Abstract
Star-forming dwarf galaxies have properties similar to those expected in high-redshift galaxies. Hence, these local galaxies may provide insights into the evolution of the first galaxies and the physical processes at work. We present a sample of 11 potential local analogs to high-z (LAHz) galaxies. The sample consists of blue compact dwarf galaxies, selected to have spectral energy distributions that fit galaxies at 1.5 < z < 4. We use SOFIA-HAWC+ observations combined with optical and near-infrared data to characterize the dust properties, star formation rate (SFR), and star formation histories (SFHs) of the sample of LAHz galaxies. We employ Bayesian analysis to characterize the dust using two-component blackbody models. Using the Lightning package, we fit the spectral energy distribution of the LAHz galaxies over the far-UV−far-infrared wavelength range and derive the SFH in five time steps up to a look-back time of 13.3 Gyr. Of the 11 LAHz candidates, six galaxies have SFH consistent with no star formation activity at look-back times beyond 1 Gyr. The remaining galaxies show residual levels of star formation at ages ≳1 Gyr, making them less suitable as local analogs. The six young galaxies stand out in our sample by having the lowest gas-phase metallicities. They are characterized by warmer dust, having the highest specific SFR and the highest gas mass fractions. The young age of these six galaxies suggests that merging is less important as a driver of the star formation activity. The six LAHz candidates are promising candidates for studies of the gasdynamics role in driving star formation.
Export citation and abstract BibTeX RIS
1. Introduction
The high-redshift (high-z) universe is very different from our local environment: galaxies are physically small with irregular morphologies; they are metal-poor, star formation rates (SFRs) are high and growing exponentially, and the interstellar gas constitutes a major component of the total baryonic mass. However, the faintness and the small sizes make it extremely difficult to study the physical processes in these galaxies in detail. Instead, a significant effort over the past decade has been devoted to identifying low-redshift (low-z) analogs to high-z galaxies (e.g., Bian et al. 2016; Sebastian & Bait 2019). These analogs to high-z galaxies are usually local low-metallicity, star-forming dwarf galaxies. They share many properties of high-redshift galaxies in terms of morphology, physical size, metallicity, and star formation (e.g., Adamo et al. 2011; Kaaret et al. 2011; Östlin et al. 2014). This provides an attractive route to study the properties of high-z galaxies in better detail.
Several previous studies have defined analogs to high-z galaxies using a variety of approaches. For instance, Heckman et al. (2005) and Hoopes et al. (2007) found that ultraviolet-luminous galaxies (UVLGs) with high surface brightness have characteristics that are remarkably similar to Lyman break galaxies (LBGs) at high z. UVLGs are rare in the local universe, but a significant sample can be defined when extending the search to z ∼ 0.2. These Lyman break analogs have LUV ≳ 2 × 1010 L⊙, SFRs of 3–30 M⊙ yr−1, sizes of a few kiloparsecs, and subsolar metallicities. In a similar manner, Overzier et al. (2011) define analogs of high-z LBGs based on their UV luminosity. Östlin et al. (2014) use Hα equivalent widths as a requirement to identify local galaxies that have Lyα luminosities comparable to high-redshift Lyα emitters (LAEs) and LBGs (the Lyα Reference Sample, or LARS). The galaxies composing LARS are small, metal-poor, ∼0.2–0.6 Z⊙, and gas-rich, with an average gas mass fraction ∼0.4 and SFRs ranging from 0.6 to ∼20 M⊙ yr−1. They are morphologically identified as dwarf irregular galaxies (Pardy et al. 2014). Interestingly, most of the LAE and LBG analogs defined by Östlin et al. (2014) are also far-infrared (FIR) bright, showing the presence of significant amounts of dust in their interstellar medium (ISM), including one galaxy with an Lyα escape fraction of ∼12%.
Another proposed analog for high-redshift LAEs is the class of Green Peas (Cardamone et al. 2009). These are compact, low-mass galaxies with subsolar metallicities. They have SFRs of ∼4 M⊙ yr−1 but very high specific SFRs (sSFRs; Izotov et al. 2011). Their properties overlap with blue compact dwarf galaxies (BCDGs) at the high-luminosity end of BCDGs, but they are otherwise distinct. Green Peas exhibit starburst-driven outflows (Yang et al. 2017), and many have a high Lyα escape fraction (Kim et al. 2021). The typical distance of Green Peas is z ∼ 0.2–0.3.
Although these analogs to high-z galaxies are "local" in a cosmological sense, they are still quite distant as far as detailed studies are concerned. The UVLGs and Green Peas are at z ∼ 0.2–0.3, or DA ≳ 700 Mpc, while the average distance to galaxies in the LARS sample is ∼300 Mpc. In linear scale, this corresponds to ≳3.3 and 1.5 kpc arcsec−1, respectively. The relatively large distances are necessary because of the low number densities of galaxies fulfilling the criteria for being selected as an LBG and/or LAE analog.
In this paper we present an alternative approach to define local analogs, which allows us to select nearby galaxies at distances ∼10–20 Mpc. This means that scales of 50 pc can be resolved even with ground-based instruments. Instead of matching properties like luminosity and/or equivalent widths of emission lines, this approach relies on how well the entire UV to near-infrared (NIR) spectral energy distributions (SEDs) of local galaxies match those of high-z galaxies. Using templates of local galaxies spanning a wide variety of morphology, star formation activity, gas-phase metallicity, and active galactic nucleus (AGN) activity and environment, we identify those templates that are most successful in fitting high-redshift galaxies as potential local analogs to high-z (LAHz) galaxies. The sample of LAHz galaxies presented in this work is selected from 129 local galaxy spectral templates compiled by Brown et al. (2014). These templates are fit to ∼180,000 high-redshift galaxies in the Cosmic Assembly Near-infrared Deep Extragalactic Legacy Survey (CANDELS) fields. It turns out that only a small number of the local templates provide the best-fit SED to galaxies at z > 2. All the local templates in this category are designated as BCDGs. The LAHz galaxies are thus scaled-down versions of star-forming high-redshift galaxies.
All of the LAHz galaxies, as well as most of the galaxies in the Lyα Reference Sample (Östlin et al. 2014), are detected at FIR wavelengths, suggesting thermal dust emission. The connection between star formation activity and dust content in galaxies is well established and provides information about the evolutionary stage of galaxies. In a study involving ∼1600 low-redshift galaxies, da Cunha et al. (2010) found a strong correlation between the dust-to-stellar mass ratio and the sSFR, as well as a strong correlation between the dust-to-SFR ratio and sSFR. These correlations suggest an evolutionary sequence and can be used as diagnostics for inferring the evolutionary stage of galaxies in combination with other parameters, like the star formation history (SFH).
Observations with the Atacama Large Millimeter Array (ALMA) over the past few years have vastly increased our understanding of the cold and dense ISM of galaxies in the early universe. Star-forming galaxies on the main sequence (MS) at z ∼ 3 appear to have dust attenuation properties similar to large and massive star-forming galaxies in our local universe (Fudamoto et al. 2017). Recent results from the ALPINE survey (Le Fèvre et al. 2020), however, suggest that the dust attenuation decreases drastically for galaxies on the MS at z ≳ 4 (Fudamoto et al. 2020), approaching that of metal-poor dwarf galaxies in the local universe.
The shape of the FIR dust SED of high-z galaxies has largely remained unconstrained, as most rest-frame FIR observations at high z consist of a single photometric data point. This is also changing thanks to data from ALMA. The FIR SED can now be constructed for galaxies at z ∼ 2, where Pantoni et al. (2021) find luminosity-weighted dust temperatures ∼50 K for 11 galaxies on the MS. Faisst et al. (2020) measured the dust SED for a handful of MS galaxies at z ≳ 4, showing that the peak temperatures characterizing the dust SEDs are warmer than for star-forming galaxies in the local universe.
In this paper we provide a detailed characterization of the dust in our sample of LAHz galaxies. We present new SOFIA-HAWC+ observations, which, together with ancillary data, allow us to define the dust SED in detail. This is used to evaluate whether they share properties with galaxies at high redshift, or whether they are similar to local massive star-forming galaxies.
This paper is organized as follows: In Section 2 we describe the sample definition and selection method. In Section 3 we describe our SOFIA observations and ancillary data used. In Section 4 we describe the two modeling procedures used to characterize the dust continuum and the far-UV (FUV) to FIR SED. In Section 5 we present the dust properties and SFHs, and in Section 6 we discuss the implications of these results. In Section 7 we summarize our results and conclusions about the properties of our sample of LAHz galaxies.
2. Sample Definition
Our sample of LAHz galaxies is defined by fitting spectral templates of local galaxies to H-band-selected galaxies in the CANDELS survey (Grogin et al. 2011; Koekemoer et al. 2011) and selecting those templates that provide the best fit to z ≳ 2 galaxies as candidates of being local analogs.
