Abstract
Review of the literature on the currently recognized, thirteen vitamins yields an overview of the electrochemical properties that include estimates of the formal potentials at physiological pH and identification of the general classes of redox mechanisms. All vitamins are electroactive and map a range of formal potentials over a 3 V window. The vitamins are grouped as lipid soluble (vitamins A, D, E, and K) and water soluble (B vitamins and vitamin C). Mechanisms are grouped as single electron transfer agents (B3, B7, B2, C, and D), vitamins that can be both oxidized and reduced (B1, B5, B6, B9, and E), and vitamins that undergo two successive, distinct reductions (B12 and K). Vitamin A voltammetry is uniquely complex. Plot of the formal potentials on a potential axis allows assessment of mechanistic paths to vitamin recycling, antioxidant behavior, pH dependence, electrochemical stability in air, acid, and water, electrochemical instability of vitamin pairs, and cooperative interactions between vitamins in medicine. The potential axis is shown as an effective tool for mapping thermodynamically complex interactions. The voltammetry literature for each vitamin is critically assessed.
Export citation and abstract BibTeX RIS

This is an open access article distributed under the terms of the Creative Commons Attribution 4.0 License (CC BY, http://creativecommons.org/licenses/by/4.0/), which permits unrestricted reuse of the work in any medium, provided the original work is properly cited.
The National Institutes of Health, Office of Dietary Supplements defines vitamin as A nutrient that the body needs in small amounts to function and maintain health.1 Merriam Webster Dictionary2 defines vitamin as:
any of various organic substances that are essential in minute quantities to the nutrition of most animals and some plants, act especially as coenzymes and precursors of coenzymes in the regulation of metabolic processes but do not provide energy or serve as building units, and are present in natural foodstuffs or sometimes produced within the body.
By modern standards, the US federal government identifies 13 vitamins.3 The water soluble vitamins are vitamin C and the eight B vitamins (B1, B2, B3, B5, B6, B7, B9, B12). The lipid or fat soluble vitamins are A, D, E, and K. The fat soluble vitamins can accumulate in the body whereas the water soluble vitamins do not. There are no current vitamins designated beyond E except for K because materials historically assigned the interposed letter designations either no longer fall under the modern definition of vitamin or several related materials were reclassified. Several vitamins exist in different but related chemical structures.
Questions considered in this perspective include whether all vitamins are electroactive; what is the redox potential of the vitamins; what are the kinetics and mechanisms of vitamins on oxidation and reduction; when are vitamins antioxidants; are vitamins stable to water and oxygen; do vitamins interact cooperatively. Although papers are available on the electrochemistry and voltammetry of individual vitamins, no single resource summarizes the electrochemical data for all vitamins. This review compiles and critically assesses the available literature on vitamin voltammetry. The compiled data serve to develop perspective on the electrochemical properties of vitamins and the role of vitamins individually and collectively as electroactive species. This CRES3T review assimilates fundamentals of thermodynamics and estimated formal potentials, kinetics, and mechanisms as assessed voltammetrically for individual vitamins to provide perspective on the collective electrochemical properties of the thirteen vitamins. Perspective on vitamin electrochemical properties may contribute to assess yet more complex bioelectrochemical processes.
The review contains two main sections. One section compiles the data for the vitamins and provides perspective on the electrochemical behavior and properties. The second section summarizes the available literature for each vitamin where papers judged the best available are included. In the first section, some compilation of the electrochemical and voltammetric properties of vitamins are summarized in Table I. The order of the reduction potentials, as approximated from voltammetric literature data at pH 7, is mapped in Figure 1. The notations for kinetics and potentials embedded in Table I and Figure 1 as well as a brief discussion of how redox potentials are estimated are provided.4 A list of abbreviations is provided at the end of the document in Table AI.
Table I. Table of Electrochemical Properties of 13 Vitamins.
