Abstract
We explore the influence of hot background temperatures in stellar clusters on the formation and evolution of photoevaporating disks. The disk forms from the gravitational collapse of a pre-stellar core. For a core with a relatively high temperature (>40 K), the angular momentum of the core is expected to be low. In the core-collapse stage, most of core mass directly falls onto the central star or the disk near the star. External photoevaporation is ineffective in this environment. The viscosity in the disk dominates its evolution, which leads to a high efficiency of the mass and angular momentum transports. The disk properties are determined by the core properties. In the vicinity of massive stars with strong external FUV fields, the disk can still survive when the background temperature is high (∼100 K). We suggest that the diversity of the molecular cloud core properties may lead to the diverse properties of the disk photoevaporation in clusters. We also consistently interpret the findings in NGC 1333 that low-mass disks (0.002–0.004 M☉) can exist in such young clusters (1–2 × 106 yr) with mild external photoevaporation.
Export citation and abstract BibTeX RIS
1. Introduction
Protoplanetary disks embedded in stellar clusters (e.g., Sicilia-Aguilar et al. 2013; Ansdell et al. 2017; Barenfeld et al. 2017; Champion et al. 2017) are remarkably different from those in regions with loose aggregates of young stars, such as Taurus-Auriga, Ophiuchus, Lupus, and Chamaeleon (e.g., Andrews & Williams 2005; Andrews et al. 2009; Ansdell et al. 2016; Pascucci et al. 2016). The disk evolution in stellar clusters is affected by two environmental mechanisms: stellar encounters and photoevaporation (e.g., Johnstone et al. 1998; Bonnell et al. 2003; Mitchell & Stewart 2010; Anderson et al. 2013; Kalyaan et al. 2015). Stellar encounters may truncate the disk and steepen the surface density profile (e.g., Rosotti et al. 2014; Vincke et al. 2015). External photoevaporation may sweep out the disk materials from the outside in (e.g., Johnstone et al. 1998; Adams et al. 2004). These two environmental mechanisms limit both the lifetime and size of disks embedded in stellar clusters (e.g., Adams 2010; Guarcello et al. 2016).
The probability of having a close encounter is related to the stellar density in clusters. Adams et al. (2006) showed that in small clusters with a few hundred members, such as NGC 1333, the close encounter rarely occurred. The smoothed particle hydrodynamics/N-body simulations performed by Rosotti et al. (2014) found that stellar encounters became important when stellar densities exceeded ∼2–3 × 103 pc−2. The studies of Cygnus OB2 have shown that close stellar encounters were rare even though stellar densities were as high as 102 pc−3 (Guarcello et al. 2016). Recently, Winter et al. (2018) investigated the disk truncation in stellar clusters by external photoevaporation and tidal encounters. Their calculations showed that even if the stellar density was high (≳104 pc−3), the strong far-ultraviolet (FUV) radiation still destroyed the disk effectively. Therefore, they considered that disk evolution in clusters is dominated by external photoevaporation.
The first clue to disk dissipation by external photoevaporation was found in the Orion Nebula Cluster (ONC; e.g., Mundy et al. 1995; Bally et al. 1998; Williams et al. 2005; Mann & Williams 2010; Eisner et al. 2016). Radio observations toward the core of the ONC by Churchwell et al. (1987) indicated that the disk mass-loss rate in the Trapezium cluster around θ1 Ori C was as high as ∼10−7–10−6 M☉ yr−1. Kim et al. (2016) first discovered the protoplanetary disks near a B star in NGC 1997. They found that a relatively high mass-loss rate (∼10−9–10−8 M☉ yr−1) could be driven by the moderate FUV fluxes. Ansdell et al. (2017) conducted a high-sensitivity millimeter-wavelength survey of protoplanetary disks in the σ Orionis cluster with the Atacama Large Millimeter/submillimeter Array (ALMA). They also found clues of significant mass-loss rates driven by moderate FUV fluxes. Champion et al. (2017) investigated external photoevaporating disks in the ONC by combining the Herschel survey with ALMA observations. They proved that the disk evolution was driven by FUV photoevaporation and the mass-loss rates were about ∼10−7–10−5 M☉ yr−1.
Observations of disks in clusters promote the development of theoretical models of external photoevaporating disks (e.g., Johnstone et al. 1998; Störzer & Hollenbach 1999; Adams et al. 2004, 2006; Clarke 2007; Fatuzzo & Adams 2008; Anderson et al. 2013). These models reproduced the observed mass-loss rates and suggested that the mass-loss rate was proportional to the intensity of external UV radiation (e.g., Johnstone et al. 1998; Adams et al. 2004; Anderson et al. 2013). Disk depletion simulations also predict that due to high mass-loss rates, the lifetimes of disks in clusters are much shorter than those in isolated stars fields, and a large fraction of disk mass is removed by external photoevaporation (e.g., Richling & Yorke 1997; Hollenbach et al. 2000; Scally & Clarke 2001; Takeuchi et al. 2005; Mitchell & Stewart 2010; Anderson et al. 2013; Kalyaan et al. 2015; Facchini et al. 2016). As a result, the rapid disk destruction in clusters severely suppresses gas giant planet formation (e.g., Armitage 2000; Holden et al. 2011; Thompson 2013; Ndugu et al. 2018).
Nevertheless, Stolte et al. (2010) found strong disk emissions in the Arches cluster, which hosted 125 O-type stars (Figer et al. 2002; Stolte et al. 2005). Considering the cluster age is about 2.5 Myr (Najarro et al. 2004), this finding is surprising. According to the theoretical models presented above, disk lifetimes should be very short (≪1 My) in such a starburst environment (Alonso-Albi et al. 2009; Espinoza et al. 2009). Richert et al. (2015) also found no evidence for disk depletion in the vicinity of O-type stars in the data set of the Massive Young Star-forming Complex Study in Infrared and X-ray (MYStIX), which surveyed 20 young OB-dominated regions with X-ray and infrared photometry (Feigelson et al. 2013). In addition, Malavolta et al. (2016) and Brucalassi et al. (2017) observed gas giant planets in open clusters M44 and M67, respectively. These findings of disks and giant planets in clusters provide important hints for us to understand disk evolution and planet formation.