We use the galaxy templates presented in Brown et al. (2014). This set of templates contains SEDs covering a wavelength range from UV to the mid-infrared (MIR) for 129 nearby galaxies. The templates span a wide range of morphologies and galaxy luminosities; they include ellipticals, lenticulars, spirals, and irregular and dwarf galaxies. The sample also contains gravitationally interacting galaxies in various stages of mergers, galaxies with an AGN, and luminous infrared galaxies. The templates combine ground-based optical spectroscopy with NIR and MIR spectroscopy from Spitzer and AKARI. The wavelength coverage is not contiguous, and gaps are filled in using MAGPHYS (Multi-wavelength Analysis of Galaxy Physical Properties code) modeling (da Cunha et al. 2008). The SED from different spectral inputs is normalized and verified with 26 bands of matched-aperture photometry. This allows the mitigation of systematic errors, such as scattered light in IRAC images and errors in the pre-launch WISE W4 filter curve (see Brown et al. 2014 for details).
For the purpose of establishing which types of templates provide the best-fit SEDs to the high-redshift galaxies, we divide the 129 templates into four T-class groups (de Vaucouleurs et al. 1991): −6 ≤ T < −2, −2 ≤ T < 2, 2 ≤ T <8, and 8 ≤ T ≤ 11. These four groups correspond to spheroidal, bulge-dominated, star-forming disk, and star-forming irregular galaxies. Galaxies without a designated morphology class are put in T-class 12, peculiar galaxies. The numbers of templates in the four main groups are 22, 24, 44, and 20, respectively. In addition, the T = 12 peculiar group contains 19 templates.
2.1. High-redshift Data
The Brown et al. (2014) templates are used to fit to the photometry of high-redshift galaxies from CANDELS. CANDELS is a treasury program on Hubble Space Telescope (HST) and provides deep multiwavelength imaging data in five legacy deep fields: GOODS-South, GOODS-North, UDS, COSMOS, and EGS (Grogin et al. 2011; Koekemoer et al. 2011). In this study we use H-band-selected catalogs from all five fields. Details on the data and photometry at different wavelength bands are given for GOODS-S (Guo et al. 2013; Santini et al. 2015), GOODS-N (Barro et al. 2019), UDS (Galametz et al. 2013; Santini et al. 2015), COSMOS (Nayyeri et al. 2017), and EGS (Stefanon et al. 2017).
The CANDELS catalogs contain photometric data from the UV to NIR and MIR wavelengths, observed in broadband, medium-band, and, in some cases, narrowband filters. The catalogs also contain derived physical parameters, such as photometric redshift, stellar mass, and SFRs, compiled using several different teams applying different SED methods, SFHs, and templates (e.g., Dahlen et al. 2013; Mobasher et al. 2015; Santini et al. 2015). The optical (HST/ACS) and NIR (HST/WFC3) data are consistently combined with the MIR data (Spitzer/IRAC) and ground-based observations (UV and K band). All of the photometric catalogs were selected in the HST/WFC3 F160W band using SExtractor (Bertin & Arnouts 1996). The catalogs for the five different CANDELS fields contain a total of 185,956 galaxies. The number of photometric bands ranges from 18 (GOODS-S) to 42 (CANDELS COSMOS). The latter catalog includes extensive narrow- and medium-band photometric bands. The MIR data are from Spitzer/IRAC (Ashby et al. 2013).
2.2. SED Fitting
All 129 of the Brown et al. (2014) templates are used in the SED fitting of the 185,956 CANDELS galaxies. The fits are done in two different modes: keeping the extinction equal to the intrinsic dust extinction of the templates, and allowing additional extinction on top of the intrinsic. The additional extinction is parameterized through the E(B − V) color index. When treated as a free parameter, we allowed additional dust extinction up to E(B − V) = 0.6. We use the Calzetti et al. (2000) attenuation law for the additional extinction. We do not include "negative extinction" in the SED fitting. The Brown et al. (2014) sample contains galaxies with a wide range of metallicities and dust content and should be representative for galaxies with metallicities down to the levels expected for moderately massive galaxies at high redshifts. As shown below, the results in terms of morphology fraction seem to be insensitive to the inclusion of additional extinction (Figure 1). An initial test was done keeping the photometric redshift as a free parameter. The redshifts from the SED fits were similar to those presented in the CANDELS catalogs. For the reminder of the analysis, we keep the redshift fixed at the CANDELS values, which includes spectroscopic redshifts whenever available.
Figure 1. The morphology fraction of the Brown et al. (2014) templates, divided into four groups based on morphological T class. The fraction for each redshift interval represents the best-fit template to CANDELS H-band-selected galaxies from all five CANDELS fields. The left panel represents the fractions where we allow additional dust extinction on top of that already implicit in the templates. In the right panel we only use the templates, without any additional extinction. The dotted blue line represents the fraction of the 11 templates selected to be LAHz galaxies. The dotted black line is the sum of the two earliest morphological classes, representing quiescent spheroidal galaxies.
Download figure:
Standard image High-resolution imageThe SED fitting procedure involved redshifting the template to the redshift of each galaxy, and then each galaxy template is convolved with the filter response functions appropriate for a given CANDELS data set. The template is then fitted to the observed flux densities using χ2 minimization. If additional extinction is included, we repeat this process for E(B − V) values ranging from 0.0 to 0.6, in steps of 0.01.
We divide the CANDELS galaxies into 13 redshift bins between 0.3 ≤ z ≤ 5 (Δz = 0.36). The total number of galaxies in each redshift bin ranges from ∼2000 at z ∼ 0.3 to ∼10,000 at z ∼ 2. For each redshift bin we derive the number of best-fit SEDs for each morphological group as defined above. In Figure 1 we plot the fraction of best-fit SEDs for the four morphological classes as a function of redshift. From the figure it is clear that the irregular class (6 ≤ T ≤ 11) provides about 35% of the best-fit templates at z ∼ 0.3 and increases to dominate at z ≳ 1. At z ≳ 2, these templates account for 85%–90% of all best-fit templates. Of the 20 templates in the 6 ≤ T ≤11 morphological class, 11 of them provide the most frequent best-fit SED (dotted line in Figure 1). These 11 galaxies are selected as our sample of potential local analog candidates.
In order to test the robustness of the morphological fractions shown in Figure 1, we selected the template galaxy with relative to the best-fit SED. The resulting morphological fraction as a function of redshift is shown in Figure 2. At z ≳ 1.5, the fraction of the 6 ≤ T ≤ 11 morphological class decreases slightly, while the fraction of star-forming spiral disks increases. However, the overall dominance of irregular galaxies as the best templates for high-redshift galaxies remains. This shows that the result is robust, and local templates with T-class morphologies corresponding to irregular star-forming galaxies are best at fitting the SED of high-redshift galaxies at z ≳ 1.
Figure 2. Similar to Figure 1. Here the dashed lines correspond to the best-fit SED to CANDELS galaxies when selecting those with relative to the best-fit SEDs shown in Figure 1 (shown as solid lines). The fraction of irregular galaxies decreases slightly, while the fraction of spiral disk galaxies increases. The overall dominance of irregular galaxies in the best-fit SEDs is, however, retained.
Download figure:
Standard image High-resolution imageThis result is not surprising, as galaxies in the 6 ≤ T ≤11 morphological class share many properties with star-forming galaxies at high redshift (e.g., Elmegreen et al. 2009, 2012). The general properties of the 11 candidates are summarized in Table 1; they have small physical sizes (1–2 kpc), they are actively forming stars, and they typically have a high gas mass fraction. Their gas-phase metallicity is low, with an average metallicity of 12 + logO/H = 8.11 ± 0.20, or ∼0.26 Z⊙. In Figure 3 we show the Sloan Digital Sky Survey (SDSS) i-band images of the 11 candidates. From these images it is clear that they have irregular morphology with prominent star-forming clumps.
Table 1. Properties of the Local Analogs
No. | Galaxy | R.A. | Decl. | D |
![]() |
![]() | fgas c | Metallicity d | Alternative |
---|---|---|---|---|---|---|---|---|---|
Name | J2000.0 | (Mpc) | (M⊙) | (M⊙) | M⊙ |
![]() | Name | ||
1 | NGC 2537 | 08:13:14.4 | +45:59:13 | 8.6 | 9.02 | 8.43 | 0.17 | 8.19 | Arp 6; "Bear Paw" |
2 | Mrk 140 | 10:16:28.3 | +45:19:18 | 27.6 | 8.60 | 8.90 | 0.56 | 8.30 | Mrk 140 |
3 | Haro 02 | 10:32:31.9 | +54:24:02 | 23.7 | 9.21 | 8.73 | 0.25 | 8.45 | Mrk 033 |
4 | Mrk 1450 | 11:38:35.6 | +57:52:27 | 14.7 | 7.27 | 7.35 | 0.54 | 7.96 | |
5 | UM 461 | 11:51:33.1 | −02:22:22 | 20.7 | 7.37 | 8.47 | 0.96 | 7.78 | |
6 | Mrk 1307 | 11:52:37.4 | −02:28:09 | 21.0 | 8.07 | 8.73 | 0.81 | 7.96 | UM 462 |
7 | Haro 06 | 12:15:18.4 | +05:45:39 | 35.1 | 8.58 | 8.83 | 0.69 | 8.18 | |
8 | NGC 4670 | 12:45:17.1 | +27:07:31 | 20.0 | 9.38 | 9.02 | 0.37 | 8.30 | Arp 163 |
9 | Mrk 475 | 14:39:05.5 | +36:48:21 | 10.9 | 6.95 | 6.62 | 0.56 | 7.93 | |
10 | UGCA 410 | 15:37:04.2 | +55:15:48 | 10.5 | 7.26 | 7.58 | 0.57 | 8.10 | Mrk 487 |
11 | Mrk 930 | 23:31:58.6 | +28:56:50 | 77.5 | 8.89 | 9.51 | 0.74 | 8.08 |
Notes.
a Stellar mass M*; this work. b H i data from Paturel et al. (2003), except for Mrk 1450 (van Driel et al. 2016) and Haro 02 (Bravo-Alfaro et al. 2004). c Gas fraction is defined as fg = Mg /(Mg + M*). d Metal Abundances UM 461 and UM 462 (Campos-Aguilar et al. 1993), Haro 02 (Davidge 1989).Download table as: ASCIITypeset image
Figure 3. SDSS i-band images of our sample. All these galaxies are classified as BCDGs. The bar corresponds to 1 kpc.
