Abstract
Solid-state UV light sources emitting below a wavelength of 250 nm (far UVC) are expected in various application fields. Although III-nitride semiconductors are promising material systems for this purpose, their emission efficiency becomes lower at shorter wavelengths. In this paper, we review two emerging singularity structures, which can improve emission efficiency. One structure is AlGaN quantum wells (QWs) formed on macrosteps due to step bunching. Compared with the adjacent planar QWs on atomically flat terraces, QWs on macrosteps have lower Al compositions and thicker wells. Consequently, they act as potential minima. Strong emissions are observed from QWs on macrosteps due to suppressed nonradiative recombination. The other structure is GaN QWs with monolayer-level thickness. Strong carrier confinement within ultrathin GaN QWs enhances radiative recombination. Additionally, the exclusion of Al from the well decreases cation vacancies and lowers the nonradiative recombination probability.
Export citation and abstract BibTeX RIS
1. Introduction
AlGaN-based quantum structures have been extensively studied due to their potential applications as UV emitters. 1–19) One target wavelength range is 260–280 nm because UV light in this range has germicidal effects. To date, the highest external quantum efficiency of AlGaN-based LEDs (∼20%) emitting below 300 nm has been achieved at 275 nm. 10) Currently, the interest in shorter wavelengths below 250 nm (far UVC) has increased 2,15,16) because far UVC can be used to detect toxic gases such as NOx and SOx , 20,21) and to inactivate viruses, including coronavirus, without harming mammalian skin. 22–24)
Wavelengths below 250 nm are typically achieved by increasing the Al composition in AlGaN quantum wells (QWs) as AlN has a wider bandgap (6.0 eV) at RT than GaN (3.4 eV). N. Lobo-Ploch et al. showed mW class emission at 233 nm from an LED with an Al0.72Ga0.28N/Al0.83Ga0.17N multiple QW emitter. 16) However, this approach has two issues. The first is a theoretically predicted reduction in the formation energy of group III vacancies. 25,26) Such a reduction tends to enhance nonradiative recombination (including recombination via deep levels), which leads to an inferior internal quantum efficiency (IQE). In fact, multiple papers have experimentally confirmed that AlGaN-based QWs emitting at shorter wavelengths exhibit lower IQEs. 27–29) Additionally, we recently demonstrated that the dominant recombination path of AlN at RT is carrier capture to the deep levels associated with Al vacancies, 30) which suggests that point defects play a role as undesirable recombination centers in Al-rich AlGaN.
The second issue is light extraction. The topmost valence band in AlN is the crystal-field split-off hole bands governed by the pz -like state, 31–36) whereas that in GaN is the heavy hole band governed by the px - and py -like states. 37–39) Here, z is parallel to [0001], and the x- and y-axes are in the (0001) c-plane. Therefore, the emission from AlN is predominantly polarized parallel to the c-axis ( E ∣∣ c), where E is the electric field vector of emitted light. By contrast, the emission from GaN is polarized perpendicular to the c-axis ( E ⊥ c). Consequently, light extraction along the [0001] direction becomes more difficult as the Al composition increases in AlGaN QWs.
This paper reviews two emerging singularity structures to circumvent these issues. In Sect. 2, AlGaN-based QWs fabricated on bunched macrosteps are discussed. In Sect. 3, GaN QWs with monolayer (ML)-level thicknesses are discussed. Both singularity structures enhance the radiative efficiency below ∼250 nm, showing promise in far-UVC emitters.
2. AlGaN QWs on macrosteps
2.1. Research trends
AlN layers grown on vicinal (0001) substrates often show wavy surfaces, which are composed of bunched macrosteps and atomically flat terraces. 40–54) Growing AlGaN QWs on such surfaces lowers the Al composition and widens the well widths on the bunched steps. 43,44,48–55) Similarly, compositional fluctuations have also been confirmed in AlGaN films grown on AlN with bunched steps. 40,46) Because wider well widths and lower Al compositions in QWs at bunched steps create potential minima, the optical transition energies of QWs on macrosteps (MS-QWs) are lower than those on adjacent planar terraces. Consequently, a double-peak emission is sometimes observed, which is unsuitable for many practical devices.