Vitamin | Estimated E1/2 (vs NHE)a | pH/aprotic | E1/2 from | pH dependent | ≈ mechanismb |
---|---|---|---|---|---|
C | 0.39 V C|Cox | 7.4 | Ep | ✓ | ![]() |
ascorbate | |||||
![]() |
![]() |
![]() |
![]() |
![]() |
![]() |
![]() |
![]() |
![]() |
![]() |
![]() |
![]() |
![]() |
![]() |
![]() |
![]() |
![]() |
![]() |
![]() |
![]() |
![]() |
![]() |
![]() |
![]() |
![]() |
![]() |
![]() |
![]() |
![]() |
![]() |
5 | |||||
![]() |
![]() |
![]() |
![]() |
![]() |
![]() |
![]() |
![]() |
![]() |
![]() |
![]() |
![]() |
![]() |
![]() |
![]() |
![]() |
![]() |
![]() |
![]() |
![]() |
![]() |
![]() |
![]() |
![]() |
![]() |
![]() |
![]() |
![]() |
![]() |
![]() |
![]() |
![]() |
![]() |
![]() |
![]() |
|
![]() |
![]() |
![]() |
![]() |
![]() |
![]() |
aUnderscore indicates the native state vitamin in solution. Charge in the native state is noted in the Synopsis for the individual vitamin.
bApproximate mechanism denoted in electron transfer steps () and chemical steps (
).
cPCET is proton coupled electron transfer (e.g.,
).
Figure 1. The potential axis for the thirteen vitamins drawn by the IUPAC convention of positive (oxidation) on the right. For each redox process, O + ne R, the reaction is denoted R|O. Vitamins in the native, unreacted state are denoted in boldface. The lipid soluble vitamins are shown in red and the water soluble in shades of blue to green. For vitamin A, retinol (A) and retinal (A') are shown. All vitamin potentials are at pH 7 unless indicated by a subscript for E1/2. As estimated, the potential E1/2 approximates the formal potential E'0 to within about 100 mV. The lower potential axis illustrates the other species such as the thermodynamic water potential window at pH 7 and other chemical species such as peroxide. All potentials are reported versus NHE.
Table AI. List of Symbols and Abbreviations.
F | Faraday constant, 96485 C/mol |
R | Gas constant, 8.31447 J/(mol K) |
T | Temperature (K) |
F/RT | 38.92 V− 1 at 298.16 K |
E0 | Standard potential (V vs NHE) |
E0' | Formal potential (V) |
E1/2 | Half wave potential (V), ![]() |
Ep1/2 | Measured potential at half maximum current in polarography |
Ep | Peak potential (V) |
Ep/2 | Potential at half peak current (V) |
ΔEp | Peak potential splitting ΔEp = |Ecp − Eap| |
Eave | Average peak potential ![]() |
ip | Maximum magnitude current (A) |
Dj | Diffusion coefficient of species j (cm2/s) |
cj* | Bulk concentration of species j (mol/cm3) |
CV | Cyclic voltammetry |
LSV | Linear sweep voltammetry |
DPV | Differential pulse voltammetry |
RDV | Rotating disk voltammetry |
SWV | Square wave voltammetry |
DPP | Differential pulse polarography |
CPE | Controlled potential electrolysis |
NHE | Normal hydrogen electrode, reference electrodea |
SHE | Standard hydrogen electrode, reference electrode |
SCE | Saturated calomel electrode, reference electrodea ENHE = ESCE + 0.2412 V |
Ag|AgCl | Silver silver chloride reference electrodea ENHE = EAg|AgCl + 0.197 V |
Fc|Fc+ | Ferrocene ferrocenium reference electrodea for nonaqueous electrolytes ![]() |
GCE | Glassy carbon electrode |
HMDE | Hanging mercury drop electrode |
DME | Dropping mercury electrode |
BDD | Boron doped diamond electrode |
CPE | Carbon paste electrode |
MeCN | Acetonitrile CH3CN |
DMF | Dimethylformamide |
THF | Tetrahydrofuran |
NMP | n-Methyl-2-pyrrolidone |
DMSO | Dimethyl sulfoxide |
TBABF4 | Tetrabutylammonium tetrafluoroborate, Bu4BF4 |
nBu4NPF6 | Tetrabutylammonium hexafluorophosphate |
α-TOH | α-Tocopherol |
IL | Ionic Liquid |
E, C | Mechanistic designations for electron transfer (E) and chemical steps (C) |
PCET | Proton coupled electron transfer (e.g., ![]() |
k0 | Standard heterogeneous electron transfer rate (cm/s) |
α | Transfer coefficient |
ki | chemical reaction rate |
log P | log P Partition coefficient P is the ratio of solute concentrations in immiscible octanol and water |
Kinetic notation
Much of the data evaluated in this review is drawn from voltammetry. Voltammetry drives electron transfer events, generically represented as , where formal potential E0' characterizes the energy of the process. Voltammetric morphology can be impacted by a wide variety of experimental conditions that include solvent, solution components, and pH as well as chemical processes. Kinetics are captured in abbreviations of
and
, that denote mechanistic steps of interfacial (heterogeneous) electron transfer and homogeneous (bulk phase) chemical reactions.
characterizes an electron transfer process at the electrode. If the electron transfer is fast compared to the rate of voltammetric perturbation (e.g., cyclic voltammetric scan rate v), then the process is said to be reversible and
is assigned to the process. Absent chemical reactions,
characterizes a Nernstian process. If the electron transfer is slow compared to the voltammetric perturbation, the irreversible electron transfer is designated
. When rates of electron transfer and perturbation are comparable, the reaction is quasireversible,
. When there are no associated chemical reactions, designation with only
is appropriate and reversibility of the electron transfer is usually readily determined.