Usually, there are massive OB stars in the dense stellar clusters (e.g., Matzner 2002; Koenig et al. 2008; Deharveng et al. 2012; Lindberg et al. 2015, 2017). These stars heat nearby dense clouds (e.g., Krumholz et al. 2014; Motte et al. 2017). Hatchell et al. (2013) measured the gas temperature of NGC 1333 by estimating the dust temperature with 450 and 850 μm submillimeter data from SCUBA-2 on the James Clerk Maxwell Telescope (JCMT). They found that the northern (IRAS 6/8) region of NGC 1333 was heated by the B star SVS3 and the dust temperature of this region was about 20–40 K. Rumble et al. (2015) also found observational evidence for radiative heating by massive stars in the Serpens WMC 297 region. The star WMC 297 heats the nearby clumps, for which the mean temperature is about 32–46 K. Lindberg et al. (2015) studied the excitation of some complex species in the Corona Australis star-forming region. They found that the H2CO rotational temperatures of the sources near the Herbig Be star R CrA were as high as 30–50 K. Recently, Lindberg et al. (2017) presented APEX 218 GHz observations of molecular emission in the Ophiuchus star-forming region. The H2CO temperatures of protostellar cores in this region are 16–124 K due to external heating by nearby massive stars. Simulations have shown that when the dense cloud core suffered radiative heating from the nearby massive stars, they were suppressed to fragment (e.g., Bate 2009; Offner et al. 2009; Hennebelle & Chabrier 2011).
Since protoplanetary disks form from the gravitational collapse of pre-stellar cores in molecular clouds (Shu 1977; McKee & Ostriker 2007), the core properties determine the properties of the formation and evolution of inherited disks (e.g., Cassen & Moosman 1981; Boss 1993; Kratter et al. 2010; Vorobyov 2010; Zhu et al. 2010). Cassen & Moosman (1981) first proved that the size, mass, and surface density profile of a disk were determined by the distribution of mass and angular momentum of its progenitor core. Then, Terebey et al. (1984) obtained the analytic solutions for the collapse of a rotating cloud core. Based on this work, Nakamoto & Nakagawa (1994), Jin & Sui (2010), and Zhu et al. (2010) investigated the dependence of the long-term evolution of protoplanetary disks on the cloud core properties. Numerical simulations also revealed that the efficiency of X-ray photoevaporation from the central protostar was related to the angular momentum of its pre-stellar core (e.g., Bae et al. 2013; Kimura et al. 2016; Xiao et al. 2016). Zhang & Jin (2015) and Xiao et al. (2017) suggested that the diversity of snow-line evolution in disks was the result of the diversity of cloud core properties. Recently, Xiao & Chang (2018) noticed that by influencing the efficiency of external photoevaporation, pre-stellar core properties also determined the evolution of disks with external photoevaporation in clusters. If the angular momentum of a core is small, most materials of the core collapse onto the central protostar and the disk size is small. External photoevaporation is suppressed. On the contrary, if the angular momentum of a core is large, most materials fall onto the disk far from the central star, and external photoevaporation is very strong. As a result, the disk mass is severely evaporated.
However, all of the investigations presented above have assumed that protoplanetary disks formed in cold environments (∼10–15 K). We still do not know how disks evolve in hot cluster environments. In this work, we explore the influence of hot background temperatures of open stellar clusters on the efficiency of external photoevaporation. This paper is organized as follows. In Section 2, we describe our disk model, including cloud core properties, basic equations, and external photoevaporation. In Section 3, calculation results of the disk evolution with external photoevaporation in hot background environments are presented, and we also discuss the implications of our calculation results for the observations. Model assumptions and limitations are discussed in Section 4. In Section 5, we present the conclusions of this study.
2. Models for Disk Formation and Evolution
The models for disk formation and evolution were introduced in Xiao & Chang (2018). In this work, we briefly review the α-disk model to interpret the influence of background temperatures on the disk properties. For details of the disk model, see Xiao & Chang (2018).
2.1. Initial Conditions
Our model begins with the gravitational collapse of pre-stellar cores. We assume that the pre-stellar core is an isothermal sphere (e.g., Ebert 1955; Bonnor 1956; Bacmann et al. 2000; Alves et al. 2001) that rotates uniformly (Goodman et al. 1993) with angular momentum,

where G is the gravitational constant, μ = 2.33 is the mean molecular weight, is the gas constant, and ω, Tcd, and Mcd are the angular velocity, temperature, and mass of a pre-stellar core, respectively, which represent the initial conditions for the protoplanetary disk formation. Shu (1977) found the self-similar solution for the collapse of an isothermal sphere and evaluated the mass infall rate onto the protostar+disk system,
, where a is the isothermal sound speed. So, the collapse time is

which is proportional to Mcd and inversely proportional to . Nakamoto & Nakagawa (1994) expressed the mass influx,
, onto the disk,

where represents the maximum radius of the core mass collapsed:
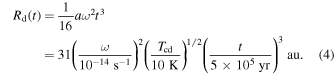
The observed range of ω is about 0.1–13 × 10−14 s−1 (Goodman et al. 1993; Jijina et al. 1999; Caselli et al. 2002). As discussed in the previous section, stellar clusters may have hot background temperatures due to the presence of massive OB stars. We estimate that Tcd is about 40–100 K near the massive stars in these clusters (e.g., Hatchell et al. 2013; Lindberg et al. 2015, 2017; Rumble et al. 2015).
We notice that for a pre-stellar core with fixed ω and Mcd, J is relatively low if Tcd is high (Equation (1)). As a result, the core mass falling directly onto the disk is small (Equation (3)). In addition, when Tcd is high, tinf becomes short (Equation (2)). According to Equation (4), the disk size is smaller during the core collapse. We expect that the characteristics of disks in clusters are very different from those in isolated star fields due to the difference in Tcd.
2.2. The Disk Model
According to mass and angular momentum conservation (Jin & Sui 2010; Zhu et al. 2010), the evolution of the disk is governed by Xiao & Chang (2018),
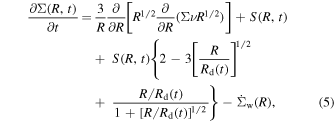
where Σ is the disk surface density, is the loss of surface density due to photoevaporation, and ν is the effective viscosity, which is expressed as
(Shakura & Sunyaev 1973), where h is the half thickness of the disk, α ≲ 1 is a dimensionless parameter reflecting the viscosity strength, and cs is the sound speed,

where Tm is the disk midplane temperature.
