa), with infrared luminosity (in L⊙) (ordinate). The molecular line luminosities are estimated based on the Gaussian fits in spatially integrated spectra within the 1''–2'' diameter circular apertures (Table 9). The solid straight lines in panels (a) and (b) are the best-fit lines for HCN J = 4–3 (log LIR = 1.00log L'HCN(43) + 3.80) and HCO+ J = 4–3 (log LIR = 1.13log L'HCO+(43) + 2.83) for various types of galaxies (Tan et al. 2018). The dotted straight lines in panels (a) and (b) are the best-fit lines for HCN J = 4–3 (log LIR = 1.00log L'HCN(43) + 3.67) and HCO+ J = 4–3 (log LIR = 1.10log L'HCO+(43) + 2.48) by Zhang et al. (2014). The dashed straight line in panel (a) is the best-fit line for HCN J = 1–0 (log LIR = 1.00log L'HCN(10) + 2.90) (Gao & Solomon 2004b). The same line is overplotted for HCO+ J = 1–0 in panel (b), because the conversion factor from molecular emission line luminosity to dense molecular gas mass is comparable for HCN J = 1–0 and HCO+ J = 1–0 (Leroy et al. 2015, 2017).
Download figure:
Standard image High-resolution imageThe best-fits for HCN J = 4–3, HCO+ J = 4–3, and HCN J = 1–0 (Gao & Solomon 2004b; Zhang et al. 2014; Tan et al. 2018) are plotted together. For subthermal excitation at J = 4–3, the best-fit lines at J = 4–3 should be at the left side of those at J = 1–0. This is the case both for HCN and HCO+. For HCN, our data at J = 3–2 are located to the right of the best-fit lines for J = 4–3 (solid and dotted straight lines in Figure 9(a)), but left of that for J = 1–0 (dashed straight line in Figure 9(a)). These trends can be explained if HCN is subthermally excited at J = 3–2 and J = 4–3, and the deviation below the thermal excitation is larger at J = 4–3 than at J = 3–2. For HCO+, the two best-fit lines for J = 4–3 (Zhang et al. 2014; Tan et al. 2018) are largely different, possibly because of different individual sources used for the fitting; however, our HCO+ J = 3–2 data are located on the left side of the best-fit line for J = 1–0 (dashed straight line in Figure 9(b)). Subthermal HCO+ excitation at J = 3–2 is indicated.
HCN J = 3–2 emission in these ULIRGs is estimated to be subthermally excited, and yet shows higher HCN-to-HCO+ J = 3–2 flux ratios than known less infrared luminous starburst-dominated galaxies (Section 5.1). This suggests that in the comparison starburst sample, the ratio of HCN J = 3–2 excitation temperature to molecular gas kinetic temperature (Tex/Tkin) is even lower (more subthermal further below thermal excitation) than the observed ULIRGs.
5.6. Spatially Integrated Continuum Emission and Spectral Energy Distribution
Table 10 summarizes spatially integrated continuum fluxes for the observed ULIRGs. The same aperture size that is employed for the molecular line flux measurement (Table 7) is used for individual sources. Namely, a circular aperture with a diameter of 1''–2'' is adopted, depending on the synthesized beam size and emission morphology. The spatially integrated continuum fluxes shown in Table 10 are substantially (a factor of 1.5–6) larger than the continuum fluxes at the nuclear peak positions within the beam size listed in Table 3, particularly for sources observed with small (01–0
2 or 150–530 pc) beam sizes, suggesting the presence of a strong, spatially resolved (a few 100 pc to a few kpc) continuum emission component at ∼240 GHz. While the continuum emission from a compact nuclear core should come from an AGN and/or very compact (<500 pc) starbursts, the spatially resolved continuum emission is most likely to come from a few kpc-scale starburst activity. The following three physical mechanisms in starbursts can contribute to the observed spatially resolved continuum fluxes: (1) dust thermal radiation, (2) free–free emission from H ii regions, and (3) synchrotron radiation. Although the third synchrotron radiation component is important at lower frequencies (<20 GHz), ∼240 GHz continuum emission from starbursts is usually dominated by dust thermal radiation and free–free emission. We thus estimated these two components.