Multiple papers have proposed ways to diminish or eliminate step bunching on the underlying Al(Ga)N until the double-peak emission is eventually suppressed. Nagamatsu et al. demonstrated that introducing Mg to AlN effectively suppresses step bunching on AlN, and they achieved single-peak photoluminescence (PL) from an AlGaN film on AlN even on a vicinal substrate. 40) Bryan et al. reported that controlling the supersaturation and misorientation angle of substrates is important to eliminate step bunching on AlN. 45) Bellmann et al. investigated the effect of the substrate off-angle and V/III ratio. They revealed that higher V/III ratios can hinder step bunching due to the suppressed diffusivity of adatoms. 47)
In contrast, it is also interesting to use MS-QWs as carrier localization centers for highly efficient light emitters. For GaAs, QWs on macrosteps act as quantum wires (QWRs), 56,57) which theoretically have higher radiative recombination probabilities than those of planar QWs at RT because the spiky density of states of QWRs suppresses carrier thermalization at elevated temperatures. 58) For AlGaN, we fabricated QWR-like structures on (0001) AlN with bunched steps. 43) (The growth method is described below.)
Figure 1(a) shows a typical cross-sectional high-resolution (HR) image of an AlGaN/AlN (0001) MS-QW acquired by transmission electron microscopy (TEM). A thicker QW forms on a macrostep. Figure 1(b) shows the position dependence of the Al composition acquired by energy-dispersive X-ray spectroscopy (EDS) under the scanning TEM mode. For the estimation, the effect of the spread of irradiated electrons within the sample was calibrated according to the method in Ref. 59. MS-QWs have a lower Al composition compared with the adjacent planar QWs. Figures 1(a) and 1(b) indicate that MS-QWs can act as potential minima. In fact, spatially resolved luminescence maps show that the emission from QWs on macrosteps is located at a lower energy than that from QWs on planar terraces. 44,48,50–55,60) Interestingly, some research groups have reported that MS-QWs emit more strongly than terrace QWs at RT despite a smaller MS-QW volume. 44,49–52)
Fig. 1. (Color online) (a) Typical cross-sectional HRTEM image of an AlGaN QW grown on AlN with bunched steps. (b) Local EDS analyses of the Al compositions along the AlGaN QW.
Download figure:
Standard image High-resolution imageFigure 2 presents an example of a strong emission from MS-QWs, where an AlGaN single QW layer is embedded in AlN. Figure 2(a) is a cathodoluminescence (CL) spectrum at RT acquired with an acceleration voltage of 5 kV. The main peak at ∼255 nm is derived from MS-QWs, while the weak shoulder at ∼235 nm may be due to terrace QWs. Figure 2(b) is a typical surface image of the same sample taken by scanning electron microscopy (SEM). Bunched steps are clearly visible. Figure 2(c) superimposes a panchromatic CL map over the SEM image in Fig. 2(b). It should be noted that the map and image cover the same area. A strong emission is observed from the step edges.
Fig. 2. (Color online) (a) CL spectrum of AlGaN MS-QWs at RT. Nealy single peak due to MS-QWs is observed. (b) Surface image observed by SEM. Lines indicate bunched steps. (c) CL map superimposed on SEM. Strong CL is observed along the step edges.
Download figure:
Standard image High-resolution imageFigure 2(a) suggests that the double peaks due to bunched steps and planar terraces are avoidable since a single peak emission from MS-QWs is achieved at RT. The reason remains unclear but it may be related to the growth conditions and structures. For example, Kojima et al. proposed that Al-rich regions formed in the underlying n-AlGaN layer restrict current paths within those regions and that the current is effectively injected into AlGaN MS-QWs through those paths. 51) In addition, Hayakawa et al. suggested partial carrier transfer from terraces to macrosteps. 44,50)
The CL intensity is generally determined by the product of IQE and the light extraction efficiency. However, nanostructures such as bunched steps have a negligible impact on the light extraction efficiency because their dimensions are much less than the wavelength. Therefore, the strong CL from MS-QWs suggests an improved IQE.
IQE is described as where
and
are the radiative and nonradiative recombination probabilities, respectively, and are the reciprocals of their respective lifetimes. This means that the improved IQE is a consequence of either an enhanced radiative recombination or a suppressed nonradiative recombination. The former is an original expectation deduced from GaAs-based QWRs on macrosteps. For conventional III–V QWRs such as GaAs QWRs
56) and InP QWRs,
61) a strong optical polarization along QWRs has been experimentally demonstrated. Such polarization is also supported theoretically.
62,63) To examine whether QWR-like electronic states form in the AlGaN-based MS-QWs, the in-plane optical anisotropy has been measured by PL spectroscopy at RT using an ArF laser (193 nm wavelength) as an excitation source.