The radiative diffusion approximation is adopted to calculate Tm according to the disk surface temperature Ts (Nakamoto & Nakagawa 1994). By considering the thermal equilibrium between the cooling and heating fluxes in the disk, Ts and Tm are expressed as

and

where σ is the Stefan–Boltzmann constant, τp = κpΣ is the Planck mean optical depth, κp is the Planck mean opacity, is the viscous dissipation rate,
is the energy generation rate by shock heating, Tir is the effective temperature of the irradiation suffered by the disk (Hueso & Guillot 2005), τR = κRΣ is the Rosseland mean optical depth, and κp = 2.39κR is the Rosseland mean opacity (Nakamoto & Nakagawa 1994; Bell et al. 1997). The details of calculating Ts and Tm are presented in Nakamoto & Nakagawa (1994) and Jin & Sui (2010).
Xiao & Chang (2018) considered that α varied with R and t,

where αGI and αMRI are the viscosity parameters caused by the gravitational instability (GI; Laughlin & Bodenheimer 1994; Laughlin & Rozyczka 1996; Laughlin et al. 1997, 1998) and the magnetorotational instability (MRI; (Balbus & Hawley 1991, 1998), respectively, and αmin is assumed to be the minimum value for the viscosity parameter. We use the model developed by Kratter et al. (2008) to calculate αGI, which is , where
,
, u is the ratio of the disk mass to the mass of the protostar+disk, and Q is the Toomre parameter (Toomre 1964),

The value of αMRI is determined according to the numerical results of Fleming & Stone (2003). If both GI and MRI cannot survive, we consider that α = αmin = 5 × 10−4, which is the mean value of Dubrulle (1993), Klahr & Bodenheimer (2003), and Chambers (2006). To summarize, during the early evolution of the disk, GI provides the turbulent viscosity, and when the disk becomes gravitationally stable, MRI or hydrodynamic processes provide the turbulent viscosity. The details of calculating α are presented in Jin & Sui (2010) and Xiao & Chang (2018).
We notice that ν and Q are the functions of cs, which is determined by Tm. Equation (8) shows that the disk temperature is higher in clusters with massive stars than that in isolated star fields. So, for disks in clusters, the transports of mass and angular momentum are more efficient due to high ν (Shakura & Sunyaev 1973). The disk lifetimes should be shorter. In addition, Q is large for the disk in clusters due to a high disk temperature (Equation (10)). The GI may be difficult to operate.
In this work, radiation from both the host star and external stars provides the photoevaporation of protoplanetary disks in clusters, which means (Anderson et al. 2013; Xiao & Chang 2018). For internal X-ray photoevaporation,
, Owen et al. (2012) showed that
, where
was presented in their Appendix B. The scale coefficient, A, is determined by

where is the total mass-loss rate due to X-ray photoevaporation (Owen et al. 2011, 2012),
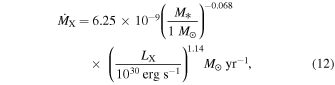
where M* is the central star mass and LX is the X-ray luminosity. Bae et al. (2013) considered that LX is a function of M* for a low-mass star :

For external FUV photoevaporation, , it is expressed as (Anderson et al. 2013; Xiao & Chang 2018)

where the parameter , which is estimated by fitting the numerical results shown in Figure 4 of Adams et al. (2004) with Equation (A3) in their paper, and Rg is the gravitational radius (Anderson et al. 2013),

where k is the gas constant, and TFUV is the function of the FUV flux G0 (Kalyaan et al. 2015),

For details of the external photoevaporation model, see Adams et al. (2004), Anderson et al. (2013), and Xiao & Chang (2018).
3. Calculation Results
Our models start from the collapse of a molecular cloud core. We set Mcd = 1.0 M☉ to study the disk formation around low-mass stars. The external FUV flux is set to be G0 = 3000 Habings, where Habings = 1.6 × 10−3 erg cm−2 s−1 (Habing 1968). The disk is axisymmetric. We divide the disk radially into 300 logarithmically spaced points. We adopt a zero-torque condition at the inner boundary Rin with Rin = 0.3 au. In order for the disk to expand freely (Bath & Pringle 1981; Ruden & Lin 1986), we set the outer boundary Rout at Rout = 50,000 au. The explicit finite-difference integration scheme is adopted to solve Equation (5). After that, disk mass, Mdisk, and disk size, Rdisk, are calculated, which are introduced in Anderson et al. (2013).
Numerical simulations have proved that external FUV photoevaporation operated in the outer region of the disk (Adams et al. 2004; Anderson et al. 2013). As shown by Xiao & Chang (2018), the efficiency of external FUV photoevaporation is determined by the depth of the gravitational potential well of the star+disk system. When M* is low or Rdisk is large, the gravitational potential well is shallow in the outer disk. In this case, external FUV photoevaporation is very effective. Xiao & Chang (2018) revealed that the ω of a pre-stellar core determined the evolution of M* and Rdisk. So, the efficiency of external photoevaporation is different in disks with different ω. For the low-ω case (Figures 7–9 in Xiao & Chang 2018), the angular momentum, J, of the core is small (Equation (1)). Most of the core mass is captured by the central star during the core collapse. The central star grows fast, and Rdisk. External photoevaporation is weak. After core collapse ends, the disk expands further due to viscosity. External photoevaporation becomes effective. The disk is truncated in the outer region and evaporates from the outside in. For the large-ω case shown in Figures 11–13 in Xiao & Chang (2018), most of the core mass falls directly onto the disk far from the central star. As a result, M* is low but Rdisk is large. External photoevaporation is so high that the disk mass is mainly removed by external FUV radiation, which results in the rapid shrink of Rdisk and the short disk lifetime. In this work, we investigate the disk evolution in hot cluster environments and show how Tcd influences the efficiency of external photoevaporation.