Table 10. Spatially Integrated Continuum Flux
Object | Observed Flux | Free–Free Emission |
---|---|---|
(mJy) | (mJy) | |
(1) | (2) | (3) |
IRAS 00188−0856 | 4.0 | ≲0.8 |
IRAS 03250+1606 | 2.6 | ≲0.5 |
IRAS 04103−2838 | 0.71 | ≲0.5 |
IRAS 09039+0503 (1![]() |
3.8 | ≲0.5 |
IRAS 10378+1108 | 3.2 | ≲0.6 |
IRAS 10485−1447 | <2 | ≲0.5 |
IRAS 11095−0238 (1![]() |
4.2 | ≲0.8 |
IRAS 13335−2612 N | 2.3 | ≲0.5 |
IRAS 13335−2612 S | 0.80 | ≲0.5 |
IRAS 14348−1447 SW | 5.2 | ≲1.8 |
IRAS 14348−1447 NE | 3.6 | ≲1.8 |
IRAS 16090−0139 | 5.7 | ≲1.1 |
IRAS 21329−2346 (1![]() |
1.7 | ≲0.5 |
IRAS 23234+0946 | 1.9 | ≲0.5 |
IRAS 00091−0738 | 7.8 | ≲0.7 |
IRAS 00456−2904 | 4.4 | ≲0.8 |
IRAS 01004−2237 | 1.3 | ≲0.6 |
IRAS 01166−0844 SE | 1.9 | ≲0.5 |
IRAS 01298−0744 | 4.5 | ≲0.7 |
IRAS 01569−2939 | 1.9 | ≲0.5 |
IRAS 02411+0353 | <2 | ≲0.4 |
IRAS 10190+1322 | 6.4 | ≲1.1 |
IRAS 11506+1331 | 2.8 | ≲0.8 |
IRAS 13509+0442 | 2.5 | ≲0.5 |
IRAS 22206−2715 (1![]() |
1.8 | ≲0.5 |
IRAS 12112+0305 NE (2'') | 9.8 | ≲2.4 |
IRAS 12112+0305 SW (2'') | 1.0 | ≲2.4 |
IRAS 20414−1651 (1![]() |
5.9 | ≲1.2 |
IRAS 22491−1808 (2'') | 4.9 | ≲1.4 |
Note. Column (1): object name. Column (2): spatially integrated continuum flux at ∼240 GHz (in mJy). A 1'' diameter circular aperture is basically employed. A 15 or 2'' diameter circular aperture is used for a small fraction of sources, which are indicated in column 1. Column (3): estimated free–free emission flux from H ii regions in starbursts at ∼240 GHz (in mJy). Upper limits mean possible AGN contribution to the observed far-infrared luminosity (Section 5.6).
Download table as: ASCIITypeset image
For a small fraction of the observed ULIRGs, there are photometric data by Herschel at 250, 350, and 500 μm (Clements et al. 2018), which are usually dominated by dust thermal radiation. These data, together with IRAS 60 and 100 μm fluxes and our ALMA spatially integrated continuum fluxes at ∼240 GHz (∼1250 μm), are plotted in Figure 10. Although the aperture sizes of the Herschel and IRAS data are much larger, our ALMA measurements roughly agree with the extrapolation from the shorter-wavelength Herschel and IRAS data (Clements et al. 2018), supporting the scenario that nearby ULIRGs are energetically dominated by compact nuclear (<a few kpc) regions (Soifer et al. 2000; Diaz-Santos et al. 2010; Imanishi et al. 2011), and that our ALMA data recover the bulk of the nuclear continuum emission. The ALMA data point in IRAS 11095−0238 (Figure 10(c)) is significantly higher than the extrapolation, which may indicate a significant contribution from additional free–free emission. For IRAS 22491−1808 (Figure 10(g)), the ALMA data point is slightly below the extrapolation, which may be due to the fit because (1) the longest wavelength Herschel data point is also below the fit and (2) the maximum recoverable scale is large (i.e., ALMA missing flux is expected to be small) among the observed ULIRGs (Section 3). For the remaining ULIRGs, such Herschel photometric measurements are not reported, which hampers the reliable estimate of the contribution from the dust thermal radiation at ∼240 GHz.