When chemical steps couple with the electron transfer, the designation is . The chemical step can precede the electron transfer (
) or follow the electron transfer (
). For more than one electron transfer, designations include
,
, and
. When there is more than one electron transfer, disproportionation may occur. Chemical steps may be further characterized as irreversible, reversible, catalytic, and dimerizations. To identify the nature of the chemical step commonly requires extensive chemical and electrochemical characterization. For this review, a simple label of
is typically used. When the chemical process is chemically irreversible,
is denoted.
Reaction sequences that include both redox potential and acid base dissociation reactions are commonly represented as square schemes. Laviron provides a well developed example for quinones that are set by two electron transfers characterized by six formal potentials and six protonations characterized by pKa values.7 The overall mechanism for quinone electrochemistry involves nine species, as shown by a square scheme in Scheme 1. A square scheme maps various paths between oxidized and reduced species. Dependent on pH and pKa values, different degrees of protonation are set for the species and
is the formal potential established for each redox pair. Vitamin K is a quinone and vitamin E is structurally similar to a quinone. Vitamin K has two sequential reduction steps in an
mechanism whereas the formal potentials for vitamin E allow disproportionation; both fall within a square scheme.
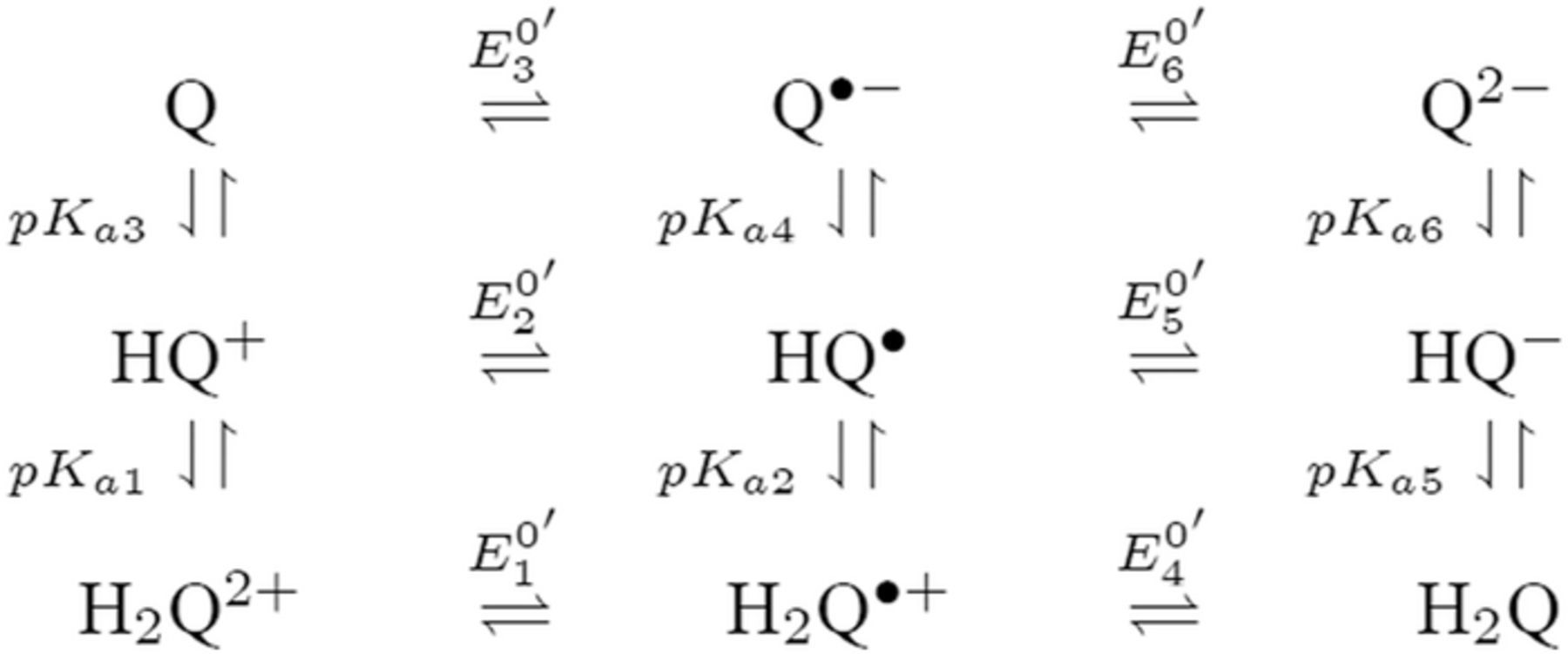
Scheme 1. An example square scheme is drawn from Laviron7 for pH and formal potential dependent pathways between benzoquinone Q and hydroquinone H2Q as a two electron, two proton reaction, . Laviron identifies values for acid dissociation constants (pKa2 ≈ −1; pKa3 ≃ −7; pKa4 = 4.1 ; pKa5 = 9.85; and pKa6 = 11.4) and formal potentials (E0'2 = 0.765; E0'3 = 0.099; E0'4 = 1.101; E0'5 = 0.459; and E0'6 = 0.027 V vs SHE (standard hydrogen electrode), where proton activity is unity). Mechanistic paths between benzoquinone and hydroquinone can trace down various paths dependent on the experimental conditions. Voltammetric morphology reflects the mechanistic path.
Potential and estimates of formal potential
There are numerous expressions of electrochemical potential.4 Several are important to estimates of the formal potential from voltammetry. Standard potential E0 is a thermodynamic value that characterizes a half reaction written as a reduction for a species relative to a reference electrode where all species are present under standard conditions of unit activities. Tabulated E0 values are typically reported relative to the normal hydrogen electrode, NHE, which is by definition 0.000 V for
at unit concentration. Formal potential E0' is a measured potential relative to NHE for half reaction