Download figure:
Standard image High-resolution imageAll 11 galaxies fall under the classification of BCDGs (e.g., Sargent & Searle 1970; Kunth et al. 1988; Thuan & Izotov 2005). BCDGs have previously been proposed to be analogs of high-redshift galaxies (e.g., Hoopes et al. 2007). As a group they share many properties, such as small sizes, clumpy, and intense star formation. They show strong optical emission lines, often exhibiting high excitation (Thuan & Izotov 2005). There are, however, differences among the BCDGs; some show the star-forming regions superposed on faint extended stellar emission, suggesting star formation extended over longer time periods (e.g., Noeske et al. 2003), while others seem to be truly young systems (e.g., Papaderos et al. 2008; Hunt et al. 2014). The gas-phase metallicities can range from extremely low, like SBS 0335−052E and I Zw 18 with Z ≈ 0.03 Z⊙ (Izotov et al. 2005), to Z ≈ 0.3 Z⊙.
One feature that most BCDGs share is the presence of dust. Even the low-metallicity systems SBS 0335−052E and I Zw 18 show thermal dust emission, even when detectable CO emission is absent (Hunt et al. 2014). Another feature shared among the BCDGs is that they are gas-rich. All of the galaxies discussed in this paper have neutral hydrogen gas with masses similar to or exceeding their stellar mass. Their gas fractions range from 0.17 to 0.96 (Table 1). The H i data are mostly based on single-dish observations. When higher angular resolution observations are available, it is found that the H i usually extends beyond the stellar component of the galaxies. Despite several dedicated surveys, only a small number of BCDGs have a detectable molecular gas component (e.g., Sage et al. 1992; Barone et al. 2000; Meier et al. 2001; Bravo-Alfaro et al. 2004; Israel 2005; Beck et al. 2020). The molecular gas mass is typically just a few percent of the total H i mass. One exception is Haro 02, where .
In the reminder of this paper we will characterize the dust emission and the SFH of the 11 local analog candidates defined above, to see whether they can be viewed as scaled-down versions of high-redshift galaxies.
3. Data
In this section we describe the data reduction process we used to produce maps of our target galaxies and the methods to obtain photometric estimates.
In order to characterize the 11 nearby galaxies that make up our sample of potential local analogs, we compile broadband photometric data, covering UV to FIR. We supplement the existing UV–NIR photometric data from Brown et al. (2014) with FIR observations of five galaxies in our sample using the Stratospheric Observatory for Infrared Astronomy (SOFIA). We combined these with archived infrared data from Herschel, Spitzer, AKARI, and WISE. A listing of the infrared data sets used in our analysis, indicating the different telescope and instrument combinations, is given in Table 2. The galaxies discussed in this paper have a small angular extent. Both the Brown et al. (2014) photometric data and the FIR data used here (SOFIA, Herschel, AKARI, IRAS) fully cover all the emission of these galaxies. This allows us to measure the global flux at all bands, without missing emission regions or blending concerns. In Figure 4 we show an example of imaging of one of our galaxies, Mrk 1450. The images shown consist of our SOFIA-HAWC+ observations, along with archival data from Spitzer and SDSS g band (Gunn et al. 2006) and Herschel PACS and SPIRE instruments. Mrk 1450 is well detected in all bands but starts to fade in the SPIRE 350 μm band and is undetected in the SPIRE 500 μm band.
Table 2. Multiwavelength Data Set a
Galaxy | FIR Photometric Data (μm) | ||||||||||||||
---|---|---|---|---|---|---|---|---|---|---|---|---|---|---|---|
No | Name | 12–22 | 24–25 | 55 | 60 | 65 | 70 | 89–90 | 100 | 140–155 | 160 | 214 | 250 | 350 | 500 |
1 | NGC 2537 | W | M | S | I | ⋯ | M | S | I | S | M | ⋯ | ⋯ | ⋯ | ⋯ |
2 | Mrk 140 | W | M | ⋯ | I | ⋯ | H | ⋯ | H | ⋯ | H | ⋯ | H | H | H |
3 | Haro 02 | W | M-I | ⋯ | I | ⋯ | H | ⋯ | H | ⋯ | H | ⋯ | H | H | H |
4 | Mrk 1450 | W | M | S | ⋯ | ⋯ | H | S | H | S | H | S | H | H | H |
5 | UM 461 | IRS16—W | M | ⋯ | I | ⋯ | H | ⋯ | H | ⋯ | H | ⋯ | H | H | H |
6 | Mrk 1307 | W | M | S | I | ⋯ | M | S | I | S | M | ⋯ | ⋯ | ⋯ | ⋯ |
7 | Haro 06 | W | M | S | I | ⋯ | H | S | H | S | H | S | H | H | H |
8 | NGC 4670 | W | M | S | I | ⋯ | M | S | I | S | M | S | ⋯ | ⋯ | ⋯ |
9 | Mrk 475 | IRS16—W | M | ⋯ | ⋯ | ⋯ | M | ⋯ | ⋯ | ⋯ | M | ⋯ | ⋯ | ⋯ | ⋯ |
10 | UGCA 410 | IRS16 | M-I | ⋯ | I | A | ⋯ | A | ⋯ | ⋯ | A | ⋯ | ⋯ | ⋯ | ⋯ |
11 | Mrk 930 | W | M | ⋯ | ⋯ | ⋯ | H | ⋯ | H | ⋯ | H | ⋯ | H | H | H |
Note.
a W stands for WISE, IRS is for Infrared Spectrograph of Spitzer, M is for MIPS, S is for SOFIA, H is for Herschel, I is for IRAS, and A is for AKARI.Download table as: ASCIITypeset image
Figure 4. Optical to FIR images of Mrk 1450. The red bar corresponds to 20'', and the blue bar indicates 1 kpc.
Download figure:
Standard image High-resolution image3.1. SOFIA Observations
SOFIA (Young et al. 2012; Temi et al. 2014) is a partnership between NASA and the German Aerospace Center (DLR), consisting of an extensively modified Boeing 747SP aircraft carrying a 2.7 m (106-inch) reflecting telescope (with an effective diameter of 2.5 m, or 100 inches). We were granted ∼8 hr of observing time with SOFIA, on Cycle 6 under the program 06-0222 (PI: T. Wiklind). We observed five galaxies of our sample, using the High-resolution Airborne Wideband Camera-plus instrument (HAWC+; Vaillancourt et al. 2007; Dowell et al. 2010; Harper et al. 2018), covering all available filters to derive the FIR SED.
The HAWC+ instrument has five bands identified as A, B, C, D, and E. We observed in bands A, C, D, and E at 53, 89, 154, and 214 μm, which have beam sizes (FWHM) of 485, 7
80, 13
6, and 18
2 respectively. Band B was not available during Cycle 6. The galaxies Mrk 1450, NGC 4670, and Haro 06 were observed in all four bands available. Due to time constraints, Mrk 1307 and NGC 2537 were only observed at 53, 89, and 155 μm bands. The data were reduced to obtain the total intensity maps using the HAWC+ Data Reduction Pipeline v1.3.0 as detailed in Harper et al. (2018).
3.1.1. SOFIA/HAWC+Photometry
The HAWC+ data are presented in five levels: level 0 is raw data, level 1 is the demodulated (chop not subtracted) data, after instrument corrections (flat-fielded, dark subtracted, bad pixel removed) becomes level 2, level 3 is obtained after flux calibration and telluric correction, and the combined observations from level 3 produce the level 4 data. More details on the pipeline process can be found in the data handbooks (Gordon et al. 2018).
We started our analysis with level 4 maps in units of Jy pixel−1 and performed annular photometry using the HIPE environment. A circular aperture, centered on the source emission, gives the flux + background emission; an annular region, free of emission and centered on the inner aperture, provides an estimate of the background. To determine the photometric errors, we placed six circular apertures of the same radius as the inner source aperture, at equidistant, flux-free areas around the source. The average flux of the six apertures (Bgave) is then subtracted from the source flux:

As a further test, we estimated the integrated flux using Casa 3 and the built-in two-dimensional Gaussian procedure. This produced integrated fluxes very similar to the ones obtained using aperture photometry.