Figure 10. Spectral energy distribution (SED) at 20–2000 μm for selected ULIRGs for which Herschel photometry at 250, 350, and 500 μm are available (Clements et al. 2018), in addition to the IRAS 60 and 100 μm photometric measurements (Table 1). These data are displayed as filled circles. The data points at ∼1250 μm (open stars) are from our ALMA measurements of spatially integrated continuum fluxes (Table 10). The best-fit gray body curves by Clements et al. (2018) are overplotted as thick curved lines after normalization at the 250 μm flux.
Download figure:
Standard image High-resolution imageOn the other hand, the contributions from the free–free emission in H ii regions can be estimated for all sources, from the far-infrared (40–500 μm) luminosities obtained from IRAS data (Table 1). The flux of the free–free emission from H ii regions (in mJy) is expressed as
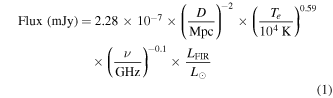
(Nakanishi et al. 2005), where D is the luminosity distance (in Mpc), Te is the electron temperature (in K), ν is the frequency (in GHz), and LFIR is the far-infrared luminosity (in L⊙). In fact, an AGN can also contribute to the far-infrared luminosity, so that the actual free–free continuum flux from H ii regions in starbursts is even smaller. These estimates of the free–free emission are shown in Table 10 (column 3), where Te = 104 K is assumed. In the majority of the observed ULIRGs, the observed continuum flux is significantly higher than the upper limit of the free–free emission from H ii regions in starbursts, suggesting that dust thermal radiation has an important contribution at ∼240 GHz.
No clear continuum emission was detected at the nuclear peak positions of IRAS 10485−1447 and IRAS 02411+0353. For both sources, we obtained continuum fluxes within a 1'' diameter circular aperture of <2 mJy at ∼240 GHz, whereas the estimated free–free continuum fluxes at ∼240 GHz from the far-infrared luminosity measured with IRAS's large aperture (>60'') are <0.5 mJy. The faint continuum fluxes in IRAS 10485−1447 and IRAS 02411+0353, as measured with our ALMA data, do not pose any serious inconsistencies.
For IRAS 10485−1447, radio synchrotron emission is detected with fluxes of 6.45 mJy and 3.37 mJy at 1.4 GHz and 4.8 GHz, respectively (Baan & Klöckner 2006). By fitting these radio data with a power-law function and by extrapolating to our ALMA frequency, the synchrotron emission component at ∼240 GHz is estimated to be ∼0.4 mJy, which is again sufficiently small compared to the observed flux upper limit. It is possible that the observed 1.4 GHz flux is significantly attenuated by free–free absorption. If we make a power-law fit using an intrinsic 1.4 GHz flux, the extrapolated synchrotron emission flux at ∼240 GHz will be even smaller.
6. Summary
We presented our ALMA Cycle 5 observational results of the complete sample of 26 nearby (z < 0.15) ULIRGs with no obvious signatures of optically detectable luminous AGNs in the HCN J = 3–2 and HCO+ J = 3–2 lines. The sample was selected from the IRAS 1 Jy sample, based on the decl. of <+20° and infrared flux above a certain threshold to secure sufficiently high nuclear dense molecular emission line peak flux. The majority of the ULIRGs were observed with a 01–0
2 (150–530 pc) synthesized beam size; 0
5–0
9 (0.9–1.7 kpc) was used for the remaining small fraction of ULIRGs. We found the following main results.
- 1.The continuum, HCN J = 3–2, and HCO+ J = 3–2 emission lines were spatially resolved in the majority of the observed ULIRGs. The HCN J = 3–2 and continuum emission sizes are roughly comparable; however, HCO+ J = 3–2 emission is spatially more extended than the HCN J = 3–2 emission. This can naturally be explained by the smaller critical density of HCO+ J = 3–2 than that of HCN J = 3–2, where low-density and temperature molecular gas in spatially resolved regions outside compact nuclear cores can collisionally excite HCO+ to J = 3 more than HCN and produce stronger HCO+ J = 3–2 emission there.