Figure 3 shows the PL spectra from an AlGaN/AlN (0001) MS-QW sample, which is composed of an AlN underlying layer, a nominally 1.5 nm thick Al0.8Ga0.2N QW, and 15 nm thick AlN cap layer. The atomic force microscopy (AFM) image of the surface indicates that the misorientation direction is toward [100] with a 1° angle (Fig. 3, inset). Because {1
00} is a stable plane of AlN, the misorientation direction of [1
00] causes relatively regular, straight steps. This implies the formation of well-defined AlGaN QWs at macrostep edges. Nevertheless, the polarization PL spectra along either the [1
00] or [11
0] direction exhibit nearly the same intensity (Fig. 3), which suggests that QWR-like states are not formed. This observation can be accounted for by the exciton Bohr radius. For conventional III–V semiconductors such as GaAs and InP, the exciton Bohr radius is on the order of 10 nm, whereas that for AlN is about 2 nm. Therefore, even if the dimensions of nanostructures on macrofacets are similar, nanostructures can act as QWRs for conventional III–V semiconductors but not for AlGaN.
Fig. 3. (Color online) Polarization PL spectra of an AlGaN/AlN MS-QW at RT acquired along either [100] or [11
0]. Inset is a surface AFM image. Stable {1
00} plane straightens the steps because the misorientation direction of this sample is along the AlN [1
00] direction.
Download figure:
Standard image High-resolution imageThese two findings, a stronger emission at step edges and the lack of a QWR-like nature, suggest that the nonradiative recombination is suppressed at the step edge. To confirm this, we investigate the detailed optical properties in the next section.
2.2. Optical properties of AlGaN/AlN MS-QWs
The samples shown in this section were grown by metalorganic vapor phase epitaxy (MOVPE) on sapphire or AlN (0001) substrates tilted 3°. The misorientation is along either the sapphire [110] direction or the AlN [1
00] direction. Both directions cause the AlN epilayers to tilt along the AlN [1
00] direction. (The growth details are given in Ref. 43 for sapphire substrates and Ref. 64 for AlN substrates.) Growth at 1200 °C forms ∼600 nm thick AlN layers and creates macrosteps. Subsequent growth at 1140 °C realizes AlGaN single QWs and AlN cap layers (∼15 nm). The thickness and Al composition for an AlGaN QW are 1.5 nm and 80% in planar QWs, but those on macrosteps are thicker and smaller, respectively (Fig. 1).
Figure 4(a) shows the temperature dependence of the PL spectra of an AlGaN/AlN MS-QW grown on the 3°-off AlN (0001) substrate. The excitation source was the fourth harmonics of a picosecond (1.8 ps) pulsed Ti:sapphire laser emitting at 210 nm, which enables selective excitation of the QW. The excitation energy density per pulse was 5 nJ cm−2, and the estimated photo-excited carrier density was as low as ∼5 × 1014 cm−3 in a 1.5 nm thick planar QW. The weak excitation condition was adopted to highlight the effect of nonradiative recombination processes. The PL was detected by a CCD camera through a monochromator.
Fig. 4. (Color online) (a) Temperature dependence of the PL spectra and (b) that of the integrated PL intensity of the AlGaN QW on the 3°-off AlN substrate. For comparison, data for an AlGaN MS-QW and a planar QW on sapphire substrates are also shown. (Reproduced from Fig. 3 in Ref. 50 with permission.)
Download figure:
Standard image High-resolution imageAt 6 K, the PL spectrum has two components at 5.50 eV (225 nm wavelength) and ∼5.35 eV (232 nm). As the temperature increases, the main peak drastically weakens and eventually disappears at ∼210 K. On the other hand, the longer-wavelength component, which has a peak located at 5.12 eV (242 nm) at RT, survives up to RT. Considering the CL maps (e.g., Fig. 2), the dominant PL at RT is due to MS-QWs, while the shorter-wavelength PL observed at low temperatures is due to QWs on terraces.
The PL spectra in Fig. 4(a) can be decomposed into two Gaussian components corresponding to the emissions from QWs on macrosteps and terraces. Figure 4(b) plots the spectrally-integrated, respective Gaussian components as functions of the measurement temperature. For comparison, those of AlGaN QWs grown on the 3°-off and on-axis sapphire (0001) substrates are also plotted. The thermal quenching of the terrace QW grown on 3°-off sapphire is similar to that of the QW on on-axis sapphire, supporting our assignment of the shorter-wavelength emission to the terrace QW emission. The normalized PL intensity of the MS-QW on 3°-off sapphire at RT is one-order-of-magnitude higher than that of the planar QW, indicating a drastically improved IQE in QWs on macrosteps. Using an AlN vicinal substrate further suppresses thermal quenching, suggesting a better IQE in a MS-QW on AlN than on sapphire. In fact, the PL absolute intensity estimated under the same experimental setup is the strongest for a MS-QW on AlN.