3.1. Evolution of Photoevaporating Disks in the Warm Regions of Stellar Clusters
As discussed in the Introduction, the massive OB stars in clusters may heat the nearby clumps, for example, the northern (IRAS 6/8) region of NGC 1333 and the Serpens WMC 297 region (Hatchell et al. 2013; Rumble et al. 2015). The mean temperature of these regions is about 40 K. Figure 1 shows the evolution of Σ and Q of a photoevaporating disk in clusters for G0 = 3000 Habings, with core properties ω = 13 × 10−14 s−1, Tcd = 40 K, and Mcd = 1.0 M☉. The disk evolution is dramatically different from that shown in Section 3.2 in Xiao & Chang (2018). They found that in clusters with low Tcd (Tcd = 10 K), when ω > 5.0 × 10−14 s−1, the falling material from the pre-stellar core was evaporated immediately due to the powerful external FUV photoevaporation. According to Equation (2), tinf decreases with increasing Tcd. Since J is smaller for the high-Tcd case with Tcd = 40 K (Equation (1)), most of the core material falls onto the disk close to the central star and then is accreted (Equation (4)). Therefore, the gravitational potential well of the disk has been deep during the core collapse. External FUV photoevaporation is suppressed (Adams et al. 2004). The mass acquired from the pre-stellar core exceeds the mass loss accreted and evaporated, so the disk expands outward, as shown in Figure 1(a). Meanwhile, the minimum value of Q in the outer radius of the disk gradually decreases and becomes smaller than 1.3 (Figure 1(c)). Since Σ increases and Tm is low in the outer region of the disk, the disk is gravitationally unstable during the core-collapse stage. The duration of the unstable state is short due to the short tinf. After the mass supply stops, Σ decreases with t due to the disk viscosity caused by the MHD turbulence or hydrodynamic processes (Figure 1(b)). The disk becomes gravitationally stable (Figure 1(d)). Its lifetime is about 4.50 × 106 yr. We show that the evolutionary features displayed in Figure 1 are similar to those shown in Figure 2 of Xiao & Chang (2018) with ω = 1.0 × 10−14 s−1 and Tcd = 10 K, because the total angular momenta (J; Equation (1)) are roughly the same for these two cores. The relatively low J means that most of the core mass is acquired by the central star. External photoevaporation is moderate. The disk evolution in these two cases is determined mainly by their own physical properties, such as MRI or hydrodynamic processes (e.g., Jin & Sui 2010).
Figure 1. Evolutions of Σ and Q of the disk for G0 = 3000 Habings, with core properties ω = 13 × 10−14 s−1, Tcd = 40 K, and Mcd = 1.0 M☉. The core collapse lasts about 7.96 × 104 yr. The left column represents the physical quantities before the core collapse stops. The right column represents the same physical quantities after the collapse stops. See the text for details.
Download figure:
Standard image High-resolution imageFigure 2(a) shows the evolution of Mdisk and M* for the disk with parameters shown in Figure 1. As discussed in the previous paragraph, the mass supply from the core exceeds the mass loss due to photoevaporation. So, both Mdisk and M* increase in the core-collapse stage. Although ω = 13 × 10−14 s−1 is large, Tcd = 40 K is relatively high. As a result, the central star gets a large amount of material from the pre-stellar core due to the low J (Equation (1)). When the collapse stops, M* is about 0.58 M☉. The gravitational potential well is so deep that external FUV photoevaporation is weak. After the collapse stops, Mdisk decreases from 0.43 to 0 M☉ within 4.4 × 106 yr due to the disk viscosity (Jin & Sui 2010) and photoevaporation (Xiao & Chang 2018). Because the efficiency of external photoevaporation is relatively low, about one half of Mdisk is accreted onto the central star (M* ∼ 0.82 M☉).
Figure 2. Evolution of mass and radius for the disk with the same core properties as shown in Figure 1. (a) Evolution of Mdisk (solid line) and M* (dashed line). (b) Evolution of Rdisk (dashed line) and Rg (solid line).
Download figure:
Standard image High-resolution imageIn Figure 2(b), we show the evolution of Rdisk for the disk with the parameters shown in Figure 1. Since M* grows quickly, as shown in Figure 2(a) during the core collapse, Rg increases rapidly to 270 au within ∼105 yr. Due to the mass supply from the pre-stellar core, Rdisk also increases quickly at the same time (Rdisk ∼ 110 au). After the collapse stops, external FUV photoevaporation coupled with viscous dissipation makes Rdisk shrink from 110 to 0 au within 4.4 × 106 yr, although the external photoevaporation becomes more and more weak.
Next, we present the effects of ω on the evolution of photoevaporating disks in warm clusters. Our calculations have indicated that when ω is lower than a critical value, no disk forms, and all of the core mass is captured by the central star (Xiao & Chang 2018). In this work, we show that the critical value of ω, ωcrit, increases with Tcd. When Tcd = 40 K, ωcrit is about 2.0 × 10−14 s−1, which is larger than that shown in Xiao & Chang (2018; ωcrit ∼ 0.3 × 10−14 s−1 for Tcd = 10 K). Figure 3 shows the evolution of Σ and Q for a photoevaporating disk (G0 = 3000 Habings) in clusters for the low-ω case, with core properties ω = 2.0 × 10−14 s−1, Tcd = 40 K, and Mcd = 1.0 M☉. The evolutionary trend of Σ is similar to the case with ω = 13 × 10−14 s−1 shown in Figure 1. The main differences are that the disk lifetime is shorter (∼1.35 × 106 yr) and Rdisk is smaller (Figure 4). In addition, the gravitational potential well is deeper, which results in the more ineffective external photoevaporation. Therefore, the disk evolves fast due to its viscosity. As shown in Figures 3(c) and (d), Q is high within the disk lifetime, with a minimum value larger than 6. The disk is always gravitationally stable.
Figure 3. Evolution of Σ and Q of the disk for G0 = 3000 Habings, with core properties ω = 2 × 10−14 s−1, Tcd = 40 K, and Mcd = 1.0 M☉. The core collapse lasts about 7.96 × 104 yr. The left column represents the physical quantities before the core collapse stops. The right column represents the same physical quantities after the collapse stops. See the text for details.
Download figure:
Standard image High-resolution imageFigure 4. Evolution of mass and radius for the disk with the same core properties as shown in Figure 3. (a) Evolution of Mdisk (solid line) and M* (dashed line). (b) Evolution of Rdisk (dashed line) and Rg (solid line).