To determine the photometry errors, we used six photometry apertures around the source, taking care to avoid any other source in the background, and then uncertainty of the measurement was defined as the standard deviation of the background apertures. The total error is computed as the addition on quadrature of the uncertainty of the measurement Bgstd plus the absolute calibration error; the absolute calibration percentages are listed in Table 3.

Table 3. Fractional Calibration Error
Telescope | Instrument | Abs. Calibration |
---|---|---|
SOFIA | HAWC+ | 15% |
Herschel | PACS | 10% |
Herschel | SPIRE | 15% |
WISE | W1-W4 | 6% |
AKARI | N:60,160,WIDE:S,L | 10% |
Spitzer | IRS | 5% |
Spitzer | IRAC | 5% |
Spitzer | MIPS | 5% |
Download table as: ASCIITypeset image
The SOFIA/HAWC+ and photometry measure and uncertainties are shown in Table 4 (the full table is available in machine-readable form in the digital version of this paper).
Table 4. UV–FIR Photometric Data a
Galaxy | HAWC+A | MIPS | HAWC+C | PACS | HAWC+D | HAWC+E | SPIRE |
---|---|---|---|---|---|---|---|
Name | 55 μm | 60 μm | 89 μm | 100 μm | 155 μm | 214 μm | 250 μm |
NGC 2537 | 1302.4 ± 255.0 | 4040.0 ± 286.4 | 4893.3 ± 914.1 | ⋯ | 6301.9 ± 1085.0 | ⋯ | ⋯ |
Mrk 140 | ⋯ | ⋯ | ⋯ | 563.2 ± 44.8 | ⋯ | ⋯ | 172.0 ± 26.3 |
Haro 02 | ⋯ | ⋯ | ⋯ | 5051.3 ± 254.6 | ⋯ | ⋯ | 911.9 ± 137.2 |
Mrk 1450 | 274.8 ± 41.6 | ⋯ | 317.0 ± 47.9 | 272.0 ± 21.8 | 168.2 ± 27.4 | 52.5 ± 17.3 | 48.7 ± 8.4 |
UM 461 | ⋯ | ⋯ | ⋯ | 132.0 ± 12.7 | ⋯ | ⋯ | 23.1 ± 7.1 |
Mrk 1307 | 724.7 ± 119.9 | 1000.0 ± 71.6 | 1277.6 ± 198.6 | ⋯ | 616.5 ± 115.4 | ⋯ | ⋯ |
Haro 06 | 646.3 ± 97.0 | ⋯ | 849.4 ± 127.4 | 742.3 ± 39.2 | 754.3 ± 115.9 | 309.8 ± 48.7 | 197.3 ± 30.0 |
NGC 4670 | 1428.2 ± 246.1 | 3470.0 ± 245.7 | 3505.9 ± 530.7 | ⋯ | 3531.9 ± 713.4 | 2486.7 ± 428.9 | ⋯ |
Mrk 475 | ⋯ | 110.0 ± 16.0 | ⋯ | ⋯ | ⋯ | ⋯ | ⋯ |
UGCA 410 | ⋯ | ⋯ | ⋯ | ⋯ | ⋯ | ⋯ | ⋯ |
Mrk 930 | ⋯ | ⋯ | ⋯ | 1140.0 ± 91.9 | ⋯ | ⋯ | 210.4 ± 31.9 |
Note.
a This is an abbreviated version of the complete table of flux values used for the analysis. The complete table is available in machine-readable form in the digital version of this paper. The full table contains 49 columns, indicating the telescope and instrument/band for each measurement and the uncertainty. Fluxes are given in mJy.Only a portion of this table is shown here to demonstrate its form and content. A machine-readable version of the full table is available.
Download table as: DataTypeset image
3.2. Ancillary UV to MIR Data
The Brown et al. (2014) templates contain SEDs covering a wavelength range from UV to the MIR for 129 nearby galaxies. As described in Section 2, the SEDs of these templates have been normalized and verified with matched-aperture photometry. They provide accurate photometric data, and since our LAHz sample is drawn from these templates, we use the photometric data as presented in Brown et al. (2014).
3.3. Ancillary FIR Data
Some galaxies in our sample were observed with the Herschel Space Observatory, using PACS at 70, 100, and 160 μm, with a field of view (FOV) of , and SPIRE at 250, 350, 500 μm, with an FOV of
.
The Herschel Dwarf Galaxy survey (DGS, Madden et al. 2013; Rémy-Ruyer et al. 2013, 2015) provides photometric and spectroscopic FIR data on 50 dwarf galaxies. This survey includes data for some of the galaxies in our survey: Mrk 930, Mrk 1450, and UM 461, which were observed with all bands of both PACS and SPIRE instruments. A limited set of Herschel photometric data is available for UGCA 410 (also knowns as Mrk 140) and Haro 02 (Herschel Science 2013). See the full Table 4 for a complete listing of the FIR photometric data for our sample. For consistency in our flux estimates, we reduced the available Herschel PACS and SPIRE data using the Herschel pipeline for total intensity photometric measurements. We used the Herschel Interactive Processing Environment (HIPE; Ott 2010) with version 7 of the photometric calibration.
3.3.1. Herschel Photometry
We use level 2.5 or 3.0 maps for our photometry estimates. These maps are created from a combination of different observations of the same target and have improved S/N levels.
For more details about Herschel products, see the PACS Data Reduction Guide. 4
To determine the total intensity in Herschel PACS 70, 100, and 160 μm maps, we use the PACS—Photometry Script, which is part of the Herschel common science system (HCSS) provided in the HIPE environment. We started with the maps: Photometry Color High Pass Filter Map (HPPHPFMAPR) level 2.5 or 3.0, depending on availability. We modified parameters in the algorithm in order to use the optimal aperture radius. The final outputs of the algorithm are the peak flux in Jy pixel−1, the flux measured by the aperture photometry in Jy, and the aperture-corrected flux in Jy. SPIRE photometry maps can be in two different observation modes, point source (psrc) in units of Jy beam−1 or extended source (extsrc) in units of MJy sr−1, and are identified as PSW, PMW, and PLW, which stand for Photometry-Short/Medium/Long Wavelength, corresponding to 250, 350, and 500 μm, respectively. Here we used the psrcPSW, psrcPMW, and psrcPLW level 2.5 maps.
Similarly to PACS, for SPIRE photometry maps we use the SPIRE Photometry Point Source Script provided within HIPE. This uses the sourceExtractorSussextractor task to identify the source and applies the color correction for point sources. The Herschel pipeline assumes α = −1; we assumed α = 2.0.
4. Methodology
In this section we describe our modeling approach to characterize the dust continuum and the stellar emission. We use two different methods: modified blackbody, and the Lightning fitting package (Eufrasio et al. 2017). Both approaches allow for a determination of the FIR luminosity and dust mass for each galaxy. The modified blackbody models are used to also derive luminosity-weighted dust temperatures, while the Lightning method allows a determination of the SFH (Section 4.2).
4.1. Blackbody Modeling
The simplest characterization of dust emission is to fit it with a blackbody Fν
∝ Bν
(T). In a more realistic situation, this is modified by taking the transfer of radiation into account. This can be done by using a so-called modified blackbody curve, . We will use the expression

where Bν
(T) is the Planck function and Ω is the solid angle extended by the emission. The opacity is parameterized as , where λ0 is the wavelength where the optical depth equals unity (e.g., Draine 2006), and the coefficient β represents the opacity spectral index (Kovács et al. 2010). Observationally, β has been derived to be between 1 and 2 (see Hildebrand 1983; Casey 2012). Data from the Planck mission provide a robust derivation of β in the Milky Way (MW), leading to β = 1.8 ± 0.1 (Planck Collaboration et al. 2011). For extragalactic objects it is common to use β in the range 1.3–2.0. Here we will adopt the commonly used value β = 1.5 and keep it constant in our fits. We did try fitting with different β values, but we did not find that it changed our results in any significant way. We also kept the wavelength where the optical depth is unity constant at λ0 = 100 μm. The two parameters β and λ0 affect the flux on the long-wavelength Rayleigh–Jeans part of the dust SED relative to the short-wavelength part; for a fixed dust temperature, an increasing λ0 leads to a relative increase in the Rayleigh–Jeans part, while an increase in β leads to a decrease in the Rayleigh–Jeans part relative to the Wien part of the SED.
When fitting a modified blackbody curve to flux measurements at λ ≳ 60 μm, most galaxies exhibit an MIR excess at wavelengths shorter than 60 μm (e.g., Casey 2012). This excess can be attributed to the presence of a warmer dust component than the one characterizing the longer wavelengths, or an additional heating source, such as an AGN (see Scoville & Kwan 1976). The observed MIR emission can be modeled as a power law, with a cutoff wavelength λc (see Casey 2012), or as a second dust component characterized by a warmer dust temperature. Since we do not expect significant AGN activity in the dwarf galaxies making up our sample, we will adopt the latter case and use two modified blackbody curves, with the same β and λ0, but with different dust temperatures. Our dust SED to be fitted to the observed data is then
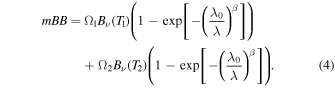
With the opacity spectral index kept fixed at β = 1.5 and the critical wavelength at λ0 = 100 μm, the modified blackbody curve contains four free parameters: two dust temperatures T1 and T2, and two normalization constants containing the solid angle of the source Ω. We use the Levenberg–Marquardt fitting algorithm. We explored the fitting using values for the dust opacity coefficient β ranging from 1.2 to 2.0 and for λ0 in the range 50–200 μm. We found no significant variation in the derived dust temperatures, and we conclude that a fixed β = 1.5 with λ0 = 100 μm is sufficient and that the models will be characterized by the temperatures and normalization coefficients.