- 2.The HCN-to-HCO+ J = 3–2 flux ratios at the compact nuclear cores of the ULIRGs, within the beam size are generally higher than (a) unity, (b) those seen in less infrared luminous starburst-dominated galaxies, and (c) those in spatially integrated areas of the same ULIRGs measured with 1''–2'' diameter circular apertures. For ULIRGs observed with small synthesized beam sizes with 0
1–0
2, we created the maps of the HCN-to-HCO+ J = 3–2 flux ratios and investigated their spatial variation. In the majority of the ULIRGs, the regions of elevated HCN-to-HCO+ J = 3–2 flux ratios spatially coincide with nuclear cores. It is likely that the putative buried AGNs and/or high HCN collisional excitation by dense and warm molecular gas at the nuclear cores are responsible for the enhanced HCN emission there. Mechanical heating by shocks originated in spatially compact nuclear outflows can also contribute.
- 3.Several ULIRGs show higher HCN-to-HCO+ J = 3–2 flux ratios at off-nuclear local regions than the nuclear cores, which is difficult to explain with the above scenario. Mechanical heating by spatially extended outflow-origin shock activity may be responsible.
- 4.We estimated that, in the majority of the observed ULIRGs, dense (>105 cm−3) molecular gas mass within the nuclear few kpc regions is as high as >109 M⊙, based on the observed HCN J = 3–2 and HCO+ J = 3–2 emission line luminosities, suggesting that nearby ULIRG's nuclei are dense molecular gas rich. For selected ULIRGs with available nuclear total molecular masses based on interferometric CO J-transition line data, dense molecular masses can be as high as the nuclear total molecular masses in most cases, suggesting that dense gas is a dominant molecular phase in nearby ULIRG's nuclei.
- 5.A comparison of the HCN J = 3–2 and HCO+ J = 3–2 emission line luminosities with infrared luminosity suggests that HCN and HCO+ are only subthermally excited at J = 3–2. Even with this subthermal HCN J = 3–2 excitation in ULIRGs, their HCN-to-HCO+ J = 3–2 flux ratios are higher than starburst galaxies. The ratios of HCN J = 3–2 excitation temperature to molecular gas kinetic temperature (Tex/Tkin) in the comparison starburst sample are suggested to be even smaller than the ULIRG sample.
- 6.Signatures of the excess emission at the lower-frequency side of the HCO+ J = 3–2 (v = 0) emission lines are recognizable in IRAS 10378+1108 and IRAS 11095−0238, in addition to three ULIRGs reported in Imanishi et al. (2016c; IRAS 12112+0305, IRAS 20414−1651, and IRAS 22491−1808), out of the 26 observed ULIRGs. The excess emission can be due to contributions from vibrationally excited (v2 = 1f) HCN J = 3–2 emission lines. The HCN v2 = 1f to v = 0 flux ratios at J = 3–2 could be as high as an AGN-hosting ULIRG with a clearly detected HCN v2 = 1f J = 3–2 emission line. If the HCN vibrational excitation to v2 = 1f is due to infrared radiative pumping, by absorbing infrared ∼14 μm photons coming from AGN-heated hot dust grains, these five ULIRGs may contain (sub)millimeter-detectable deeply buried AGNs. The low detection rate (5/26 ∼ 19%) of the signatures of the HCN v2 = 1f J = 3–2 emission lines may be due to the combination of limited signal-to-noise ratios and large molecular line widths in ULIRGs that hamper the identification of faint HCN v2 = 1f J = 3–2 emission lines by clearly separating from the nearby much brighter HCO+ J = 3–2 (v = 0) emission lines. Signatures of the HCO+ v2 = 1f J = 3–2 emission lines were not seen in any of the observed ULIRGs.
- 7.High HCN-to-HCO+ J = 3–2 flux ratios are found in (a) all ULIRGs with significant (>20%) energetic contributions from infrared spectroscopically identified luminous buried AGNs and (b) some fraction of ULIRGs without such infrared-identified luminous buried AGNs. Although the HCN v2 = 1f J = 3–2 emission lines (which is another potential AGN indicator) were not clearly detected in the majority of these ULIRGs, if the observed high HCN-to-HCO+ J = 3–2 flux ratios are due to AGN effects (including both AGN radiation feedback and mechanical feedback by AGN-origin compact nuclear outflows), then our (sub)millimeter molecular line method (a) provides a consistent picture with the infrared spectroscopic method about the presence of energetically significant luminous buried AGNs for the former ULIRGs and (b) may suggest the presence of infrared-elusive but (sub)millimeter-detectable extremely deeply buried luminous AGNs for the latter ULIRGs.