Download figure:
Standard image High-resolution imageIn Figure 4(a), we show the evolution of Mdisk and M* for the disk with parameters shown in Figure 3 (ω = 2.0 × 10−14 s−1, Tcd = 40 K, and Mcd = 1.0 M☉) and external FUV fields (G0 = 3000 Habings). The evolution of Mdisk and M* is similar to that shown in Figure 2. As discussed in the previous paragraph, the low J of the core originating from the low ω causes M* to be higher and Mdisk to be much lower than that for the case in Figure 2. When the collapse ends, M* is about 0.97 M☉, and Mdisk is only about 0.02 M☉. Since the gravitational potential well is very deep, the external FUV photoevaporation is very weak. Finally, the central star acquires almost the entire core mass within the disk lifetime (M* ∼ 0.99 M☉). Figure 4(b) shows the evolution of Rdisk for the disk with parameters shown in Figure 3. The low ω means that most core mass falls directly onto the central star or the disk near the star. Therefore, Rg grows quickly to its final value of ∼394 au, and Rdisk is very small (<20 au). The disk evolution is primarily determined by the core properties (e.g., ω) rather than external photoevaporation (Xiao & Chang 2018).
Comparing with the studies of Xiao & Chang (2018), we find that the external photoevaporation is suppressed in warm clusters with Tcd ∼ 40 K. The effectiveness of external photoevaporation is mainly determined by the initial angular momentum of the progenitor core of the disk. Therefore, the core properties should be considered when investigating the evolution of a photoevaporating disk. The external photoevaporation becomes important only when the disk can expand to a few tens of au (Anderson et al. 2013; Kalyaan et al. 2015). In this case, the gravitational potential well of the disk in the outer edge is shallow, and the disk gas can easily escape. For a warm cloud core, J is relatively small. A large amount of collapsing core mass concentrates near the central star, most of which is accreted onto the star because of the disk viscosity originating from the MHD turbulence or hydrodynamic processes (Jin & Sui 2010). As a result, the effect of external photoevaporation is weakened due to the small size of Rdisk. Observations of molecular cloud cores suggested that the most frequent value of ω was about 1–2 × 10−14 s−1 (e.g., Goodman et al. 1993; Jijina et al. 1999; Caselli et al. 2002). Our calculations imply that for the core with Mcd ∼ 1 M☉ in warm clusters, the lifetime of the inherited disk is ∼106 yr, and only a few disks can last as long as ∼5 × 106 yr. It is very different from that shown in Xiao & Chang (2018), who suggested that disk lifetimes in cold clusters are >5 × 106 yr.
3.2. Evolution of Photoevaporating Disks in the Hot Regions of Stellar Clusters
Simulations of molecular clouds have suggested that radiative heating of a cloud could suppress it to fragment and collapse (e.g., Bate 2009; Offner et al. 2009; Hennebelle & Chabrier 2011). However, observations found evidence of the presence of disks in the hot regions of stellar clusters (e.g., Stolte et al. 2010; Richert et al. 2015). In this work, we assumed that the pre-stellar core is able to collapse even in hot environments. Figure 5 shows the evolution of Σ and Q for a photoevaporating disk (G0 = 3000 Habings) in the hot cluster regions, with core properties ω = 13 × 10−14 s−1, Tcd = 100 K, and Mcd = 1.0 M☉. Since Tcd is high, the collapse time, tinf, is very short (∼2 × 104 yr). Although ω is large in this case, the J of the core is still very low due to the high Tcd (Equation (1)). Most of the core material falls directly onto the central star during the very short collapse stage. The external photoevaporation is too ineffective to influence the disk evolution. Under the action of disk viscosity, Σ decreases with t (Figure 5). In this case, almost all of the core mass is captured by the central star, and Mdisk is very low (Figure 6(a)). Figure 6(b) shows that Rdisk is rather small (<17 au), so the disk is gravitationally stable all the time (Figure 5). The disk lifetime is short (∼1.2 × 106 yr).
Figure 5. Evolution of Σ and Q of the disk for G0 = 3000 Habings, with core properties ω = 13 × 10−14 s−1, Tcd = 100 K, and Mcd = 1.0 M☉. The core collapse is very short, 2 × 104 yr. The left column represents the profiles of Σ in different t. The right column represents the profiles of Q in different t. See the text for details.
Download figure:
Standard image High-resolution imageFigure 6. Evolution of mass and radius for a disk with the same core properties as shown in Figure 5. (a) Evolution of Mdisk (solid line) and M* (dashed line). (b) Evolution of Rdisk (dashed line) and Rg (solid line).
Download figure:
Standard image High-resolution imageWe finally present the disk evolution with higher G0. Figure 7 shows the evolution of Σ and Q for a photoevaporating disk (G0 = 30,000 Habings) in the hot cluster regions, with core properties ω = 13 × 10−14 s−1, Tcd = 100 K, and Mcd = 1.0 M☉. The evolution of Mdisk, M*, and Rdisk is shown in Figure 8. We find that the evolution of the disk with G0 = 30,000 Habings is the same as the case of G0 = 3000 Habings with the same core properties adopted, which illustrates that the disk properties in the hot environments are determined by their progenitor core properties rather than the radiative intensity of external FUV fields. We show that in the hot regions of clusters (Tcd ∼ 100 K), J is low for the core with Mcd ∼ 1 M☉, which causes Rdisk to be small with Rdisk < 20 au, and Mdisk is also low, with Mdisk < 0.02 M☉. The disk lifetime is very short (<106 yr).
Figure 7. Evolution of Σ and Q of the disk for G0 = 30,000 Habings, with the same core properties as in Figure 5. The left column represents the profiles of Σ in different t. The right column represents the profiles of Q in different t. See the text for details.
Download figure:
Standard image High-resolution imageFigure 8. Evolution of mass and radius for the disk with the same core properties as shown in Figure 7. (a) Evolution of Mdisk (solid line) and M* (dashed line). (b) Evolution of Rdisk (dashed line) and Rg (solid line).