As mentioned in the previous section, an enhanced radiative recombination probability is unlikely to improve IQE in the present MS-QWs. Thus, we considered the effect of the suppressed nonradiative recombination at RT. The time-resolved PL was performed at RT because carrier recombination processes are dominated by nonradiative recombination at this temperature. The experimental setup is the same as that for Fig. 4, except that PL was detected by a streak camera instead of CCD.
The PL decay curves of four different samples are compared. AlGaN/AlN QWs on the nominally on-axis (0001) sapphire and AlN substrates exhibit nearly the same lifetime (20–40 ps), despite their vastly different threading dislocation (TD) densities. The edge dislocation densities in the AlN epilayers derived from X-ray diffraction are 1 × 1010 cm−2 on sapphire and less than 105 cm−2 on the AlN substrate. This finding indicates that TDs do not play a key role in determining the recombination process in Al-rich AlGaN QWs at RT. However, point defects are crucial, as previously suggested. 30,65)
The MS-QW on sapphire shows a one-order-of-magnitude longer lifetime (268 ps) than that of the planar QW. The lifetime of the MS-QW on AlN is even longer (1.43 ns). Because longer PL lifetimes at RT suggest slower nonradiative recombination processes, the experimentally observed long lifetimes in the MS-QWs suggest a reduction of point defects or suppression of the probability of carrier capture at point defects. One possible mechanism is that the mass transport from the terrace to the macrostep regions during MOVPE growth increases the local supersaturation of group III adatoms, which inhibits the formation of cation vacancies in the film. Another possibility is that the formation of MS-QWs induces potential minima, which prevents carriers from being captured by nonradiative recombination centers. Furthermore, as shown in the inset of Fig. 3, macrosteps involve kinks, though the number of kinks is not so large. The kink sites may be sources of additional potential fluctuations, as suggested in Fig. 2.
We consider that the difference in the lifetimes of the MS-QWs on sapphire and AlN substrates is not a direct consequence of the difference in the TD densities, because the PL lifetimes of the planar QWs on sapphire and AlN are similar (20–40 ps). One possible cause is impurities: because oxygen can diffuse from sapphire substrates to AlN epilayers at high growth temperatures, oxygen-related deep levels can form in heteroepitaxial layers and act as an additional recombination channel. The suppressed nonradiative recombination in MS-QWs may have unveiled a difference due to impurities. Another possibility is that suppressing nonradiative recombination via point defects may have highlighted the difference in the TD densities.
Figure 5 plots the reported PL lifetimes at RT as a function of the emission energy. 8,66–69) A clear trend is not discernible between sapphire and AlN substrates. This is consistent with a weak influence of TDs on carrier recombination. As the emission energy increases, the PL lifetime tends to be shortened. This finding indicates faster nonradiative recombination at higher energies (shorter wavelengths) and is a negative factor to realize highly efficient UV emitters at shorter wavelengths. The PL lifetime of the MS-QW on the AlN substrate (1.43 ns) is also plotted in Fig. 5. The lifetime is much longer than the reported lifetimes in nominally planar QWs emitting at similar wavelengths, showing promise of MS-QWs as highly efficient UV emitters below 250 nm. Additionally, we examined another MS-QW emitting in the conventional germicidal wavelength (∼265 nm). Although the MS-QW is grown on sapphire, the PL lifetime at RT is as long as 3.2 ns under the same weak excitation conditions. (Vicinal AlN substrates have yet to be explored). The lifetime plotted in Fig. 5 indicates that MS-QWs effectively suppress nonradiative recombination in this spectral region.
Fig. 5. (Color online) Reported PL lifetimes of AlGaN QWs at RT as functions of the emission energy. Lifetimes of MS-QWs emitting at 242 or 265 nm are also plotted. (Reproduced from Fig. 6 in Ref. 50 with permission.)