- 8.Molecular outflows have usually been discussed based on the detection of broad components in the bright CO emission lines, but no such broad components were clearly seen in the HCN J = 3–2 and HCO+ J = 3–2 emission lines in the observed ULIRGs.
- 9.Spatially integrated continuum fluxes at ∼240 GHz are significantly higher than the estimated free–free emission fluxes from H ii regions in spatially resolved (a few kpc) starbursts, suggesting that thermal radiation from dust contributes significantly to the continuum flux in band 6 (∼240 GHz).
We thank Dr. K. Saigo and F. Egusa for their supports regarding ALMA data analysis and the anonymous referee for their valuable comments that helped improve the clarity of this manuscript. M.I. is supported by JSPS KAKENHI grant No. 15K05030. This paper made use of the following ALMA data: ADS/JAO.ALMA#2017.1.00057.S, 2013.1.00032.S, and 2015.1.00027.S. ALMA is a partnership of ESO (representing its member states), NSF (USA) and NINS (Japan), together with NRC (Canada), NSC and ASIAA (Taiwan), and KASI (Republic of Korea), in cooperation with the Republic of Chile. The Joint ALMA Observatory is operated by ESO, AUI/NRAO, and NAOJ. Data analysis was in part carried out on the open use data analysis computer system at the Astronomy Data Center, ADC, of the National Astronomical Observatory of Japan. This research has made use of NASA's Astrophysics Data System and the NASA/IPAC Extragalactic Database (NED), which is operated by the Jet Propulsion Laboratory, California Institute of Technology, under contract with the National Aeronautics and Space Administration.
Facility: ALMA. -
Appendix A: Comments on Continuum Emission for Selected Galaxies
For IRAS 04103−2838, the HCN J = 3–2 and HCO+ J = 3–2 emission line peaks are significantly displaced from the continuum peak (04 12 19.445, −28 30 25.00) ICRS (Figure 1(c)). The spectrum in Figure 2(c) was extracted at the peak position of the HCN J = 3–2 and HCO+ J = 3–2 emission lines.
IRAS 09039+0503 is a single-nucleus ULIRG in seeing-sized optical and near-infrared K-band (2.2 μm) images (Kim et al. 2002). However, our high-spatial-resolution ALMA molecular line and continuum data reveal double nuclear morphology (southwest and northeast), with ∼05 separation in Figures 1(d), 3(g), and 3(h). The spectra shown in Figures 2(d) and (e) were extracted for both nuclei separately.
IRAS 11095−0238 is also a single-nucleus ULIRG in the optical and near-infrared (Kim et al. 2002); however, our ALMA data show a faint secondary emission component in the ∼05 southwestern part of the main nucleus in the continuum and HCO+ J = 3–2 line maps (Figures 1(f) and 3(l)). The HCO+ J = 3–2 emission line shows a significant feature in-between these two continuum-emitting regions in IRAS 11095−0238 (Figure 1(f)).
For IRAS 01166−0844, continuum and molecular line emission were detected at the southeast nucleus, but not at the northwest nucleus (Kim et al. 2002).
For IRAS 10485−1447, neither the continuum nor HCN J = 3–2 and HCO+ J = 3–2 emission lines were detected. No detectable CO J = 1–0 emission line was seen in pre-ALMA interferometric observations (Dinh-V-Trung et al. 2001). Thus, IRAS 10485−1447 may be deficient in molecular gas.
Appendix B: Comments on Selected Galaxies
In Figure 11, the best Gaussian fits for the detected molecular emission lines are overplotted on the actual data.
Figure 11.
Gaussian fit of the detected emission line in Figure 2. The solid curved line is the best Gaussian fit, and the dotted straight line is the zero flux level. (An extended version of this figure is available.)
Download figure:
Standard image High-resolution imageAppendix C: Intensity-weighted Mean Velocity and Intensity-weighted Velocity Dispersion Maps
Figures 12 and 13 display intensity-weighted mean velocity (moment 1) and intensity-weighted velocity dispersion (moment 2) maps of HCN J = 3–2 and HCO+ J = 3–2 emission lines of selected ULIRGs, for which we can obtain meaningful dynamical information of these dense gas tracers (mostly >10σ detection in their integrated intensity (moment 0) maps). For most of the ULIRGs with spatially resolved molecular line emission, a rotation pattern with blueshifted and redshifted components is visible (Figure 12).
