Abstract
On 14 September 2015, the two detectors of the Laser Interferometer Gravitational-wave Observatory (LIGO) in the US recorded the first direct detection of gravitational waves. This paper reviews the contributions to this discovery by V B Braginsky's group at the Physics Department of Lomonosov Moscow State University.
Export citation and abstract BibTeX RIS
1. Introduction
On 14 September at 5:51 Eastern Standard Time (EST) [13:51 Moscow time (MSC)], the twin detectors of Advanced LIGO (Laser Interferometer Gravitational-Wave Observatory) in Livingston (Louisiana, USA) and Hanford (Washington, USA) detected a gravitational signal. An article regarding this event [1] was published on behalf of the LIGO Scientific Collaboration and Virgo Collaboration communities.
The analysis of the signal shape has shown that the source of the gravitational wave was a merger of two black holes with masses of about and
(
is the solar mass), which took place at a distance of approximately 1.3 billion light years. During this event, the energy
(!) was emitted as gravitational radiation in fractions of a second. At the final stage, the distance between the black holes became of the order of the Schwarzschild radius,
(
is the gravitational constant,
is the total mass of the merging black holes, and
is the speed of light); the relative speed of the black holes before the merger was about half the speed of light.
The magnitude of the gravitational signal is quite large (corresponding to ). Moreover, the detection was performed independently by two detectors located
apart (using a coincidence circuit). Both these facts prove that for the first time gravitational waves were observed directly.
We recall that in 1993 J Taylor and R Hulse received the Nobel Prize in physics for the indirect discovery of gravitational waves through the observation of the change in the period of the binary pulsar PSR . According to the General Theory of Relativity, the emission of gravitational waves by a binary star can lead to rotational energy loss and hence to an increase in the rotation frequency; the observed frequency change coincided with theoretical predictions [2].
The LIGO program was started in 1992 as a scientific megaproject (funded by the National Science Foundation (NSF), USA). A scientific group under the direction of V B Braginsky at the Physics Department of Moscow State University (MSU) has been working in the field of gravitational physics since the 1970s, and at the beginning of the 1990s it started the research on gravitational-wave laser antennae. Below, we describe the main aspects of this research.
2. Experimental investigation of mirror suspension noises
The suspension for test masses at the Advanced LIGO (ALIGO) gravitational-wave detector is a complicated four-stage structure that allows suppressing the seismic influence on test masses and controlling their position in order to maintain the interferometer adjustment. The main contribution to the thermal noise is made by the first stage, where the test mass (interferometer mirror) is suspended as a pendulum with an angular oscillation eigenfrequency
and a mechanical loss angle
, where
is the quality factor of the pendulum oscillation mode. The power spectral density of thermal noise of a test-mass displacement at a frequency
has the form [3]