Download figure:
Standard image High-resolution image2.3. Future prospects
MS-QWs have already been applied for LEDs emitting above 250 nm. 49,53) Therefore, one of the next targets is to fabricate and characterize MS-QW-based LEDs emitting below 250 nm. AlGaN/AlN MS-QWs display PL below 250 nm (Figs. 3 and 4). Thus, a remaining issue is related to device fabrication such as doping in Al-rich AlGaN.
Although we have suggested a few possible causes for the suppression of nonradiative recombination in MS-QWs, the physics behind it remains debatable. Further fundamental studies such as exploring the effect of growth conditions are necessary. Unveiling the mechanics will pave the way for reproducible fabrication of highly efficient UV emitters below 250 nm.
Furthermore, it will be interesting to reduce the dimension of MS-QWs. One benefit of smaller dimensions is shortening the emission wavelength. Another benefit is the possible appearance of the QWR-like nature. As described in Sect. 2.1, QWRs can heighten the radiative recombination probability at RT and can further improve IQE (and external quantum efficiency).
3. GaN ML QWs
3.1. Research trends
Since the pioneering work by Kahn et al., 70) many experimental and theoretical studies have examined ultrathin GaN wells with Al(Ga)N barriers. 71–89) Very recently, an excellent review paper was published, in which many aspects of ultrathin GaN wells such as (sub ML) digital alloys for p-doping or light-emitting layers, QWs, and superlattices are widely described. 90) Therefore, this paper concentrates on our interest, that is, UV emitters below a wavelength of ∼250 nm based on GaN ML QWs.
One important feature of GaN-based QWs is the absence of Al in the well region. Theoretical studies have predicted that AlN has a much lower formation energy of cation vacancies than GaN. 25,26) Therefore, using GaN QWs instead of conventional AlGaN QWs should suppress the cation-vacancy formation and lead to a higher IQE. In Sect. 3.2, carrier recombination dynamics and IQE of GaN ML QWs are discussed.
Another interesting feature of GaN-based QWs is optical anisotropy. As mentioned in Sect. 1, the optical polarization of AlGaN QWs switches from E ⊥ c to E ∣∣ c as the Al composition increases. Hence, light extraction along the [0001] direction becomes difficult in Al-rich AlGaN QWs. GaN QWs exclusive of Al from the well can intrinsically avoid this issue. In fact, Refs. 72 and 78 have experimentally demonstrated the dominant E ⊥ c emission from GaN ML thin superlattices below ∼240 nm. Strictly, because thin barriers (<∼3 nm <Bohr diameter) in their superlattices promote inter-well interactions of charged carriers, the electronic states of the superlattices differ from those of QWs with well-isolated wells. In addition, they measured the luminescence from the sample surface, which is suitable for characterizing emission patterns from devices but is not suitable for quantifying the polarization degree. This is because the refraction effect strongly limits the emission angle within the material, making it difficult to precisely estimate the contribution from the E ∣∣ c component. In Sect. 3.2, we directly examine the out-of-plane polarization properties of a 1-ML GaN/AlN single QW from the sample edge, which shows the promise of GaN/AlN ML QWs as (0001) surface UV emitters below 250 nm.
Since GaN has a bandgap of 3.4 eV (365 nm) at RT, GaN QWs must be very thin in the ML-level to reach a wavelength below 250 nm. Figure 6(a) shows the transition energy at RT calculated with the effective mass approximation. The results are consistent with those derived from the first principles calculations assuming 0 K (5.5 eV and 5.1 eV for 1-ML and 2-ML GaN QWs, respectively). 86) This is also supported by wavefunction calculations [Fig. 6(b)].
Fig. 6. (Color online) (a) Transition energy of GaN/AlN QWs at RT calculated with the effective mass approximation. (b) Calculated electron and hole wavefunctions of a 1-ML GaN QW in AlN shown in the upper image. Solid and dotted curves are based on first-principles calculations and the effective mass approximation, respectively.
Download figure:
Standard image High-resolution imageFigure 6(b) shows the squares of the electron and hole wavefunctions, and
in a 1-ML GaN QW in AlN. For the first-principles calculations, we assumed 5-ML AlN barriers. Similar wavefunctions derived from the first principles calculations have already been demonstrated for 1-ML GaN/AlN QWs.
81,89) The wavefunctions calculated within in the effective mass approximation (broken curves) well reproduce the envelopes of the wavefunctions by the first-principles calculations (solid curves). Figure 6(a) suggests that to reach 250 nm and below, the GaN well width must be thinner than 2-ML. It should be noted that the square overlap of the electron and hole wavefunctions calculated with the effective mass approximation is 0.7 for the 1-ML GaN/AlN QW and 0.2 for a conventional 1.5 nm thick Al0.75Ga0.25N/AlN QW emitting at a similar energy, suggesting an improved IQE in terms of radiative recombination.