where the ratio of product to reactant concentrations, cvrprod/cvoreact, is unity or concentrations for all species in the system are fixed and reported. Activity coefficients and equilibrium constants can be embedded in the formal potential. For example, standard potential requires pH = 0, which would destroy many biological materials. A formal potential can be reported under conditions of physiological pH. Formal potential embeds thermodynamic information of standard potential and activity under formally specified conditions.
Various diagnostic potentials are measured voltammetrically. For this review, measured potentials are used to estimate , which can be drawn from half wave potential E1/2
![Equation ([1])](https://content.cld.iop.org/journals/1945-7111/165/2/G18/revision1/d0001.gif)
where DR and DO are the diffusion coefficients for the reduced and oxidized halves of the couple. Typically, DR and DO are comparable and E1/2 well approximates E0'. In cyclic voltammetry undertaken at planar electrodes, peak potential Ep measured at the largest magnitude peak currents |ip| estimates or E1/2. For simple
, the average of the anodic and cathodic Ep values, Eave yields
.
One objective of this review is to estimate the reduction potential characteristic of each vitamin through measures of either E1/2, E0', E0, or similar. A few of the vitamins have voltammetry that is relatively simple to interpret, but in most cases cyclic voltammetric data include coupled chemical reactions. Throughout this review, estimates of E1/2 and so E0' are made to within 100 mV or better. Estimates of and E1/2 dependent on the electrochemical mechanism defined by
and
, are made as detailed by Bard and Faulkner.4 When stated in the literature, redox potentials and mechanisms are reported. Otherwise, literature voltammograms are reviewed to approximate mechanisms and estimate formal potentials from E1/2 values. Mechanisms are estimated from gross examination of voltammetric morphologies.
Perspectives on Vitamin Electrochemistry
This Section provides perspectives on the electrochemistry of the 13 vitamins recognized by NIH collectively and draws from kinetics and thermodynamics of the individual vitamins. The individual vitamins are reviewed and summarized in the Section Synopsis for Each Vitamin. Electrochemical properties are summarized in Table I. Relative E1/2 values for all the vitamins are mapped in Figure 1.
E1/2 and mechanistic data in Table I and potential axis of Figure 1
Perspectives are drawn from Table I and Figure 1. Table I summarizes E1/2 values near physiological pH and general information about the electrochemical mechanisms for each vitamin. Figure 1 is the potential axis that plots the approximate E1/2 values for all the vitamins and identifies oxidized and reduced forms. The potential axis is a useful but underutilized tool for charting thermodynamically possible vitamin reactions and interactions.