We employ Bayesian statistics inference in multiparameter spaces to determine the most probable set of model parameters ϕ of a model mBBi in comparison to the data Di . Bayesian inference is based on Bayes's theorem, which establishes the conditional probability of the parameters ϕ given the data Di as

where the likelihood of the model is p(Di ∣ϕ). Then, p(ϕ∣Di ) is the posterior probability, p(ϕ) are the priors or initial knowledge of the parameters. p(Di ) is used to normalize the posterior probability. The likelihood is assumed to be

By maximizing the posterior probability, we will determine each ϕ parameter's best values for the modified blackbody models mBBi . Since we are assuming a fixed β = 1.5 and λ0 = 100 μm for both components, the ϕ parameters are the temperatures T1 and T2 and normalization constants Ω1 and Ω2. The priors are defined by allowing the ϕ parameters to vary through a very wide range initially, to get a posterior probability, and then Bayesian analysis allows us to update the knowledge of the probability distribution of the parameters and apply new priors multiplying by flat priors that reduce the range of the parameter space. This allows us to reduce the region covered by the parameter and obtain a better resolution of the parameter grid. Inspection of the marginalized probability distribution of each parameter space allows us to determine the appropriate range in which the best set of solutions are located, as well as the most likely value. We can also define the confidence interval for each parameter.
4.1.1. Dust Masses
The dust mass can be derived from the flux density of optically thin emission:

where Sν is the observed flux density, Bν (Td ) is the blackbody emission at a given temperature, D is the distance, and κν is the dust absorption coefficient for dust grains at frequency ν. The dust absorption coefficient is a parameter encompassing both the dust grain composition and the grain size distribution and is difficult to derive observationally (e.g., Gordon et al. 2014).
Different values of the ratio of visual extinction to reddening RV are used to define κν . RV = 3.1 is the standard value for the diffuse galactic ISM, also used for the Large Magellanic Cloud by Fitzpatrick (1986). Prevot et al. (1984) and Bouchet et al. (1985) found RV = 2.72 ± 0.21 for the Small Magellanic Cloud (SMC), and Calzetti et al. (2000) found RV = 4.05 ± 0.8 for starburst galaxies. More recently, Weingartner & Draine (2001) derived κν for different values of RV for MW dust and LMC and SMC extinction laws. For the dust masses presented in this paper, we use the updated MW extinction laws 5 κν presented in Weingartner & Draine (2001), and we calculate κν for the average of RV = 3.1, 4.0, and 5.5 at λ = 250 μm. This yields κ250 = 0.41 m2 kg−1. Using the MW, we may be underestimating the dust mass; using the SMC and LMC dust extinction laws produces κ250 = 0.52 m2 kg−1 and κ250 =0.50 m2 kg−1, which would yield dust masses ∼20% larger. We do not have observed flux values at λ = 250 μm for all of our galaxies. Instead, we use the flux density provided by our mBB fits to the overall dust SED. Hence, the dust mass, using the flux density at 250 μm, can be expressed as

The dust masses derived in this way are presented iin Section 5.1 and discussed in Section 5.3.
4.2. Lightning SED Fits
We performed SED fitting using Lightning (Eufrasio et al. 2017, ASCL:1711.009 6 ), which fits nonparametric SFHs in discrete, fixed-width, stellar age bins. We made use of the most recent update to Lightning, which uses an adaptive Markov Chain Monte Carlo (MCMC) algorithm (Doore et al. 2021). For this work we have used SFH bins of 0−10 Myr, 10−100 Myr, 100 Myr−1 Gyr, 1−5 Gyr, and 5−13.3 Gyr and assumed a Kroupa initial mass function (Kroupa 2001) and a metallicity of 0.2 Z⊙. We used PEGASE (Fioc & Rocca-Volmerange 1997, 1999) stellar populations. For intrinsic attenuation, we adopted a Calzetti et al. (2000) extinction law, modified as in Noll et al. (2009) to include a UV bump at 2175 Å and a parameter δ to control the slope of the extinction curve. For instance, δ = 0 produces the Calzetti curve, a lower δ corresponds to a higher slope of the extinction curve, and a higher δ corresponds to a flatter extinction curve. Draine & Li (2007) MW dust emission was used. For a more thorough description of the prescriptions adopted by Lightning, we refer the reader to Eufrasio et al. (2017).
The IR photometry previously described described was combined with literature SEDs from Brown et al. (2014), producing an SED with 45 data points from the FUV to the FIR (including GALEX, Swift UVOT, SDSS, 2MASS, WISE, Spitzer, SOFIA, IRAS, AKARI, and Herschel). Our fits explore a 13-dimensional parameter space, with 5 parameters regarding the SFH intensities ψi
, 3 parameters , δ, and
) controlling the dust extinction, and 5 parameters controlling dust emission (α, Umin, Umax, γ, and qPAH).
The range allowed for each parameter is shown in Table 5. This range of δ allows the dust extinction curve to be steeper or flatter relative to the Calzetti extinction curve (for more details, see Eufrasio et al. 2017). Also, the five dust emission parameters explore the full space of the Draine & Li (2007) models. An illustration of how these parameters affect the SED is shown in Leja et al. (2017). We run the adaptive MCMC algorithm for 3 × 105 steps and derive statistics from the last 5000 steps of the chain. All the derived quantities quoted here (SFHs, SFRs, M⋆, Mdust) are derived from these last 5000 steps.
5. Results
We derive properties of the dust in our LAHz galaxies using two different methods. Some of the derived parameters are duplicated in the two methods, such as the FIR luminosity and dust mass. Others are unique for each method. For instance, the modified blackbody models provide luminosity-weighted dust temperatures, which the Lightning models do not. The Lightning models provide an estimate of the SFR integrated over the last 100 Myr and a parameterized SFH. The derived parameters from the modified blackbody models are presented in Table 6, and those from the Lightning models are presented in Table 7.
Table 5. Parameter Range
Parameter | Initial | Final |
---|---|---|
ψi | ≳0.0 | ⋯ |
![]() | 0.0 | 3.0 |
δ | −2.3 | 0.4 |
![]() | 0.0 | 4.0 |
α | 0.0 | 4.0 |
Umin | 0.1 | 25 |
Umax | 103 | 3 × 105 |
γ | 0.0 | 1 |
qPAH | 0.0047 | 0.0458 |
Download table as: ASCIITypeset image
Table 6. Modified Blackbody Derived Quantities
No | Galaxy | Td Warm | Td Cold | S24 μm/S160 μm a | Md | LFIR |
---|---|---|---|---|---|---|
Name | (K) | (K) | (M⊙) | (109 L⊙) | ||
1 | NGC 2537 |
![]() |
![]() | 0.06 |
![]() | 0.638 ± 0.028 |
2 | Mrk 140 |
![]() |
![]() | 0.10 |
![]() | 0.784 ± 0.043 |
3 | Haro 02 |
![]() |
![]() | 0.29 |
![]() | 6.222 ± 0.172 |
4 | Mrk 1450 |
![]() |
![]() | 0.41 |
![]() | 0.150 ± 0.008 |
5 | UM 461 |
![]() |
![]() | 0.42 |
![]() | 0.161 ± 0.009 |
6 | Mrk 1307 |
![]() |
![]() | 0.23 |
![]() | 0.913 ± 0.038 |
7 | Haro 06 |
![]() |
![]() | 0.14 |
![]() | 1.809 ± 0.092 |
8 | NGC 4670 |
![]() |
![]() | 0.08 |
![]() | 2.665 ± 0.109 |
9 | Mrk 475 |
![]() |
![]() | 0.24 |
![]() | 0.024 ± 0.003 |
10 | UGCA 410 |
![]() |
![]() | 0.71 |
![]() | 0.103 ± 0.006 |
11 | Mrk 930 |
![]() |
![]() | 0.29 |
![]() | 16.225 ± 1.313 |
Note.