To fabricate ML thin QWs, molecular beam epitaxy (MBE) is better suited than MOVPE because a reflection high-energy electron diffraction system can achieve in situ monitoring of the ML thickness. In fact, many of the reports on ultrathin GaN adopt MBE However, practical devices such as LEDs and LDs based on III-nitride semiconductors are mostly fabricated by MOVPE. Hence, establishing reproducible MOVPE growth of ML QWs is desirable.
Very recently, we found that appropriately adjusting the balance between crystallization and evaporation of Ga adatoms during MOVPE can realize self-limits of GaN thickness to 1-ML. 80) The balance can be controlled by the source precursors' ratio (V/III ratio). With this discovery, the luminescence peak position becomes nearly independent of the growth time, which means that a good reproducibility is achieved. Figure 7(a) shows a typical cross-sectional HRTEM image of a 1-ML GaN/AlN (0001) QW grown by MOVPE. Abrupt interfaces are confirmed. Figure 7(b) shows a PL spectrum of the 1-ML GaN QW at RT. The excitation laser was the fourth harmonics of a Ti:sapphire laser emitting at 212.5 nm. The emission peak is located at 5.36 eV (231 nm wavelength), which agrees well with the theoretical prediction in Fig. 6(a).
Fig. 7. (Color online) (a) Typical cross-sectional HRTEM image of a 1-ML GaN/AlN (0001) QW grown by MOVPE viewed along the [110] direction. (b) PL spectrum of the same QW at RT.
Download figure:
Standard image High-resolution image3.2. Optical properties of GaN/AlN ML QWs
The MOVPE procedures and sample structures are similar to those in Sect. 2.2, except for the substrates and QWs. In this section, nominally on-axis AlN or sapphire (0001) substrates are used. Furthermore, instead of AlGaN QWs, GaN single QWs are grown at 1150 °C with a V/III ratio of 109. To date, systematic differences due to substrates (sapphire or AlN) have not been observed. The reason of which is under investigation.
PL measurements were performed under essentially the same conditions as in Sect. 2.2. The temperature quenching of the integrated PL intensities of a 1-ML GaN/AlN QW and conventional 1.5 nm thick Al0.8Ga0.2N/AlN QW emitting at a similar wavelength are compared. The latter QW is the same as that in Fig. 4(b). The PL intensity at RT with respect to that at the lowest temperature increases from 0.1% for the conventional AlGaN QW to 5% for the 1-ML GaN/AlN QW, strongly suggesting an improved IQE.
The PL decay curves of these QWs are acquired at RT. The PL lifetime of the conventional AlGaN QW is 40 ps, while that of the 1-ML GaN/AlN QW becomes longer as 100 ps. To find the cause of this observation, the PL lifetime is decomposed into radiative and nonradiative lifetimes,
and
respectively. Assuming 100% IQE at the lowest temperature, which was 6 K in this study, the PL intensity at a certain temperature normalized by that at the lowest temperature can be regarded as IQE at a given temperature. The following general equations give
and
at a given temperature


Recently, the assumption of 100% IQE at low temperatures has been debated.
91–93) Because the precise IQE quantification is beyond the scope of this study, we discuss the carrier dynamics using the obtained and
as qualitative indices.
Table I summarizes the results. The faster radiative recombination lifetime for the 1-ML GaN/AlN (0001) QW is likely due to the increased overlap of electron and hole wavefunctions. The slower nonradiative lifetime for the 1-ML GaN suggests a decrease in nonradiative recombination centers. Both results are as expected in Sect. 3.1. The PL temperature quenching was analyzed in detail in comparison with the PL properties of AlN. 30,80) The results suggest a reduced density of cation vacancies, 30,80) supporting the assertion of the suppressed nonradiative recombination.
Table I. Carrier lifetimes at RT evaluated for a 1.5 nm thick Al0.8Ga0.2N/AlN QW, a 1-ML GaN/AlN (0001) QW, and a 2-ML GaN/AlN (102) QW.