Table I: Shown in Table I are E1/2 near pH 7 for each vitamin, where E1/2 approximates within about 100 mV. These values are appropriate under standard conditions of unit concentration except as noted as a condition for formal potential (e.g., pH 7). Because for
,
, the case where
is also approximated by
. Formal potentials provide a first approximation to whether a reaction is thermodynamically feasible. Kinetics dictate whether thermodynamically feasible reactions occur. Formal potentials do not capture kinetics and so may not fully describe phenomena observed in the physiological microenvironments inside cellular structures. In Table I, the general mechanism for each vitamin (e.g.,
,
) provides a first impression of kinetics.
In the Table, data in water and aprotic solvents such as acetonitrile (MeCN) are provided. Water soluble vitamins can be evaluated in water, constrained to potentials within the water solvent window. In water at pH 7, thermodynamics specify that potentials positive of +0.8 V vs NHE allow evolution of O2 and potentials negative of −0.42 V vs NHE evolve H2 . Choice of electrode can enlarge the potential window where evolution of oxygen and/or hydrogen is kinetically suppressed. Water window shifts with pH, but the focus here is about physiological pH. Lipid soluble vitamins are not water soluble and are evaluated in aprotic solvents. Aprotic solvents have larger potential windows than water that allow measurement of potentials and currents at more extreme potentials. Because water soluble vitamins tend to be soluble in aprotic polar solvents, B vitamin voltammetry in aprotic solvents assesses more extreme potentials and so more energetic oxidation and reduction reactions than accessible in water.
Potential axis of Figure 1
The E1/2 values of Table I are plotted in potential order in the potential axis of Figure 1 with an uncertainty of about 100 mV at pH 7. The potential axis is shown in IUPAC convention with positive potentials to the right. On the axis, the generic reaction at
is denoted as R|O, with the reduced form on the left toward potentials negative of E1/2. The upright | is centered over
so that for potentials negative of
, R is the dominant form.
The potential axis is a useful tool for charting reactions that are and are not possible based on thermodynamics and for deconvolving complex reaction sequences. For two reactants, and
where E0'A|B is positive of E0'C|D, the potential axis is shown as D|C—–B|A. In this representation, the thermodynamics are such that if D and A are present, they can react spontaneously to form B and C. All other combinations (A + C, A + B, B + C, D + C, and D + B) are thermodynamically stable. The potential of redox couples plotted on a potential axis provides an initial assessment of what can react and what is stable. The difference in the
values for D|C—–B|A,
estimates the energy of the reaction between A and D. For a electrons transferred on reaction of A with D, the free energy of the reaction
.
The values are for species at unit concentration unless otherwise formally specified. Based on the Nernst equation where the stoichiometry for A to B is 1:1,
, the potential
when [B] = [A]. So, in systems where concentrations of A and B are comparable,
remains a reasonable estimate for the potential of the redox couple, as plotted in Figure 1. If concentrations of A and B are not well matched, the potential shifts from
in accord with the Nernst equation. The
values on the potential axis in Figure 1 at pH 7 are appropriate for similar concentrations of A and B, but if the concentrations are not well matched, the
value is less precise as the concentrations become more disparate. In Figure 1, a few potentials are noted at pH other than 7 so that E0' may differ by ≲ 100 mV at pH 7.
Separation of formal potentials should be considered in assessing reactivity. As formal potentials converge, reactions between the components A, B, C, and D can impact concentrations. Roughly, for a one electron transfer, separation of 180 mV or more is sufficient to prevent a significant reaction based on the equilibrium . For example, because the potential separation between vitamin B1 at 0.6 V vs NHE (B1|B1red) and B5 at 0.5 V vs NHE (B5|B5red) is not large, some reaction between B1 and B5 in solution is anticipated based on
. In Figure 1, below the vitamin potential axis, the limits of the water potential window at pH 7 are shown. Thermodynamic stability of the vitamins to acid (H+) and air (O2) can be assessed. For example, vitamin B1 is thermodynamically unstable in air at pH 7, based on B1|B1ox—–H2O|O2.
On the potential axis, the initial, native state of the vitamin is denoted in boldface, such as X. The oxidation and reduction products are denoted Xox and Xred. If a second reaction occurs, such as Xred is further reduced, the reduction product is denoted Xred, 2.