a The flux ratio S24 μm/S160 μm is derived from the modified blackbody best-fit model.Download table as: ASCIITypeset image
Table 7. Lightning-derived Quantities
No. | Galaxy | Mdust | LFIR | M* | SFR a | sSFR |
---|---|---|---|---|---|---|
Name | (104 M⊙) | (109 L⊙) | (108 M⊙) | (M⊙ yr−1) | (Gyr−1) | |
1 | NGC 2537 | 9.5 ± 3.0 |
![]() |
![]() |
![]() | 0.19 ± 0.04 |
2 | Mrk 140 | 25.7 ± 7.2 |
![]() |
![]() |
![]() | 0.46 ± 0.14 |
3 | Haro 02 | 21.5 ± 4.8 |
![]() |
![]() |
![]() | 0.80 ± 0.23 |
4 | Mrk 1450 | 1.9 ± 0.2 |
![]() |
![]() |
![]() | 1.60 ± 0.44 |
5 | UM 461 | 1.3 ± 0.3 |
![]() |
![]() |
![]() | 1.17 ± 0.50 |
6 | Mrk 1307 | 11.0 ± 2.9 |
![]() |
![]() |
![]() | 2.51 ± 0.55 |
7 | Haro 06 | 81.8 ± 5.8 |
![]() |
![]() |
![]() | 1.83 ± 0.40 |
8 | NGC 4670 | 207.0 ± 66.0 |
![]() |
![]() |
![]() | 0.08 ± 0.03 |
9 | Mrk 475 | 0.17 ± 0.04 |
![]() |
![]() |
![]() | 0.39 ± 0.20 |
10 | UGCA 410 | 2.6 ± 1.2 |
![]() |
![]() |
![]() | 2.21 ± 0.64 |
11 | Mrk 930 | 68.7 ± 19.5 |
![]() |
![]() |
![]() | 4.71 ± 0.87 |
Note.
a The SFR averaged over the last 100 Myr.Download table as: ASCIITypeset image
5.1. Modified Blackbody Models
The blackbody modeling described in Section 4.1 shows that a reasonable description of the dust SED can be achieved by using a two-component modified blackbody fit. The warm component is less well constrained than the cold component owing to a smaller number of photometric data points at wavelengths λ ≲ 60 μm. Nevertheless, the model SED joins smoothly with the NIR and MIR fluxes of the Brown et al. (2014) templates.
In Figure 5 we show multiparameter-space diagnostic plots for two galaxies in our sample: Haro 06 and Mrk 1450. We can see the marginalized distribution for each ϕ parameter in the upper frame of each column; the lower frames show the marginalized probability in blue and the correlation values between the two parameters. The value that maximizes the χ2 distribution (likelihood) for each parameter is indicated with a red star; this is also shown as a red dashed line in the upper frames for each parameter. The confidence interval is shown in light red and indicates the 16th and 84th percentiles in the probability distribution, which imply that 68% of the probability is inside that region.
Figure 5. Corner plots for the modified blackbody models of the SED of Haro 06 and Mrk 1450. In each panel, in the bottom, the 2D scatter plots (blue area) of the values recovered from the four parameters grid are shown for the two-by-two combination of parameters (two temperatures and two normalization constants). The top frame of each column are the the 1D marginalized probability distribution for each parameter. Top right: best-fit model in black, along with the two dust components, cold component in yellow and warm in light red. The red are the SOFIA data points, and blue data points are the ancillary data available. The green solid line shows the Brown et al. (2014) spectral data, which are not part of the fits; however, they are in good agreement with the modified blackbody model.
Download figure:
Standard image High-resolution imageThis visualization of the probability distributions allows us to understand how the different parameters relate. For instance, we can see how the temperature T1 and the constant Ω1 are anticorrelated, while the two constants Ω1 and Ω2 are positively correlated for both galaxies. In the upper right box we see the best-fit model indicating the two blackbody components representing a warm and a cold dust component. The corresponding Brown et al. (2014) template is shown as a reference, but it is not part of the fit. The resulting best-fit model agrees smoothly with the Brown templates.
In Figure 6 we present the SEDs produced by the two-component blackbody models for all of our LAHz galaxies. In a few cases the warm and the cold components form two humps or peaks in the SED, often found for star-forming galaxies. However, in several cases, the warm and cold components blend together and form a broad single-peaked SED. In the figures we also include the corresponding NIR and MIR spectra from Brown et al. (2014). These spectra were not part of the fit, but they merge smoothly with the dust SED. In three cases (NGC 2537, Haro 02, and NGC 4670), the MIR spectra suggest that there might be additional flux at wavelengths between 24 and 70 μm, compared to what is indicated in the two-component blackbody fits.
Figure 6. Blackbody best fits: LAHz dust emission. The best modified blackbody fit is shown with the black solid line; the light-green and light-red dashed lines are the warm and cold components, respectively. The fits only include wavelengths ≥12 μm. The SOFIA bands are shown in red. Ancillary data are shown in blue: WISE, Herschel, Spitzer, AKARI, and IRAS. All data used are specified in Table 2. The Brown et al. (2014) templates are plotted as a reference (green solid lines) but are not part of the blackbody fits.
Download figure:
Standard image High-resolution image5.1.1. Dust Temperatures
We obtained the dust temperatures characterizing the warm and cold components from the modified blackbody fits. For comparison, we use the DGS presented by Rémy-Ruyer et al. (2013), which includes local dwarf galaxies, and in order to provide a comparison with more evolved galaxies, we use a subset of 58 galaxies from the Key Insights on Nearby Galaxies: a Far-Infrared Survey with Herschel (KINGFISH; Kennicutt et al. 2011), where we exclude the irregular dwarf galaxies and early-type galaxies from the original set of 109 galaxies.
In order to compare our results with the galaxies in the DGS and KINGFISH samples, we reanalyzed the FIR photometric data from these surveys using the same modified blackbody method as for our LAHz galaxies. For each galaxy we derive the temperatures characterizing the warm and cold components, as well as the temperature corresponding to the peak of the combined SED, defined as

where b is Wien's displacement constant. All the galaxies were characterized using two dust components. The average dust temperatures for the sample of LAHz are Td,warm ∼ 124 K and Td,cold ∼ 42 K. This is significantly higher than for star-forming disk galaxies in the KINGFISH sample, with Td,warm ∼ 68 K and Td,cold ∼ 23, and higher than for the DGS sample, with Td,warm ∼ 74 K and Td,cold ∼ 24. The range of temperatures for the DGS survey for the cold component is, however, quite large, ranging from 18 to 41 K (Rémy-Ruyer et al. 2013). The results are shown in Figure 9.
5.2. Lightning Models
As described in Section 4.2, the Lightning models fit the whole UV–FIR SED, while self-consistently applying an extinction correction. The FIR is modeled using Draine & Li (2007) templates. The resulting SEDs for our LAHz galaxies are shown in Figure 7. The black data points represent the observed SED; the blue line is the spectra without dust attenuation; the red line is the SED with attenuation; the orange line is the best-fit dust emission. In Table 7 we show luminosities, dust masses, SFR averaged over the last 100 Myr, and sSFRs derived from the Lightning models.
Figure 7. FUV-to-FIR SEDs produced with the Lightning fitting package. Intrinsic stellar spectra are shown in blue. Attenuated stellar spectra are shown in red. Dust emission spectra are shown in orange. The observed data are shown as black diamonds. Red diamonds are the model SEDs, i.e., the attenuated stellar spectra plus the dust emission spectra convolved with the filter functions. When no observations are available in a band, models are still computed.
Download figure:
Standard image High-resolution image5.3. Far-infrared Luminosity and Dust Mass
We derive dust masses and LIR using the SEDs produced with the two different modeling methods described in Section 4. The dust masses and LIR from the mBB models and the Lightning method are presented in Tables 6 and 7, respectively. The LIR is derived by integrating the SED from 8 to 1000 μm. The LAHz galaxy luminosities cover a wide range of for the blackbody models; similarly, the Lightning method luminosities range between
.
The derived dust masses using the blackbody models are in the range of . As expected, the cold dust component dominates the total dust mass. We also derive the dust mass from the Draine & Li best fits in the Lightning models:
. Some of the galaxies in our sample have published dust mass values, derived using a subset of the photometric data used here (e.g., Engelbracht et al. 2008; Rémy-Ruyer et al. 2013), which agree very well with the results presented here. For the discussion presented in the rest of this paper, we use the dust masses derived with the mBB method, since this allows the comparison with dust masses derived for survey samples like KINGFISH and DGS using the same methodology.
We compare the dust masses and FIR luminosities for our LAHz galaxies, derived with the modified blackbody and Lightning methods. The latter method uses the Draine & Li (2007) templates to model the FIR SED. The dust masses derived from the mBB and the Draine & Li models show dispersion of ∼0.3 dex but show no systematic difference. The FIR luminosities, however, do show a systematic offset, where the Draine & Li (2007) based models give ∼20% higher LFIR than those derived by integrating over the modified blackbody models. This is expected since the Draine & Li (2007) models include PAH emission in the NIR and better account for the NIR to MIR emission than the blackbody models. In the rest of the paper we will use the mBB-derived dust masses for comparison with the DGS and KINGFISH surveys, as well as the Lightning FIR luminosities for discussions and conclusions.
5.4. Star Formation Histories
In Figure 8 we show the SFH for the LAHz galaxies in our sample. For comparison, we also show the SFH of M51 from Eufrasio et al. (2017). The SFH was derived using the Lightning fitting package, and it is presented in five look-back time bins: 0−10 Myr, 10–100 Myr, 0.1–1 Gyr, 1–5 Gyr, and 5–13.3 Gyr. The Lightning modeling treats the SFR as constant over each time bin. The solid red lines denote the median SFR for each time bin from the simulations, and the yellow region denotes the 16th and 84th percentiles, enclosing the 68% confidence intervals. The SFHs of the LAHz are for the most part very different from those of more massive galaxies. For instance, the SFH of M51, a large spiral galaxy gravitationally interacting with a neighbor galaxy, shows a more complex SFH, with a significant star formation contribution in bins corresponding to ages older than 5 Gyr.