Samples | Normalized RT-PL intensity (∼RT-IQE) |
![]() |
![]() |
![]() |
---|---|---|---|---|
1.5 nm Al0.8Ga0.2N/AlN QW | 0.1% | 40 | 40 | 40 |
1-ML GaN/AlN (0001) QW | 5% | 100 | 2 | 105 |
2-ML GaN/AlN (1![]() | 50% | 195 | 0.37 | 410 |
GaN-based QWs are expected to realize E ⊥ c polarization (i.e. TE polarization), as noted in Sect. 3.1, which is confirmed by the polarization PL measurements at RT. The examined samples are the same as those for the above PL measurements. The samples were excited at the surface normal by an ArF ns laser (193 nm) through a cylindrical lens, and the PL from the sample edge was detected by a CCD camera through a monochromator. A Glan–Thompson linear polarizer was placed within the optical path of the collimated PL. Prior to the measurements, the system polarization was calibrated using an unpolarized UV light source. The inset of Fig. 8 is a schematic of the experimental setup.
Fig. 8. (Color online) Polarization PL properties of the 1-ML GaN/AlN (0001) QW and the 1.5 nm thick AlGaN/ALN (0001) QW emitting at a similar wavelength of 230 nm. Inset shows that the samples are excited at the surface normal, and PL is detected from the sample edge through a Glan–Thompson polarizer. Horizontal axis represents the rotation angle of the polarizer.
Download figure:
Standard image High-resolution imageFigure 8 shows the results. The reference 1.5 nm thick AlGaN QW is nearly unpolarized but is slightly polarized along the c-axis (i.e. TM polarization). This is consistent with the previous study,
94) which demonstrated the difficulty in extracting the emitted light along the [0001] c-axis. By contrast, the 1-ML GaN/AlN (0001) QW shows strong TE polarization with an intensity ratio of 2/1. Although this value deviates from the previously reported values,
78) Fig. 8 confirms that a strong surface emission is possible below 250 nm using ML GaN QWs. The numerical deviation may be due to the different electronic structures between superlattices and QWs or due partially to the different measurement configurations.
3.3. Future prospects
To realize practical devices emitting below 250 nm with ultrathin GaN/AlN QWs, electron-beam pumping is one solution because high-energy electron beams can excite ultra-widegap materials with insufficient carriers for current injection. In fact, electron-beam-pumped spontaneous emission below 250 nm has already been demonstrated. 79,81) A more general device configuration is LEDs by electric injection, but doping difficulty into AlN-based materials is an obstacle for realizing high-performance devices. Although a few papers have reported LEDs using an AlN pn junction with ML GaN/AlN emitters below 250 nm, 72,83) thorough studies on novel device designs such as LEDs with AlGaN barriers 73) and novel doping techniques, including polarization doping, 75,76,78,84,95) will be necessary to boost the LED performance.
We have demonstrated that RT-IQE of ML GaN/AlN (0001) QWs is improved compared with conventional Al-rich AlGaN QWs. However, further improvements are expected. One clue can be found in Table I, which shows the radiative lifetime is relatively slow on the order of ns. Therefore, if similar ML GaN QWs can be fabricated on semipolar or nonpolar planes, IQE should be improved due to the suppressed piezoelectric and spontaneous polarizations.
We examined the growth on the (102) r-plane AlN substrates and found that the GaN thickness is self-limited to 1- or 2-ML, depending on the growth temperature (Tg
).
80) Figure 9 shows PL at 9.5 K from 1-ML and 2-ML GaN/AlN QWs on (1
02) r-plane AlN. Emission below 250 nm is achieved. (The emission wavelength of a 2-ML GaN QW on (1
02) is ∼250 nm at RT.
80)) Because 1-ML of the (1
02) plane is thinner than that of the (0001) plane, the PL of (1
02) GaN QWs shifts to shorter wavelengths compared with (0001) GaN QWs. The temperature dependence of PL from the 2-ML GaN/AlN QW on (1
02) was examined under the same condition as Fig. 4. (The 1-ML GaN/AlN QW on (1
02) cannot be excited by our ps-pulse laser.) The PL intensity at RT is as high as ∼50% of that at a low temperature of 6 K, which suggests drastic improvement of IQE, compared with those of the 1-ML GaN/AlN QWs on (0001) or conventional AlGaN QWs.
Fig. 9. (Color online) PL spectra of 1-ML or 2-ML GaN/AlN QWs on (102) at 9.5 K. Excitation laser is ArF because the 1-ML QW cannot be excited by our ps-pulse Ti:sapphire laser.