More data are cited in Table I than plotted on the potential axis of Figure 1. For vitamin A, reactions of retinol (A) and retinal (A') are shown and coupled as A is oxidized irreversible to A'. Values for β-carotene are not shown on the potential axis but are noted in Table I as Aβ|Aβ, ox at +0.8 V and Aβ, red|Aβ at −1.4 V, where the E1/2 are similar to those for A and A'. For B2 and B6, data are available in water and aprotic solvent; on the potential axis, values are shown for vitamin B6 in MeCN and vitamin B2 in water. Vitamin B9 is studied in water where B9 adsorbs to the electrode surface and complicates voltammetry. For vitamin B9, three or four reductions are reported8,9 but only the first B9red|B9 at −0.3 V is shown. Voltammetry for B12 varies with the axial ligand. The aquo complex exhibits voltammetry for a clean process as shown on the potential axis.
Evaluations of vitamin electrochemistry drawn from Table I and the potential axis of Figure 1
Data in Table I and Figure 1 allow evaluation of the vitamins collectively, as outlined below for a spectrum of redox events; groupings of the vitamin electrochemical mechanisms; identification of antioxidants; effects of pH; stability to air and acid; and, in a few cases, cooperative effects observed between vitamins. All reactions may occur directly or by mediation by a species that is not a vitamin. These examples illustrate the potential axis to chart electrochemical events. Examples generally increase in complexity as the review progresses.
Spectrum of observations
Drawn from Table I, the potential axis (Figure 1), and the properties of the individual vitamins, several general observations are made. When several vitamins are discussed, they are listed in order of E1/2 from negative to positive, to rank the energies of the half reactions.
- (1)All vitamins are electroactive.
- (2)All vitamins can serve as redox active components in physiological processes. The redox behavior will be impacted by whether the vitamin is water or lipid soluble. For the water soluble vitamins and vitamin K, redox properties are impacted by the availability of protons.
- (3)The lipid soluble vitamins are A, D, E, and K. Other than the B vitamins, only vitamin C is water soluble.
- (a)The lipid soluble vitamins, A, D, E, and K, and the water soluble C contain only C, H, and O.
- (b)The water soluble B vitamins all contain nitrogen. Biotin also contains sulfur.
- (c)Only vitamin B12 contains a metal, cobalt.
- (d)All the vitamins are solids at room temperature except B5, E, and K.
- (e)The vitamin with the lowest molecular weight is vitamin B3 (123.111 g/mol). Vitamin B12 has the highest molecular weight ( ∼ 1350 g/mol).
- (4)From the potential axis in Figure 1 where lipid soluble vitamins are shown in red and native state of the vitamins is marked in bold face:
- (a)The range of formal potentials represented as E1/2 covers the range of potentials fairly uniformly.
- (b)For the water potential window between −0.42 V and +0.81 V:
- (i)All water soluble vitamins except vitamins B3, B6, and B7 have formal potentials within the water window.
- (A)Vitamin B9 is reduced B9red|B9 within the water window but oxidized B9|B9ox at potentials positive of the water window.
- (B)Vitamin B12 exhibits a clean
mechanism where the first reduction B12red|B12 is in the water window but the second B12red, 2|B12red is outside the window at more negative potentials.
- (ii)Lipid soluble vitamins have formal potentials at potentials positive (A, D, E) and negative (A, K) of the water window, but none have potentials within the water window.
- (c)For the water window of 1.2 V, a potential gap of 0.4 V, 1/3 of the window, is between B5red|B5 and B12red|B12 at −0.2 and +0.21 V. The gap is centered at 0 V vs NHE.
- (d)All vitamins in the native state with formal potentials positive of the gap undergo oxidation X|Xox and native states with a formal potential negative of the gap undergo reduction Xred|X.
- (i)At the edge of the gap, B12 at positive potentials undergoes reduction B12red|B12, a behavior that differs from the other native vitamins. This may relate to control of peroxide; see Subsection Antioxidants.
- (5)The most extreme potentials are summarized as:
- (a)For the native state of the vitamins, are +1.4 to +1.5 V for D|Dox and −1.95 V for Ared|A, both lipid soluble.
- (b)For inclusion of the various oxidation and reduction products, +1.4 to +1.5 V for D|Dox and −2.13 V for Ared, 2|Ared.
- (c)In native states, neither D nor A is a high energy reactant, but both Ared and Ared, 2 are strong reducing agents. Dox is a strong oxidizing agent.
Mechanistic observations and groupings
Based on the mechanisms outlined in Table I, vitamins are grouped by mechanisms in Figure 1.
- (1)Five vitamins undergo only one electron transfer reaction: B3red|B3; B7red|B7; B2red|B2; C|Cox; and D|Dox, for E1/2 from negative to positive.