where is the Boltzmann constant and
is the absolute temperature.
The operational frequency band of the gravitational-wave detector is , which is significantly higher than the resonance frequency of the oscillation mode (around
). At these frequencies, test masses can be considered to be free and the spectral density of thermal noise is proportional to the loss angle
. Reducing the losses is the key factor that leads to the thermal noise suppression. Mechanical losses
of the pendulum mode are determined by losses in the pendulum suspension wires. We note that these losses are much smaller than those in the material of the wires. This is because the pendulum energy is mainly stored as a nondissipative energy of the gravitational field, and only a small part is stored as the elastic deformation energy [4]. Other suspension modes, such as string, longitudinal, etc., also make a contribution to the test-mass thermal noise depending on the degree of their coupling to the horizontal movement of test masses.
In first-generation detectors (Initial LIGO), test masses fabricated from high-purity fused silica were suspended on steel wires. Such suspensions did not provide minimal energy losses for the eigenmodes of test-mass elastic oscillations and center-of-mass oscillations. Moreover, they demonstrated highly undesirable additional excess noise.
Braginsky's group developed a monolithic suspension with both the test mass and wires fabricated from fused silica. The wires were drawn in the flame of an oxygen burner [5]. Further investigations led to an improvement in the suspensions, and it was experimentally shown that the damping time of silica test mass pendulum oscillations is approximately five years, which corresponds to [6, 7]. Measured
-factors of the string oscillation modes of silica suspensions were also higher than
[8, 9]. This resulted in the development of a quasi-monolithic silica test-mass suspension for the ALIGO detectors [10]. Currently, the group is carrying out research on suspensions for silicon test masses [11], which will be used in the next-generation cryogenic gravitational-wave detector LIGO Voyager [12].
3. Experimental investigation of electric charges on mirrors
Another problem is associated with electric charges, which are always present on silica test masses. Their sources are any contacts of the test mass with other objects, including limiters of motions caused by earthquakes, gas desorption processes, etc. Important fundamental sources of electric charges are cosmic rays [13, 14]. The charges interact with the bodies and electric fields that surround the test mass, creating an additional fluctuating force acting on the test mass. To lower the noises caused by electrostatic charges, we need to decrease their number and increase the relaxation time of charge distribution.
Researchers at the MSU Physics Department performed detailed experimental research on the processes of electric charge migration over the surface of fused silica samples and the interaction of charges with surrounding bodies and fields, including the electric field of the electrostatic actuator used for the adjustment of the test mass (interferometer mirrors) position. It was reported that the relaxation time of an electric charge placed on the test mass reaches three years [15, 16]. For such long relaxation times, the charge noise at LIGO can be significantly lowered by using the electric charge neutralization technique [12].
4. Experimental investigation of excess noise
Excess (nonthermal) mechanical noises could be a serious problem in the development of gravitational wave detectors. The detector response to the action of a gravitational wave is a small change in the distance between the test masses, and this is a very infrequent event. At the same time, structural changes (dislocation motion, microcrack formation and evolution, impurity diffusion, etc.) in the test mass and suspension materials can lead to the emission of stored energy and excitation of oscillations that imitate the response to a gravitational wave. One such effect is acoustic emission, which is usually observed in strained constructions made of metals and alloys. Critical elements are the suspensions, which in the first-generation detectors, as was mentioned before, were constructed of steel wires and in modern detectors are silica wires. For a
load, the elastic energy stored in the wire is more than 20 orders of magnitude larger than the energy that corresponds to the response to a gravitational wave.
A series of experiments were conducted in 1995–1998 in order to find the excess noise in the suspension prototypes. First, the excess noise was found in tungsten wires [17] and then in steel wire samples provided by the LIGO laboratory [18]. The measurements were performed using a Michelson interferometer with the surface of the investigated samples (wires) acting as mirrors. The setup was measuring the amplitude fluctuations of thermal oscillations in the fundamental string mode. Because the wire mass is much smaller than the supposed load mass, the requirements for the setup sensitivity were much lower than for the detectors themselves. The obtained results were used in the first-generation LIGO detectors: the diameter of the suspension wires was increased, which weakened (but did not eliminate) this effect.
After that, our laboratory investigated silica wires. We used an original monolithic silica construction with a miniature mirror and a Fabry–Perot interferometer. The mechanical -factor of the investigated string modes turned out to be
and the sensitivity was
. The excess noise was not observed above the level of 1% of equilibrium thermal oscillations, which led to the conclusion that this effect would not occur in second-generation gravitational-wave detectors with a silica wire suspension [19].
5. Calculation of thermal noise in mirrors
The light beam multiply reflects in the arms of the LIGO interferometer and acquires a phase proportional to , where
is the dimensionless additive variation of the metric (caused by the gravitational wave) and
is the arm length. Therefore, thermal fluctuations of the mirror surfaces from which the light reflects inside the arms mask the desired signal and cause an inevitable error in the measurements. One of the main factors that limit the ALIGO sensitivity is the thermal fluctuations of the mirror surface averaged over the power profile of the incident beam.
We recall that the fluctuation–dissipation theorem states that the spectral density of thermal noise of some generalized coordinate at a frequency is determined by the damping at this frequency, or rather the imaginary part of the permittivity [20, 21].
Historically, the first thermal noise of the mirror surface to be calculated was the one caused by losses in the material of the mirrors [21, 22]. In particular, the spectral density of surface oscillations averaged over the power profile of the laser beam
with the effective radius
(
is the radial coordinate) is expressed as