Download figure:
Standard image High-resolution image3.3. Evolution of Photoevaporating Disks with High Core Mass
We have shown that for the core with Mcd ∼ 1.0 M☉, Rdisk is expected to be ≲100 au when the background temperature is ≳40 K. According to Equation (1), the J of the core is also the function of Mcd. In this subsection, we investigate whether a disk with large Rdisk in warm clusters could form from a massive cloud core. The evolution of Σ and Q of a photoevaporating disk (G0 = 3000 Habings) in clusters, with core properties ω = 13 × 10−14 s−1, Tcd = 40 K, and Mcd = 3.0 M☉, are shown in Figure 9. Obviously, when Mcd increases, Σ becomes higher compared with the case shown in Figure 1. Therefore, Mdisk and M* are both higher with Mdisk ∼ 1.1 M☉ and M* ∼ 1.0 M☉ when the core collapse ends (Figure 10). However, J also becomes large with increasing Mcd (Equation (1)). As a result, Rdisk is larger with Rdisk ∼ 340 au after the core collapse ends. In this case, the external photoevaporation is effective. In addition, the internal X-ray photoevaporation rate is also high due to the high M* (Equation (12)). So, the decreasing of Mdisk and Rdisk are both more rapid than the case shown in Figure 2. The final M* is about 1.2 M☉, which means that about 60% of Mcd is evaporated by the internal and external photoevaporation.
Figure 9. Evolution of Σ and Q of the disk for G0 = 3000 Habings, with core properties ω = 13 × 10−14 s−1, Tcd = 40 K, and Mcd = 3.0 M☉. The core collapse lasts about 1.9 × 105 yr. The left column represents the physical quantities before the core collapse stops. The right column represents the same physical quantities after the collapse stops. See the text for details.
Download figure:
Standard image High-resolution imageFigure 10. Evolution of mass and radius for the disk with the same core properties as shown in Figure 9. (a) Evolution of Mdisk (solid line) and M* (dashed line). (b) Evolution of Rdisk (dashed line) and Rg (solid line).
Download figure:
Standard image High-resolution imageFigure 11 shows the evolution of Σ and Q of a photoevaporating disk (G0 = 3000 Habings) in the hot cluster regions, with core properties ω = 13 × 10−14 s−1, Tcd = 100 K, and Mcd = 3.0 M☉. The evolution of Mdisk, M*, and Rdisk is shown in Figure 12. As discussed in Section 3.2, J is lower for hot molecular cloud cores. So, Mdisk is lower (<1.0 M☉) and Rdisk is smaller (∼100 au) compared with the case shown in Figure 10. Most of Mcd is accreted onto the central star (Figure 12). External photoevaporation is still suppressed, although Mcd is larger (Mcd = 3.0 M☉).
Figure 11. Evolution of Σ and Q of the disk for G0 = 3000 Habings, with core properties ω = 13 × 10−14 s−1, Tcd = 100 K, and Mcd = 3.0 M☉. The core collapse is very short, 6 × 104 yr. The left column represents the profiles of Σ in different t. The right column represents the profiles of Q in different t. See the text for details.
Download figure:
Standard image High-resolution imageFigure 12. Evolution of mass and radius for the disk with the same core properties as shown in Figure 11. (a) Evolution of Mdisk (solid line) and M* (dashed line). (b) Evolution of Rdisk (dashed line) and Rg (solid line).
Download figure:
Standard image High-resolution image3.4. Comparison with Other Studies
Our studies simulate the disk formation from the collapse of a molecular cloud core, which shows that Rdisk never exceeded Rg. Adams et al. (2004) explained how the external photoevaporation operated in these subcritical disks. Due to the external FUV radiation, some of the gas near Rdisk expands to a larger distance. When the gas temperature is high enough, it will escape from the disk and form the outflow. Adams et al. (2004) derived the analytic approximations for the mass-loss rates from the disk outer edge and surface, which are both dominated by the exponential term (Equations (A7) and (A8) in Adams et al. 2004). They found that the mass-loss rate from the outer edge was much higher than that from the disk surface. So, many papers that investigate the viscous evolution of disks in clusters include the external photoevaporation from the disk outer edge only (e.g., Clarke 2007; Kalyaan et al. 2015; Rosotti et al. 2017; Haworth et al. 2018). In this study, we adopt the method as reported in Anderson et al. (2013) to handle the external photoevaporation. Since the mass-loss rate is dominated by the exponential term, Equation (14) can capture the features of the external photoevaporation discussed above. To quantitatively illustrate how disk evolution changes between the two methods mentioned above, we adopt Equation (24) from Kalyaan et al. (2015) to simulate disk evolution with the same core properties as shown in Figure 1. We show that the evolution of Σ, Mdisk, and Rdisk in Figures 13–15 is very similar to that shown in Figures 1 and 2. Therefore, the formula for mass-loss rate adopted in this study does not change the features of external photoevaporation in subcritical disks. Since Rdisk ≪ Rg, the effect of external photoevaporation is weak, and the disk viscosity drives mass accretion and transport of angular momentum. The main differences are that the maximum value of Rdisk is larger with the ratio of the maximum Rdisk for the two methods about 1.2, Rdisk shrinks more slowly, and the disk lifetime (∼4.82 × 106 yr) is slightly longer with the ratio of the disk lifetimes for the two methods about 1.1, as shown in Figure 15.
Figure 13. Evolution of Σ and Q of the disk for G0 = 3000 Habings, with core properties ω = 13 × 10−14 s−1, Tcd = 40 K, and Mcd = 1.0 M☉. The external photoevaporation rate is calculated according to Equation (24) from Kalyaan et al. (2015), who considered that the disk loses mass from its outer edge only. The core collapse lasts about 7.96 × 104 yr. The left column represents the physical quantities before the core collapse stops. The right column represents the same physical quantities after the collapse stops. See the text for details.
Download figure:
Standard image High-resolution imageFigure 14. Evolution of Mdisk (top panel) and M* (bottom panel) for the disk with the same core properties and mass-loss rate as shown in Figure 13. For comparison, the evolution of Mdisk and M* for the case shown in Figure 2 is also presented (red lines). The evolution of Mdisk and M* for the photoevaporating disks, in which two different formulae for external photoevaporation are adopted, is almost the same. This result proves that the method for handling disk mass loss reported by Anderson et al. (2013) captures the features of the external photoevaporation that happens primarily from the disk outer edge. Anderson et al. (2013) also included the mass loss from the disk surface, so Mdisk decreases more quickly in their models (red lines).