- (a)These water soluble vitamins are demonstrated proton dependent except biotin (B7), but B7 has a carboxylate group and predicted acid and base dissociation constants. Likely, B7 is also proton dependent.
- (b)The three B vitamins undergo reduction from their native state. Vitamins C and D undergo oxidation.
- (c)Simple
mechanisms characterize B2red|B2 (
) in water and B7red|B7 (
). Both B2 and B7 can serve as redox buffers when both halves of the couple are present. Because there are no following reactions, both easily serve as simple redox probes in academic studies.
- (d)B3red|B3, C|Cox, and D|Dox are
mechanisms and likely chemically irreversible (
). All can serve as sacrificial electron donors (C and D) and acceptors (B3).
- (i)Vitamin C is an especially important reducing agent based on its E1/2. See Subsection Antioxidants.
- (ii)Of the vitamins in their native states, B3 and D have extreme E1/2 values Their utility in redox control of the system is when they are reduced and oxidized to B3red and Dox, very strong reducing and oxidizing agents, respectively. The energetic reactions of B3red and Dox may explain the irreversible following reactions (
) found for reactions of the native B3 and D.
- (2)The B vitamins B1, B9, B5, and B6 each undergo a reduction (Xred|X) and an oxidation (X|Xox) from the native state.
- (a)If these B vitamins are both reduced and oxidized, the energy is available to regenerate X as Xred + X
2X.
- (b)The energy of the reaction Xred + X
2X ranks with the difference in the formal potentials. Here the energy of the disproportionations increases as B5 (0.7 V), B1 (1 V), B9 (1.3 V), and B6 (1.9 V).
- (i)The midpoint potential (Eox1/2 + E1/2red)/2 for the four vitamins is fairly invariant: B5 (0.15 V vs NHE), B1 (0.10 V vs NHE), B9 (0.35 V vs NHE), and B6 (0.15 V vs NHE). The midpoint potentials fall into the gap on the potential axis and correlate well with the midpoint potential for the water window (0.2 V vs NHE at pH 7).
- (ii)Vitamins B5, B1, and B9 are evaluated in water. The three vitamins provide a voltage that increases in steps of 0.3 V. Note evaluation of B9 is complicated by adsorption to the electrode in water, where several adsorption peaks are observed.
- (iii)When E1/2 values are within about 200 mV, the possibility of reactions between the two couples should be considered. The potential differences for B5, B1, B9, and B6 are sufficiently large that no significant equilibrium from the native state to form Bred and Box is anticipated.
- (iv)B6 has the largest potential difference with E1/2 values for B6red|B6 and B6|B6ox sufficiently extreme that B6 is evaluated in aprotic solvents.
- (v)The formal potentials for water soluble vitamins tend to cluster so that there are formal potentials within 100 mV. For example, oxidations include {B9|B9ox and B6|B6ox}; {B5|B5ox, B1|B1ox, and C|Cox}, whereas reductions are {B6red|B6 and B12red, 2|B12red} and an impressive four component {B2red| B2, B5red|B5, B9red|B9, and B1red|B1}. All equilibrate to allow reaction with other vitamins with similar
values.
- (A)These closely grouped formal potentials allow reactions at equilibrium that can convert a significant fraction of vitamins to higher energy forms. For example, B5ox in the presence of B1 will equilibrate to form some concentration of the higher energy oxidant B1ox and stronger reductant B5.
- (B)Mixtures of species tends to redox buffer the environment and limit drastic excursions of potential.
- (c)Mechanistically for this group:
- (i)The only simple
mechanisms are B1red|B1 (
) and B6red|B6 (
). Both can serve as simple redox probes in academic studies. If both the native and reduced forms of the couple are present, a redox buffer may be formed.
- (ii)The remaining reactions in this group are classified as
, with most likely
. Comment on B9red|B9 is reserved as adsorption complicates interpretation of B9 voltammetry.
- (3)Two vitamins undergo two electron transfers that are characterized by a simple
mechanism: K and B12.
- (a)Both exhibit two well separated voltammetric waves.
- (b)Both undergo sequential reductions, Xred|X and at more negative potentials Xred, 2|Xred. If both X and Xred, 2 are present, disproportionation will generate Xred.
- (c)B12 is likely pH dependent, with predicted pKa and pKb values and by observation for B12red|B12red, 2.