where (rad
) is the spectral frequency,
and
are Young's modulus and the Poisson coefficient, and
is the loss angle, which is formally defined as an imaginary addition to Young's modulus,
. We note that in the currently accepted model of so-called structural damping [3], the loss angle
is independent of the frequency
(on the contrary, in the model of viscous damping,
formally depends on the frequency).
Currently, the test masses are fabricated from high-quality fused silica. In the early 2000s, when discussing next-generation detectors, it was suggested to make test masses of artificial sapphire, which has a slightly lower loss angle. We demonstrated that besides structural damping, there is also thermoelastic damping, which turned out to be larger in sapphire [23].
The physical reasons for thermoelastic damping are the fundamental temperature fluctuations, which result in additional mirror surface fluctuations due to thermal expansion. The corresponding spectral density was obtained in a simple model where the mirror is replaced with a semi-infinite space [23],

where is the thermal expansion coefficient,
is the heat capacity,
is the thermal conductivity coefficient, and
is the density (of the material of the mirror).
Expression (3) can be derived from semiclassical considerations (Fig. 1), when surface fluctuations are averaged over the spot with a radius that is much larger than the characteristic thermal diffusion length
at the frequency
,

In the case of the ALIGO detector, the characteristic frequency is , with
. In fused silica,
and the inequality
confidently holds. We can calculate thermodynamic fluctuations of the temperature in any small volume
and the corresponding size fluctuations due to thermal expansion,
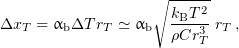
where is the thermal expansion coefficient of a small volume. Thermal fluctuations in each volume can be considered independent of each other and the number of these volumes can be estimated as
. Thus, the fluctuations of the spot displacement
, averaged over the spot with a radius
, can be estimated as the sum of independently fluctuating volumes:

Comparing (3) and (5), we see that the relation is satisfied up to a factor of the order of unity if we assume that
. Therefore, our semi-qualitative consideration is justified.
Figure 1. Qualitative explanation of thermoelastic damping. Mirror surface fluctuations are averaged over the Gaussian profile of the incident beam power with the width . These fluctuations are caused by independent temperature fluctuations in small volumes with the size of the order of the thermal diffusion length
at a frequency
.
Download figure:
Standard imageActually, the number of thermal noises of different natures is quite large: one can even speak of a 'noisy zoo' [24]. Figure 2 shows some of them. Currently, the main contribution is made by the Brownian damping in the interference coating of the mirrors [25, 26].
Figure 2. Contribution of different noises in ALIGO mirrors, recalculated as the square root of the power spectral density of the mirror fluctuation displacement.
Download figure:
Standard imageThe same thermodynamic temperature noises cause fluctuations of the reflection coating refractive index, so-called thermorefractive noises (TR noises) [27], which were for the first time experimentally observed in silica microresonators of whispering gallery modes [28].
Because the source of thermoelastic (TE) and thermorefractive (TR) noises is the same (thermodynamic fluctuations of the temperature), they can partially compensate each other, as was shown in [29, 30].
Thermal noises of different natures were also calculated in a multilayer reflection coating [20, 31, 32], diffraction grating used as a mirror [33], and the deflection mirror in the GEO 600 experiment [34] (the distribution of incident power on it has the form of interference fringes).
In order to avoid or decrease fluctuations in the reflective coating, it was suggested to use corner reflectors instead of mirrors [35] and double mirrors (Khalili etalon) [36–38].
Additionally, calculations were performed for noises caused by cosmic ray showers [13, 14] and photoelastic (PTE) noises (volume fluctuations causing refractive index fluctuations) [39] and spontaneous crystallization noises [40] in fused silica. It was shown that all these noises make a negligible contribution to the net noise at ALIGO.
6. Parametric oscillatory instability
Large powers of light that should circulate in the arms of the laser interference detector ( are planned for ALIGO) can lead to the appearance of unwanted nonlinear effects, including parametric oscillatory instability (POI) [41]. POI corresponds to the energy transfer from the fundamental pumped mode of a Fabry–Perot resonator with a frequency
into the optical Stokes mode with a frequency
(not pumped) and the elastic oscillation mode in one of the mirrors with a frequency
under the frequency matching condition