Download figure:
Standard image High-resolution imageFigure 15. Evolution of Rdisk and Rg for the disk with the same core properties and mass-loss rate as shown in Figure 13. The dashed line represents the evolution of Rdisk. The solid line represents the evolution of Rg. For comparison, the evolution of Rdisk and Rg for the case shown in Figure 2 is also presented (red lines). Since the formula for external photoevaporation adopted by Kalyaan et al. (2015) considers the mass loss from the disk outer edge only, the effect of the external photoevaporation is slightly weaker compared with the case shown in Anderson et al. (2013). Therefore, the maximum value of Rdisk is larger with the ratio of the maximum Rdisk for the two methods about 1.2, and Rdisk shrinks more slowly (black dashed line). The disk lifetime (∼4.82 × 106 yr) is slightly longer with the ratio of the disk lifetimes for the two methods about 1.1.
Download figure:
Standard image High-resolution imageWe notice that many studies of external photoevaporating disks do not consider the disk formation. For example, Anderson et al. (2013) and Kalyaan et al. (2015) set the initial values of M* = 1.0 M☉, Mdisk = 0.1 M☉, and Rdisk = 30–100 au. In this case, the external photoevaporation is moderate. Most of the disk mass is accreted onto the central star due to the disk viscosity. The effects of external photoevaporation are truncating Rdisk and dissipating the disk rapidly from the outside in when the disk approaches its lifetime. In this study, we show that the evolution of a external photoevaporating disk may be very different for different core properties. The initial value of J of the core determines the effectiveness of the external photoevaporation. If J is low (for example, Tcd = 100 K and Mcd = 1.0 M☉ or Tcd = 40 K, Mcd = 1.0 M☉, and ω = 2 × 10−14 s−1), most of core mass collapses onto the central star, and Rdisk is very small (<20 au). The external photoevaporation is severely suppressed. In this case, for a core with Mcd ≳ 1.0 M☉, M* should be high (M* > 1.0 M☉), but Mdisk should be low (Mdisk/M* ≪ 1). If J is very high (for example, Tcd = 10 K and ω > 5 × 10−14 s−1), as discussed in Xiao & Chang (2018), most of core mass falls onto the disk far from the central star. The external photoevaporation is very strong, and Mdisk is severely evaporated. In this case, both Mdisk and M* are very low. However, when J is moderate (for example, Tcd = 40 K and ω = 13 × 10−14 s−1), both Mdisk and M* are relatively high, and Rdisk becomes large after the core collapse ends. The external photoevaporation becomes important when the evolution of the disk approaches its lifetime. In this case, the disk evolution is similar to that shown in Anderson et al. (2013) and Kalyaan et al. (2015).
3.5. Implications
Theoretical studies of external photoevaporation in clusters have predicted that the diversity of the Mdisk − Rdisk relation originates from the diverse G0 and α (Anderson et al. 2013). However, the diversity of protoplanetary disks near massive stars in clusters (such as the core of Orion; e.g., Stolte et al. 2010; Richert et al. 2015; Gorti et al. 2016) may not be explained by this mechanism, because these disks are expected to have the same G0 and α. We notice that temperatures in the vicinity of massive stars may be relatively high, ∼35–70 K (e.g., Hogerheijde et al. 1995; Arab et al. 2012; Goicoechea et al. 2017). Since the background temperature (Tcd) is relatively high, the external photoevaporation is suppressed (Figure 7). The disk properties are determined by the initial cloud core properties. If ω or Mcd is large, Mdisk and Rdisk are both large. Otherwise, if ω and Mcd are small, Mdisk and Rdisk are both small. The diversity of core properties leads to the diversity of disk properties. The role of external photoevaporation is to prevent disk spreading and remove it from the outside in.
NGC 1333 is a very young stellar cluster (1–2 × 106 yr old) in the Perseus molecular cloud. Dodds et al. (2015) found that the disk mass in this cluster was very low. They discovered eight disks with one having a mass of about 0.009 M☉ and the others having only 0.002–0.004 M☉. Since the stellar density of NGC 1333 is low with <100 pc−3 (Huff & Stahler 2006; Scholz et al. 2013), dynamical interactions of stellar encounters may not work (Rosotti et al. 2014). Hatchell et al. (2013) confirmed that there were only B stars near NGC 1333, which implied that the effect of UV radiation on the disks was weaker than that in the ONC. However, Hatchell et al. (2013) found that the B star SVS3 was heating the part region of NGC 1333 and the temperature of this region was about ∼40 K. Our calculations in Figure 3 suggest that the disks found in NGC 1333 may form in the mild UV radiation environments from the pre-stellar core with warm background temperature ∼40 K and low ω (ω ∼ 2.0 × 10−14 s−1). As discussed in Xiao & Chang (2018), the low ω leads to the lower angular momentum of the cloud core. As a result, the disk is less massive, and its size is small. The disk lifetime is short, ∼1.35 × 106 yr.
We finally try to understand the formation of the 114–426 disk found in the ONC. Observations have shown that its diameter is as large as 950 au (McCaughrean et al. 1998; Bally et al. 2015). Bally et al. (2015) provided the details of the disk properties: the disk mass was relatively low, ∼3 × 10−3 M☉, and the central star had a mass of ∼1–1.5 M☉. In addition, they also found that this disk might be an evolved and depleted disk because of the absence of dense-gas tracers. Considering the background temperature is as high as 50–80 K, the formation of the disk is very interesting. The external photoevaporation model predicted that Rdisk should be very small (<100 au) because external photoevaporation truncated the disk and dissipated it from the outside in (e.g., Adams et al. 2004; Anderson et al. 2013; Kalyaan et al. 2015). Our calculations showed that only in the early evolution of the disk, Rdisk might be larger than ∼300 au. However, at this time, the disk is much higher than 0.1 M☉. The failure in explaining the formation of the 114–426 disk implies that the external photoevaporation may be very weak. The disk evolution is not determined by the core properties. This means that UV radiation is prohibited from irradiating the disk, or the disk is simply far from the UV radiation sources (Bally et al. 2015).