Figure 8. SFHs for our sample. The SFHs are divided into five time bins: 0–10 Myr, 10–100 Myr, 100 Myr–1 Gyr, 1–5 Gyr, and 5–13.3 Gyr. The solid lines denote the median values from the MCMC chain, and dotted lines show the 16th and 84th percentiles, enclosing the hashed areas. For comparison, the last frame includes the SFH of M51, derived by Eufrasio et al. (2017), following a similar approach.
Download figure:
Standard image High-resolution imageThe SFH temporal resolution is nonlinear, with longer timescales for higher look-back times. Even a very low SFR in the older bins can significantly contribute to the total stellar mass. However, for six galaxies we recover SFH solutions compatible with null stellar masses older than 1 Gyr: Mrk 1450, UM 461, Mrk 1307, Mrk 475, UGCA 410, and Mrk 930. These galaxies do not present statistically significant evidence of a stellar population older than 1 Gyr. This suggests that they are the youngest galaxies in our sample. Two of the galaxies, NGC 2537 and NGC 4670, show star formation activity in all five time bins, showing a statistically significant presence of an old stellar population. Hence, despite being designated as BCDGs, the stellar masses of these two galaxies are dominated by old stars. The situation is less clear for three of the galaxies in our sample, Mrk 140, Haro 02, and Haro 06, where the Lightning results indicate that it is probable that they had a low level of star formation activity at times ≳1 Gyr (see Figure 8).
We consider the six LAHz galaxies that have SFHs consistent with being younger than ∼1 Gyr as our prime candidates for being local analogs. NGC 2537 and NGC 4670 are clearly not young galaxies. Mrk 140, Haro 02, and Haro 06 are likely to have an old stellar population, although Haro 06 is a borderline candidate for being a local analog.
6. Discussion
6.1. Characteristic Dust Temperatures
When fitting a two-component modified blackbody curve to our LAHz galaxies, the DGS, and spiral galaxies in the KINGFISH sample, we find that the DGS and LAHz galaxies show a higher Tpeak than the more massive KINGFISH galaxies (Figure 9). While the average Tpeak is 33 and 35 K for the LAHz and DGS samples, respectively, the KINGFISH galaxies have an average of Tpeak = 23 K. When we compare the warm/cold dust component separately, we see that the LAHz galaxies are characterized by higher dust temperatures than both the KINGFISH and DGS galaxies. These results are consistent with a general trend of warmer dust in metal-poor galaxies compared with the more metal-rich and more massive counterparts (e.g., Rémy-Ruyer et al. 2013), as well as for high-z galaxies (e.g., Faisst et al. 2017; Sommovigo et al. 2020).
Figure 9. Comparison of peak, warm, and cold temperatures characterizing the dust. Solid lines indicate average temperatures; the circles are the individual values for each galaxy. KINGFISH galaxies are shown in blue, DGS in green, and the LAHz candidates in red. All temperatures were determined using the modified blackbody procedure (see Section 4.1).
Download figure:
Standard image High-resolution image6.2. Dust-to-stellar Mass Ratio
In Figure 10 (top left panel), we show the dust-to-stellar mass ratio as a function of stellar mass for our sample, together with those of the DGS and KINGFISH spiral galaxies. While the KINGFISH galaxies seem to have a weak dependence on stellar mass, no such correlation is apparent for our LAHz galaxies or for galaxies in the DGS sample. In fact, the dust-to-stellar mass ratio for the LAHz galaxies seems to be approximately constant, with a median ratio /M⋆) = (8.5 ± 5) × 10−4. A similar argument can be made for the DGS sample, but with a slightly lower average dust-to-stellar mass ratio. The dashed line shown in Figure 10 shows the anticorrelation between dust-to-stellar mass ratio and stellar mass found by Clemens et al. (2013). This anticorrelation was obtained for galaxies from the Planck Survey, and the fit is dominated by galaxies in the stellar mass range
. The anticorrelation is surprising in view of the fact that the gas-phase metallicity scales with stellar mass (e.g., Tremonti et al. 2004) and one would expect a lower Mdust/M⋆ ratio for low-mass galaxies. The Clemens et al. (2013) relation has been interpreted as being due to a rapidly increasing gas fraction for lower-mass galaxies, more than offsetting the metallicity effect. However, the results given here, for both our sample and that of DGS, show that this relation cannot be extended to low-mass galaxies.
Figure 10. Top left: dust-to-stellar mass ratio as a function of stellar mass. The magenta dashed line denotes the relation from Clemens et al. (2013), based on more massive galaxies. Top right: dust-to-stellar mass ratio as a function of metallicity. The power law defined by Rémy-Ruyer et al. (2013) is shown as a dashed line. For comparison, we include the extremely low metallicity galaxies SBS 0335−052 and I Zw 18 (purple circles; e.g., Hunt et al. 2014). Bottom right: LIR/Mdust as a function of metallicity. The dashed line corresponds to the relation found by Rémy-Ruyer et al. (2013). We include SBS 0335−052 and I Zw 18 as purple circles. The histograms on the right show the distribution of each group. In all three panels, red squares are the LAHz candidates, green circles are the DGS sample, and blue circles represent the KINGFISH spiral sample.
Download figure:
Standard image High-resolution imageThe mass–metallicity relation is established down to . Below this stellar mass, depletion of metals through galactic winds starts to become important (e.g., Tremonti et al. 2004). Our LAHz galaxies have
in the range 7.0–9.4, straddling the
value. In Figure 10, top right panel, we show the dust-to-stellar mass ratio as a function of metallicity. In the figure we also include the DGS and KINGFISH spiral samples. Based on the latter two samples, Rémy-Ruyer et al. (2013) defined a correlation between the dust-to-stellar mass ratio and gas-phase metallicity, shown as a dashed line in Figure 10. We find no obvious correlation between the dust-to-stellar mass ratio and metallicity for our LAHz sample, and the overall dispersion of dust-to-stellar mass ratios is large. As discussed in Rémy-Ruyer et al. (2013), this could at least in part be due to observational uncertainties. However, for the metal-poor and low-mass galaxies, this could also be due to the impact of individual SFHs, where the interstellar gas can be promptly enriched through recent star formation or depleted owing to galactic winds. Evidence for both effects has been seen in these types of galaxies; Lagos et al. (2018) found spatial variations in the gas-phase metallicity in UM 461, attributed to recent infall of low-metallicity gas, and Haro 02 shows clear evidence of a galactic gas outflow (e.g., Meier et al. 2001; Beck et al. 2020).
For comparison, we also include two of the lowest-metallicity galaxies known, SBS 0335−052E and I Zw 18, in Figure 10. Both of these galaxies have very low gas-phase metallicity, 12 + logO/H ∼ 7.2, but even at these extremely low metallicities they still show dust continuum emission (SBS 0335−052E, Hunt et al. 2014; I Zw 18, Wu et al. 2007). These two particularly low-metallicity galaxies were studied by Hunt et al. (2014) and found to have dust-to-stellar mass ratios within the range of values found for the galaxies presented in Figure 10. The dust-to-stellar mass ratio of I Zw 18 is , which is consistent with the LAHz average of
. SBS 0335−052E, however, has
, which is significantly higher than the LAHz and DGS galaxies, comparable to metal-rich spiral galaxies. While this wide disparity among the most metal-poor galaxies could be due to observational uncertainties, it could also be an effect of the above-mentioned prompt enrichment and galactic outflow/inflow processes affecting low-mass galaxies.
The bottom right panel of Figure 10 shows the LIR/Mdust ratio as a function of metallicity for the LAHz galaxies (red squares). For comparison, we also show the DGS and KINGFISH galaxies. The distribution of LIR/Mdust is indicated on the side for each group. The LIR/Mdust of the LAHz galaxies is distributed in the same range as the DGS (Rémy-Ruyer et al. 2013). This ratio tends to increase for lower metallicities, and when we examine the left region in the range of metallicities, the LAHz galaxies exhibit a higher LIR/Mdust ratio than the DGS or KINGFISH galaxies for the corresponding metallicity. It is not possible with the present data to determine whether this is due to different grain properties or is simply an effect of the higher dust temperatures characterizing the dust emission in these galaxies. As shown in Figure 10, this trend extends to the extremely metal-poor galaxies SBS 0335−052E and I Zw 18, covering more than an order of magnitude in both metallicity and LIR/Mdust. These two galaxies are dominated by warm dust; their single-component dust SEDs are characterized by Tdust = 59 and 67 K, respectively (Hunt et al. 2014). While these temperatures are high, they are within the range of the temperatures characterizing our LAHz galaxies.
6.3. Star Formation versus AGN
The high temperatures characterizing the warm dust component of the LAHz galaxies, as well as the high MIR fluxes relative to the peak FIR flux of several of the LAHz galaxies, could potentially be due to the presence of a nonstellar heating source, such as AGNs. AGNs in low-mass galaxies are more difficult to detect than their counterparts in massive galaxies. None of our LAHz galaxies are, however, believed to harbor an active AGN. Reines et al. (2020) used the Very Large Array to show that previously identified radio sources that could potentially be AGNs in Haro 02 and Mrk 1307 are most likely associated with star formation activity.