The mechanism of such a process is obvious. On the one hand, small oscillations in the Stokes optical mode give rise to a ponderomotive force that acts on the mirror at the difference frequency , which resonantly pumps the mechanical oscillations. On the other hand, small mechanical oscillations of the mirror due to the Doppler effect lead to the generation of waves after reflection from the mirror with the frequencies
, one of which,
, resonantly excites oscillations in the optical Stokes mode. As the power of the pump at the frequency
increases, this mechanisms leads to larger energy transfer. According to the Manley–Rowe relations, the energy is transferred from the pump wave to the optical Stokes and mechanical modes. This effect of POI can be regarded as an instance of negative damping.
POI can be observed in a Fabry–Perot resonator if the circulating power reaches the threshold and the condition [41]

holds, where


and
are the Stokes optical and elastic mode damping coefficients,
is the distance between the Fabry–Perot resonator mirrors,
is the mirror mass,
is the mismatch,
is the overlap coefficient for the distributions of the fundamental Stokes optical and elastic modes,
and
are the light field distribution functions over the beam cross section for fundamental and Stokes modes,
is the component of the elastic mode displacement vector
normal to the surface of the cylindrical mirror base,
corresponds to the integration over the mirror surface, and
corresponds to the integration over the mirror volume
.
For a given pump frequency , we should analyze all suitable combinations of Stokes and elastic modes that satisfy condition (6) and verify that condition (7) holds for them. The number of such pairs can be relatively large. Also, in the case of a Fabry–Perot resonator with spherical mirrors, the eigenfrequencies and optical mode distributions can be calculated analytically, while the elastic modes of the mirrors have to be calculated numerically. Fortunately, in a resonator with finite-size mirrors, the diffractive losses of optical modes increase with the mode number (this corresponds to the increase in the optical damping
), and therefore the number of modes that has to be calculated is limited (around 40). We note that the frequencies
of the Fabry–Perot mirror elastic modes are comparable by the order of magnitude with the mode spacing
in the Fabry–Perot interferometers located in the ALIGO arms, which makes it easier to satisfy condition (6).
POI was additionally analyzed in ALIGO with a power recycling mirror [42] and a signal recycling mirror [43] in the case of a small difference in arm lengths [44] in the GEO 600 laser detector [45]. A more detailed analysis with a numerical calculation of mirror elastic modes [46] was performed using the Laguerre–Gauss mode as the fundamental one (this was suggested as a method for thermal noise suppression) [47].
As one of the methods for suppressing the POI, the use of resonators with a sparse spectrum was proposed; in these 'single mode' resonators [48, 49], the number of possible optical Stokes modes is decreased.
The processes of POI development were calculated so as to allow the POI suppression operations to be triggered based on precursors. POI was experimentally observed in the model of a Fabry–Perot resonator with a membrane [52] and in the full-scale ALIGO interferometer [53].
A detailed review of POI in laser gravitational detectors can be found in [54, 55].
7. Quantum measurements
In the Initial LIGO, gravitational waves were detected by the displacement of the interferometer end mirrors playing the role of test masses, with an accuracy of about (for the average frequency and the frequency band around
). Currently, the ALIGO accuracy is of the order of
; the planned accuracy is
.
We note that the measurement of such small displacements, , was already experimentally demonstrated in the 1980s [56] using the superconducting cavity sensor, but for a long measurement time
and a small gap
. The key difference of the displacement measurements at LIGO is in the short measurement time (
instead of
) and the huge distance between the mirrors (
instead of
). A record small error of relative displacement will be achieved in ALIGO:
.
It was already clear at the LIGO planning stage that such a small error of the displacement measurement should lead to the appearance of quantum limitations. It is known that in continuous measurements of a coordinate, the standard quantum limit (SQL), first predicted by Braginsky [57], manifests itself.
To clarify the SQL concept, we consider a particular case: a free mass is influenced by a force
, which has to be registered by measuring the coordinate twice after the time interval
. By performing the first measurement of the coordinate with an accuracy
, we inevitably impart a random momentum
to the mass, in accordance with the Heisenberg uncertainty principle. Measuring the coordinate for the second time with an accuracy
, we obtain the error of the coordinate difference
:

We can make arbitrarily small, but there is an optimal value of
for which the error
is minimal (this is the SQL):

The reason for the SQL is the inevitable introduction of fluctuation back reaction by the coordinate measurement (in our example, it is ) as a consequence of the Heisenberg uncertainty principle.
This reasoning can be easily generalized by considering a continuous measurement of the coordinate as a sequence of instantaneous measurements [58, 59].
In the Initial LIGO, the sensitivity to small displacements was almost lower than the SQL, but the planned sensitivity of ALIGO is of the order of the SQL and can possibly overcome it.
It is known that the SQL can be surpassed by changing the measuring procedure. Such procedures were called quantum nondemolition measurements (QNDs) [58, 60, 61]. Braginsky's group was the first to describe QND measurements of the oscillator energy [62] and stroboscopic measurement of the oscillator coordinate [63, 64] (in fact, these were the first examples of QND measurements).
One of the most interesting examples is the proposal of a quantum speed meter, which is the velocity measurement [65–67] in laser gravitational detectors. Basically, the proposal was to measure not the coordinate but the coordinate difference , which for a small time delay
actually corresponds to the velocity measurement. We recall that for a free mass, the momentum is a QND variable, not subject to fluctuation back-reaction noises, and therefore velocity measurement allows overcoming the SQL.
Another proposal was to perform a variation measurement. The idea was to use the output laser beam carrying information about the mirror displacement and measure the special quadrature component in which the information about fluctuation back reaction is absent (is subtracted) [68–71]. We note that formally, the variation measurement is not a QND measurement because there is no corresponding QND variable.
Another method to overcome the SQL involves using optical rigidity [72–76]. Introducing optical rigidity allows transforming the test mass into an oscillator, for which the SQL is lower, while the noises remain at the same level (we note that the introduction of mechanical rigidity is usually followed by additional noises).
A detailed review of the prospects of quantum measurement applications in laser gravitational-wave detectors can be found in [59].
8. Conclusions
The research performed by the Braginsky group at the MSU Physics Department has made a significant contribution to the development and fabrication of LIGO gravitational-wave detectors. Currently, the group continues its research on the improvement of the existing detectors and the development of elements for future gravitational-wave antennae. Importantly, many of the results mentioned have an independent significance for the development of the theory and methods of precise physical measurements.
The authors are deeply grateful to all PhD and master students at the MSU Physics Department who participated in the work of the scientific group, to mechanics V K Apal'kov, Yu N Kiselev, and A A Khorev, who worked on the assembly of unique experimental setups, and to our colleagues who took part in the discussions of the results.
The work of the group was funded by the Ministry of Education and Science of the Russian Federation, the NSF USA, the Dynasty Foundation, and others. Currently, the work is funded by grants PHY-130586 (NSF USA) and 14-02-00399A (RFBR).