4. Discussion
In this study, we ignore the effects of magnetic fields, although observations infer that molecular cloud cores are magnetized (e.g., Li et al. 2014). The MHD simulations have shown that the angular momentum of the core is efficiently transported away by the well-known magnetic braking (e.g., Galli et al. 2006; Hennebelle & Fromang 2008; Mellon & Li 2008; Seifried et al. 2012). As a result, the formation of a rotation-supported disk is prevented even if nonideal MHD effects and magnetic field-rotation misalignment are considered (e.g., Hennebelle & Ciardi 2009; Machida & Matsumoto 2011; Krumholz et al. 2013). Seifried et al. (2015) showed that the presence of turbulence in the cloud core might resolve the "magnetic braking problem," which could reduce the magnetic braking efficiency sufficiently. Machida et al. (2014) showed that in order to form a disk, a high spatial resolution of <1 au must be satisfied. Machida et al. (2016) found that if the magnetized core was initially unstable, it evolved fast, and a large amount of angular momentum was introduced into the disk-forming region. In this case, the effect of magnetic braking is weakened. The disk formation is similar to the results that are shown in the hydrodynamic simulations (e.g., Seifried et al. 2015; Machida et al. 2018). The main differences are that the disk mass is lower and the disk size is smaller due to the weak magnetic braking.
The photoevaporative flow originates from the escape of gas heated by FUV photons. Strictly speaking, we need to calculate the grain photoelectric heating and electronic excitation of H2 due to FUV photon absorption in order to determine the temperature of the disk atmosphere (Adams et al. 2004; Facchini et al. 2016). Therefore, TFUV is a function of gas number density, FUV optical depth, and G0. According to the photodissociation region temperature profiles in Figure 2 of Adams et al. (2004), the temperature is nearly constant for a fixed G0 until visual extinction ≳0.2. We notice that the gas number density is usually no higher than 105 beyond Rdisk. Based on these considerations, we simplify the estimation of TFUV by assuming that it is a function of G0 only, although the change of gas number density can cause TFUV to become higher or lower by a factor Λ (where 0.5 ≲ Λ ≲ 2; Anderson et al. 2013). Figure 16 shows how Mdisk and Rdisk are influenced due to the fluctuation of TFUV (with different Λ). In most of the disk evolution, the disk evolves dominated by its own viscosity rather than photoevaporation. So, the fluctuation of TFUV hardly affects the evolution of Mdisk and M*. However, it mildly affects the evolution of Rdisk because external photoevaporation operates mainly at the disk outer edge. According to Equation (14), is a function of Rg/R and dominated by the exponential term. When Rg/R ≲ 4.6,
increases with Rg/R. However, when Rg/R ≳ 4.6,
decreases with Rg/R. Figure 16(b) shows that before the core collapse ends, Rg/R never exceeds 4.6. Since Rg/R is inversely proportional to TFUV (Equation (15)), the mass-loss rate is lower for higher TFUV, and Rdisk is larger. After the core collapse ends, Rdisk shrinks effectively due to external photoevaporation. In this stage, Rg ≫ Rdisk. As a result, the external photoevaporation is more effective for higher TFUV, which causes Rdisk to shrink more quickly, and the disk lifetime is shorter.
Figure 16. Comparison of Mdisk and Rdisk with the same core properties as shown in Figure 1 and different Λ. (a) Solid lines represent M*, and dashed lines represent Mdisk. (b) Solid lines represent Rdisk, and dashed lines represent Rg.
Download figure:
Standard image High-resolution image5. Conclusions
In this work, we explore the formation and evolution of photoevaporating disks in clusters with hot background temperatures. The model starts from the pre-stellar cloud core collapse. Theoretical studies suggest that the external photoevaporation becomes important when the disk can expand to a few tens of au (Adams et al. 2004; Anderson et al. 2013; Kalyaan et al. 2015). Since the initial angular momentum of the molecular cloud core determines the disk size during the disk formation, the core properties should be considered when investigating the evolution of a photoevaporating disk. We show that external photoevaporation is suppressed in the hot temperature environments and the disk properties are determined by the core properties, such as Tcd and ω.
In the warm region of clusters with Tcd = 40 K, external FUV photoevaporation is still ineffective even if ω is very high (ω = 13 × 10−14 s−1). The initial angular momentum is relatively low for this warm core (Equation (1)). Most of the core mass collapses onto the disk close to the central star and then is accreted (Equation (4)). The gravitational potential well of the disk is deep. The disk becomes gravitationally stable after the core collapse ends. The properties of the disk evolution in this case are quite different from those shown in Xiao & Chang (2018) with the same ω but low Tcd (Tcd = 10 K), where most of the core mass falls onto the disk far from the central star, and the disk is evaporated efficiently by the external FUV photoevaporation. As ω decreases, the angular momentum of the core becomes low enough that almost all of the core mass collapses directly onto the central star. The disk mass is very low (<0.02 M☉), and it size is very small (<20 au). Therefore, the disk lifetime is short, ∼106 yr. In the vicinity of massive stars, the temperatures of the molecular clouds may be as high as Tcd ∼ 100 K. In this case, almost all of the core mass is captured by the central star regardless of whether ω is high or low. The disk properties are determined by its progenitor core properties rather than the radiative intensity of external FUV fields. The disk lifetime is very short, <106 yr. We consider that the diversity of photoevaporating disks in clusters is not only the result of the diversity of G0 and α but also the result of the diversity of the progenitor cloud core properties. When the J of the core is low, the external photoevaporation is severely suppressed. As J increases, the external photoevaporation becomes important in truncating and dissipating the disk from the outside in when the disk approaches its lifetime. When J is very high, the external photoevaporation is very strong, and Mdisk is severely evaporated. We suggest that low-mass disks (0.002–0.004 M☉) in NGC 1333 with 1–2 × 106 yr are likely to form from the warm pre-stellar cores (>40 K) with low ω (<2 × 10−14 s−1).
We are grateful to the anonymous referee for constructive and fruitful comments that helped us improve our paper significantly. This research is supported by the National Natural Science Foundation of China (NSFC) grant No. 11703075, CAS "Light of West China" Program 2017-XBQNXZ-B-020, and the Heaven Lake Hundred-Talent Program of Xinjiang Uygur Autonomous Region of China. L.X. thanks Key Laboratory for the Structure and Evolution of Celestial Objects of CAS for foundation support (grant No. OP201711). L.P.J. acknowledges support from the National Natural Science Foundation of China (NSFC) grant No. 11373019.