Download figure:
Standard image High-resolution imageFigure 12. Intensity-weighted mean velocity (moment 1) map of the HCN J = 3–2 and HCO+ J = 3–2 emission lines for selected ULIRGs with sufficiently high detection significance. The abscissa and ordinate are R.A. and decl. in ICRS, respectively. The contours are 38,500 and 38,600 km s−1 for IRAS 00188−0856 HCN J = 3–2 and HCO+ J = 3–2, 40,940 km s−1 for IRAS 10378+1108 HCN J = 3–2 and HCO+ J = 3–2, 31,950 km s−1 for IRAS 11095−0238 HCN J = 3–2 and HCO+ J = 3–2, 24,770 and 24,840 km s−1 for IRAS 14348−1447 SW HCN J = 3–2, 24,750 and 24,900 km s−1 for IRAS 14348−1447 SW HCO+ J = 3–2, 24,695 and 24,760 km s−1 for IRAS 14348−1447 NE HCN J = 3–2, 40,060 km s−1 for IRAS 16090−0139 HCN J = 3–2, 40,040 km s−1 for IRAS 16090−0139 HCO+ J = 3–2, 37,400 and 37,530, 37,630 km s−1 for IRAS 21329−2346 HCN J = 3–2 and HCO+ J = 3–2, 32,910 and 33,010 km s−1 for IRAS 00456−2904 HCN J = 3–2, 32,880 and 32,950 km s−1 for IRAS 00456−2904 HCO+ J = 3–2, 35,360 km s−1 for IRAS 01004−2237 HCN J = 3–2, 35,335 and 35,385 km s−1 for IRAS 01004−2237 HCO+ J = 3–2, 35,080 and 35,180 km s−1 for IRAS 01166−0844 SE HCN J = 3–2, 35,200 km s−1 for IRAS 01166−0844 SE HCO+ J = 3–2, 41,040 km s−1 for IRAS 01298−0744 HCN J = 3–2, 40,990 and 41,070 km s−1 for IRAS 01298−0744 HCO+ J = 3–2, 42,000 and 42,200 km s−1 for IRAS 01569−2939 HCN J = 3–2, 41,920 and 42,120 km s−1 for IRAS 01569−2939 HCO+ J = 3–2, 22,740 and 22,880 km s−1 for IRAS 10190+1322 HCN J = 3–2, 22,710 and 22,820 km s−1 for IRAS 10190+1322 HCO+ J = 3–2, 38,150 km s−1 for IRAS 11506+1331 HCN J = 3–2, 38,080 and 38,180 km s−1 for IRAS 11506+1331 HCO+ J = 3–2, 39,460 and 39,510 km s−1 for IRAS 22206−2715 HCN J = 3–2, and 39,530 km s−1 for IRAS 22206−2715 HCO+ J = 3–2. For IRAS 14348−1447 NE, the map of the faint HCO+ emission line is not shown. The moment 1 maps of IRAS 12112+0305 NE, IRAS 20414−1651, and IRAS 22491−1808 were found in Imanishi et al. (2016c) and are not presented here. Beam sizes are shown as open circles in the lower-left region. An appropriate cutoff is applied to prevent the resulting maps from being dominated by noise.