We explore the possibility of AGN, LINER, or Seyfert activity in the LAHz galaxies by plotting them in a BPT diagram (Baldwin et al. 1981). This diagnostic uses the Hα/[Nii] ratio together with the [O iii] λ5007/Hβ ratio to distinguish between star formation and AGN activity. The spectral line observations presented in Moustakas & Kennicutt (2006) include all of our LAHz galaxies, and the BPT diagram of the galaxies in Moustakas & Kennicutt (2006) is shown in Figure 11, with our LAHz galaxies marked as red circles. All LAHz galaxies, except Mrk 140, fall in the star formation part of the diagram. Mrk 140 falls close to the line depicting maximum star formation activity as defined by Kewley et al. (2001). Since there are no other indications suggesting that Mrk 140 harbors an AGN, we will treat it as a pure star-forming system.
Figure 11. BPT diagram (Baldwin et al. 1981) for nearby galaxies in the Moustakas & Kennicutt (2006) sample. This sample contains all 11 of our local analog candidates, marked as red circles. The blue line depicts the "maximum starburst line" (Kewley et al. 2001) and is an upper limit for excitation caused by star formation. The red line is an alternate definition based on SDSS galaxies (Kauffmann et al. 2003). The green line divides Seyfert galaxies and LINERs (Cid Fernandes et al. 2010).
Download figure:
Standard image High-resolution image6.4. Star Formation Rates
In Figure 12 we show the correlations between Mdust/SFR and Mdust/M⋆ as a function of the sSFR (sSFR = SFR/M⋆). As shown in da Cunha et al. (2010), the dust-to-stellar mass ratio strongly correlates with the sSFR, confirming that dust mass and star formation are tightly related. In Figure 12 we color-code the stellar mass of each galaxy. More massive galaxies exhibit a lower sSFR and higher Mdust/SFR ratio, contrary to the lower-mass galaxies, which show higher sSFRs, tracing a clear decreasing relation between and sSFR. In the right panel we plot Mdust/M⋆ ratio versus sSFR. Here we see an increasing trend, where Mdust/M⋆ ratio increases as sSFR increases. Interestingly, the less massive galaxies seem to follow a parallel path to the more massive ones. In both cases, the LAHz candidates fall in the right side, showing that they have higher sSFR than more massive and dusty galaxies.
Figure 12. Left: the ratio of dust mass and SFR vs. sSFR. Right: the ratio of dust and stellar mass vs. sSFR. In both frames, the LAHz candidates are shown as squares. For reference we include the KINGFISH galaxies as circles (Kennicutt et al. 2011) and the DGS galaxies as triangles (Rémy-Ruyer et al. 2013). The two extremely low metallicity galaxies I Zw 18 and SBS 0335−052 (Hunt et al. 2014) are shown as diamonds. The color indicates stellar mass as shown in the right bar. More massive galaxies are red; less massive ones are blue.
Download figure:
Standard image High-resolution image7. Summary and Conclusions
The 11 galaxies designated as potential LAHz galaxies were initially selected based on the frequency with which they provide the best fits to the SED of high-z galaxies. They were drawn from the Brown et al. (2014) sample of local galaxy templates, which, although extensive in terms of number of galaxies, only provide a limited set of dwarf irregular galaxies. It is highly likely that there are other local galaxies with similar properties that could fit into the role as LAHz candidates; they just were not part of the Brown sample.
As a group, these 11 LAHz galaxies are similar to galaxies in the more diverse DGS (Madden et al. 2013). The LAHz galaxies tend to have higher sSFRs, have larger Mdust/M⋆ ratios, and be characterized by warmer dust temperatures than the average for the DGS sample. Despite the small sample size of LAHz candidates, we found that they have different properties. The SFHs for 6 of the 11 LAHz galaxies are consistent with zero star formation activity at times ≳1 Gyr, making them ideal candidates for comparison with the young galaxies in the high-z universe. This subset of LAHz consists of Mrk 1450, UM 461, Mrk 1307, Mrk 475, UGCA 410, and Mrk 930, and we will refer to it as the "gold" sample. Two of the LAHz galaxies, NGC 2537 and NGC 4670, have SFHs showing significant star formation activity over a Hubble time. These two galaxies, despite their appearance as BCDGs, are therefore dominated by an old stellar population and hence cannot be representative for high-z galaxies. For the benefit of this discussion, we will refer to these two galaxies as the "bronze" sample. The remaining three LAHz galaxies, Haro 02, Haro 06, and Mrk 140, have SFHs that cannot rule out star formation activity on longer timescales ≳5 Gyr. Hence, they fall in between the "gold" and "bronze" samples, and so we will designate them as the "silver" sample.
While we are dealing with small number statistics, there are several notable differences between the "gold" and "bronze" galaxies: The average sSFR of the "gold" sample is ∼15 times higher than the average of the two "bronze" galaxies. The average temperature of the cold dust component of the "gold" sample is 48 K, while both the "silver" and "bronze" galaxies have an average Tcold of 35 K. These differences could be due to the stochastic nature of star formation activity in these small galaxies. However, it is also accompanied by a systematic difference in gas-phase metallicity: the "gold" sample contains all the most metal-poor systems, 0.19 Z⊙, while the "silver" and "bronze" samples have an average metallicity of 0.40 Z⊙. The interstellar gas fractions are 0.68, 0.46, and 0.25 for the "gold," "silver," and "bronze" samples, respectively. Another parameter setting the "gold" galaxies apart is the flux ratio S24/S160, describing the strength of the MIR relative to the FIR. These ratios are listed in Table 6. The "gold" sample has an average ratio ∼0.4, compared with 0.07 for NGC 2537 and NGC 4670.
In Figure 13 we plot the SFR versus stellar mass for our LAHz galaxies and several other surveys, including the DGS and KINGFISH samples, the GOALS sample of ultraluminous infrared galaxies (Armus et al. 2009), and the Jarrett et al. (2019) sample of the 100 largest nearby galaxies. In addition, we plot high-redshift galaxies with 1 < z < 4.5 from the CANDELS survey (Santini et al. 2015). Diagonal dashed lines show different sSFR values, and the thick diagonal lines show the MS for redshifts z = 0, 1.5, and 4 (Schreiber et al. 2015). Our LAHz galaxies are shown as squares, with colors corresponding to whether they belong in the "'gold" (green), "silver" (red), or "bronze" (orange) samples. Most of the DGS, KINGFISH, and Jarrett et al. (2019) galaxies are centered around the MS for z = 0, including the turnoff at high stellar masses. The GOALS galaxies have, not surprisingly, a higher sSFR. The "gold" LAHz galaxies have high sSFR and are located above the z = 0 MS. The two "bronze" LAHz galaxies (NGC 2537 and NGC 4670) are located below the z = 0 MS, and the three "silver" LAHz galaxies are on the z = 0 MS. Hence, the six LAHz galaxies in the "gold" sample can be viewed either as z = 0 starburst systems or as MS galaxies at z ∼ 1.5–4.
Figure 13. Comparison SFR vs. stellar mass (M⋆). The MS is shown with the thick light-purple solid line for z = 0, purple for z = 1.5, and dark purple for z = 4. As a comparison, we show the CANDELS galaxies in gray, the KINGFISH galaxies in orange, the DGS in cyan, the GOALS in green, and the Jarrett et al. (2019) galaxies in light green. The sample of LAHz galaxies (this study) are shown as black squares, filled in green whenever they follow the MS for any z ≳ 1.5, in yellow whenever they follow the MS for any z ≳ 0, and in red when they follow below the MS for z = 0.
Download figure:
Standard image High-resolution imageBased on these findings, we propose that the six galaxies in the "gold" sample are good candidates for follow-up studies, especially determining their gasdynamics. If they truly are young systems, their age puts their formation epoch at z ∼ 0.08, or a luminosity distance of only ∼350 Mpc. This short timescale means that gravitational interaction and merging are not likely to be driving the star formation activity.
Based on observations made with the NASA/DLR Stratospheric Observatory for Infrared Astronomy (SOFIA). SOFIA is jointly operated by the Universities Space Research Association, Inc. (USRA), under NASA contract NNA17BF53C, and the Deutsches SOFIA Institut (DSI) under DLR contract 50 OK 0901 to the University of Stuttgart. Financial support for this work was provided by NASA through award No. 06-0222 issued by USRA. We extend our thanks to the SOFIA science support team, for all the help in the data acquisition and the data analysis process. Also, we thank the referee for constructive comments that helped improve this paper.
Software: HIPE (v7; Ott 2010), HAWC+ Data Reduction Pipeline (v1.3.0; Harper et al. 2018), Lightning (Eufrasio et al. 2017), MAGPHYS (da Cunha et al. 2008), Matplotlib (Hunter 2007), NumPy (van der Walt et al. 2011), SciPy (Virtanen et al. 2020).
Footnotes
- 3
- 4
- 5
Updated values https://www.astro.princeton.edu/~draine/dust/dustmix.html.
- 6