Download figure:
Standard image High-resolution imageThe carrier dynamics was investigated for the 2-ML GaN (102) QW using the same ps-pulse laser. Figure 10 shows the temperature dependence of
Using Eqs. (1) and (2),
and
at RT are evaluated and are listed in Table I. Figure 10 and Table I suggest a faster radiative recombination in GaN/AlN QWs on (1
02) than on (0001). Additionally, it is noteworthy that
becomes longer as the temperature approaches RT (Fig. 10). This suggests that even at RT,
is strongly affected by
and is consistent with the drastically improved IQE. Table I confirms this argument. Further investigations on, for example, the growth conditions and crystal planes will provide higher IQEs.
Fig. 10. (Color online) Temperature dependence of of a 2-ML GaN/AlN (1
02) QW.
Download figure:
Standard image High-resolution imageFinally, we would like to comment on the excitonic properties. Some theoretical works predict high exciton binding energies in ML GaN/AlN QWs on (0001). 74,89) The predicted binding energies are insensitive to the GaN QW widths but are sensitive to the AlN barrier widths. For example, the binding energies of 1-ML GaN QWs are ∼100 meV for the 2-ML AlN barrier and ∼200 meV for the 8-ML barrier. The binding energy decreases for a thinner barrier because thinner barriers promote inter-well interactions, and the materials can be regarded as alloys without strong carrier confinement. Irrespective of the barrier width, excitons have sufficient binding energies to be stabilized even at RT. Due to exciton formation, a higher radiative recombination probability is expected, especially under weak excitation conditions. Bayerl et al. predicted radiative lifetimes of excitons in ML GaN/AlN (0001) QWs on the order of ns, which are faster than those of free carriers. 89) The predicted radiative lifetimes agree well with our analyses in Table I, suggesting that excitons contribute to the improved IQE. Elucidating exciton-related phenomena will be interesting both in science and engineering.
4. Conclusions
Here, we discuss two emerging structures based on III-nitride semiconductors for next-generation light sources emitting in the far UVC range below a wavelength of ∼250 nm. One is AlGaN QWs on macrosteps. This structure effectively suppresses nonradiative recombination and improves IQE. The other is GaN QWs with ML thin wells. ML QWs enhance radiative recombination due to a strong carrier confinement and suppress nonradiative recombination most likely by suppressing cation vacancy formation. Although AlGaN-based UV LEDs suffer from low emission efficiencies at short wavelengths, such novel structures can pave the way toward an efficiency boost in the far UVC region.
Acknowledgments
The authors acknowledge Y. Hayashi, S. Ichikawa, M. Hayakawa, H. Kobayashi, and M. Morioka for their help with the experimental and theoretical works. This work was partially supported by JSPS KAKENHI (Grant Nos. JP15H05732, JP16H02332, JP16H06426, JP20H05622, and JP21H04661).
Biographies
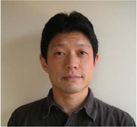
Mitsuru Funanto is an Associate Professor of Electronic Science and Engineering, Kyoto University since 2007. He received his B.Sc., M.Sc., and D.Sc. degrees in Electronic Science and Engineering, Kyoto University, Kyoto, Japan, in 1989, 1991, and 1998, respectively. In 1991, he joined Kyoto University, Kyoto, Japan as a Research Associate in the Department of Electrical Engineering. In 2002, he became a Lecturer and was promoted to an Associate Professor in the Department of Electronic Science and Engineering in 2007. During 2000–2001, he was a Visiting Research Fellow in the Department of Physics, Heriot-Watt University, the United Kingdom. His current research interests include crystal growth, characterization, and device applications of III-nitride semiconductors.
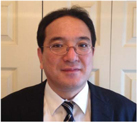
Yoichi Kawakami is a Professor of Electronic Science and Engineering, Kyoto University since 2007. He received his B.Sc., M.Sc., and D.Sc. degrees in Electrical Engineering from Osaka University, Osaka, Japan, in 1984, 1986, and 1989, respectively. In 1989, he joined Kyoto University, Kyoto, Japan as a Research Associate in the Department of Electrical Engineering. He became an Associate Professor in 1997, and was promoted to a Professor in the Department of Electronic Science and Engineering in 2007, and was engaged in research on widegap semiconductors. During 1991–1992, he was a Visiting Research Fellow in the Department of Physics, Heriot-Watt University, the United Kingdom. He was awarded the JSAP fellow from The Japan Society of Applied Physics in 2011. His current research interests include spatial and temporal luminescence spectroscopy of excitons in GaN-based low-dimensional semiconductors, fabrication of new photonic devices, and applications of solid-state lighting using white light-emitting diodes.