Download figure:
Standard image High-resolution imageDownload figure:
Standard image High-resolution imageFigure 13. Intensity-weighted velocity dispersion (moment 2) map of the HCN J = 3–2 and HCO+ J = 3–2 emission lines for selected ULIRGs with sufficiently high detection significance. The abscissa and ordinate are R.A. and decl. in ICRS, respectively. The contours are 60 and 85 km s−1 for IRAS 00188−0856 HCN J = 3–2, 60 and 80 km s−1 for IRAS 00188−0856 HCO+ J = 3–2, 60 and 90 km s−1 for IRAS 10378+1108 HCN J = 3–2, 60 and 85 km s−1 for IRAS 10378+1108 HCO+ J = 3–2, 75 km s−1 for IRAS 11095−0238 HCN J = 3–2, 44 km s−1 for IRAS 11095−0238 HCO+ J = 3–2, 55 and 83 km s−1 for IRAS 14348−1447 SW HCN J = 3–2, 47 and 65 km s−1 for IRAS 14348−1447 SW HCO+ J = 3–2, 90 km s−1 for IRAS 14348−1447 NE HCN J = 3–2, 80 and 130 km s−1 for IRAS 16090−0139 HCN J = 3–2, 90 and 120 km s−1 for IRAS 16090−0139 HCO+ J = 3–2, 102 and 130 km s−1 for IRAS 21329−2346 HCN J = 3–2, 100 and 130 km s−1 for IRAS 21329−2346 HCO+ J = 3–2, 55 and 72 km s−1 for IRAS 00456−2904 HCN J = 3–2, 36 and 50 km s−1 for IRAS 00456−2904 HCO+ J = 3–2, 30 and 42 km s−1 for IRAS 01004−2237 HCN J = 3–2, 34 and 48 km s−1 for IRAS 01004−2237 HCO+ J = 3–2, 65 and 85 km s−1 for IRAS 01166−0844 SE HCN J = 3–2, 40 and 80 km s−1 for IRAS 01166−0844 SE HCO+ J = 3–2, 50 and 72 km s−1 for IRAS 01298−0744 HCN J = 3–2, 40 and 60 km s−1 for IRAS 01298−0744 HCO+ J = 3–2, 120 and 156 km s−1 for IRAS 01569−2939 HCN J = 3–2, 120 and 164 km s−1 for IRAS 01569−2939 HCO+ J = 3–2, 49 and 70 km s−1 for IRAS 10190+1322 HCN J = 3–2, 45 km s−1 for IRAS 10190+1322 HCO+ J = 3–2, 42 km s−1 for IRAS 11506+1331 HCN J = 3–2, 45 km s−1 for IRAS 11506+1331 HCO+ J = 3–2, 75 and 96 km s−1 for IRAS 22206−2715 HCN J = 3–2, and 74 and 91 km s−1 for IRAS 22206−2715 HCO+ J = 3–2. For IRAS 14348−1447 NE, the map of the faint HCO+ emission line is not shown. The moment 2 maps of IRAS 12112+0305 NE, IRAS 20414−1651, and IRAS 22491−1808 were found in Imanishi et al. (2016c) and are not presented here. Beam sizes are shown as open circles in the lower-left region. An appropriate cutoff is applied.
Download figure:
Standard image High-resolution imageAppendix D: A Continuum-emitting Source within the IRAS 13509+0442 Field
A bright continuum-emitting source was detected at the ∼10'' northern side of IRAS 13509+0442 at ∼235 GHz and ∼310 GHz (Imanishi et al. 2016c, 2018). This source is considered a distant submillimeter galaxy (SMG) candidate (Imanishi et al. 2016c, 2018). We made a clean map with a wider field of view (1024 × 1024 pixels of 002 pixel−1) to investigate this source in our ALMA Cycle 5 high-spatial-resolution data.
A continuum map created using all channels (because there are no obvious emission lines in this source) is displayed in Figure 14 (left). The continuum emission is clearly detected with the peak flux of 0.84 (mJy beam−1, 16σ) and is spatially resolved with the beam size of 220 (mas) × 160 (mas, position angle = 29° east of north). The estimated intrinsic deconvolved emission size is 421 ± 43 (mas) × 323 ± 35 (mas) (position angle is 56° ± 23° east of north). The spatially integrated continuum flux within a 1'' diameter circular aperture is 3.6 (mJy). Figure 14 (right) shows a spectrum without continuum subtraction at the peak position within the beam size. No significant emission line is recognizable.
Figure 14. Continuum image (left) and spectrum without continuum subtraction at the nuclear peak position within the beam size (right) of the bright continuum-emitting source at ∼10'' northern side of IRAS 13509+0442 (Imanishi et al. 2016c, 2018). The contours represent 4σ, 10σ, and 15σ (1σ = 0.054 mJy beam−1) in the left panel.
Download figure:
Standard image High-resolution imageFootnotes
- 3
A decreased HCO+ abundance is another possibility and is argued in highly turbulent regions (Papadopoulos 2007). However, we regard that this could happen in wide areas of merging ULIRGs rather than only at the compact nuclear cores.