- (d)Vitamin K exhibits two well resolved voltammetric waves in acetonitrile with ≲ 50 mM water, but as water is increased, the waves merge until at 7.2 M water, a single two electron wave is observed with Eave of −0.5 V, comparable to the −0.6 V observed for Kred|K in low water content.10
- (4)Vitamin E also has two electron transfer reactions, but the potentials are such that disproportionation occurs at
. On oxidation of vitamin E, spontaneous reaction can occur to regenerate E. This recycles vitamin E. The disproportionation can tend to maintain the redox potential near 1 V vs NHE, between the two E1/2 values for vitamin E. Vitamin E is the only vitamin noted with disproportionations of this type.
- (5)Vitamin A is composed of retinol (A) and retinal (A') and sometimes includes the precursor, β-carotene (Aβ). Vitamin A electrochemistry is the most complex of the vitamins.
- (a)A is oxidized irreversibly to A' at 1.0 V vs NHE. Then A' can be oxidized to A'ox at 1.3 V.
- (b)For the reductions, A is reduced to Ared at −1.95 V, with a subsequent reduction Ared, 2|Ared reported at –2.13 V. For retinal, A'red|A' is reported at −1.1 V with a following reduction A'red, 2|A'red at −1.5 V.
- (c)Based on D|C—–
, A and A' are stable in the presence of both.
- (d)β-carotene undergoes an oxidation Aβ|Aβ, ox and a reduction Aβ, red|Aβ at +0.8 and −1.4 V, respectively. The E1/2 value for Aβ|Aβ, ox overlaps with Eox|Eox, 2 and Aβ, red|Aβ overlaps with B3red|B3. Potentials for β-carotene are not shown on the potential axis.
- (e)Mechanistically, all reactions of vitamin A are an electron transfer followed by a chemical step (
) except A'red|A' and Aβ|Aβ, ox, which are both
.
Antioxidants
Reactive oxygen species (ROS) are oxygen containing molecules, ions, and radicals that drive energetic reactions. Energy dissipation often leads to indiscriminant reactions as ROS react to lower energy. Within biological systems, high energy ROS reactions damage cellular processes. Examples of ROS include peroxide and the radicals superoxide O• −2, peroxyl HO•2, and hydroxyl HO•. Generation of ROS radicals from oxygen and peroxide occurs in an electrochemical reaction sequence that is mapped on the potential axis. The energy available from an electron transfer reaction at unit activity D|C—–B|A is characterized by the difference in the formal potentials of the half reactions. Formal potentials are mapped for several ROS below the potential axis in Figure 1 at pH 7. Formal potentials are from Buettner's excellent paper.11 A thorough review of ROS with comments on kinetics is provided by Krumova and Cosa.12
The sequence that generates ROS radicals from oxygen and peroxide is followed on the potential axis. When oxygen is present, O2 can be reduced to peroxide and from peroxide and oxygen, other ROS radicals are formed. This is mapped on the potential axis where the first step is the formation of peroxide on the reduction of oxygen H2O2|O2 at 0.28 V. From peroxide, the more reactive superoxide, peroxyl, peroxide, and hydroxyl radicals can be formed. Peroxide oxidizes to superoxide H2O2|O• −2 at 0.94 V, and with protons, peroxyl radical is formed at a comparable potential, H2O2|HO•2 at 1.06 V. Peroxide can be reduced at 0.32 V to form hydroxyl radical HO•|H2O2. Radicals superoxide and peroxyl are similarly formed from oxygen (O• −2|O2, −0.33 V and HO•2|O2, −0.46).
Once formed, peroxide and superoxide, peroxyl, and hydroxyl radicals are available as higher energy oxidants: H2O|H2O2 at 1.35 V; H2O2|O• −2 at 0.94 V; H2O2|HO•2 at 1.06 V; and H2O|HO• at 2.31 V. Each radical also provides a lower energy reductant: O• −2|O2 at −0.33 V; HO •2|O2 at −0.46 V; and HO•|H2O2 at 0.32 V. Each radical can react by disproportionation back to peroxide and oxygen or water R•|C—–B|R•. Damage to cells occurs when the radical reactions are other than disproportionation. Conversely, if properly controlled, cells may exploit energy available from the radical reactions to build energy and materials within the cell.
Antioxidants reduce high energy oxidant species to form lower energy species. For example, from the potential axis and D|C—–B|A, vitamin C is an antioxidant for H2O2, O• −2, HO•2, and HO•, where reaction yields products of water and peroxide, where peroxide can undergo subsequent reaction with vitamin C to form water. Prooxidants serve a similar function but oxidize a reduced species. From the potential axis, examples may include reactions of B12 with superoxide and peroxyl radicals.
Various vitamins are identified as having antioxidant activity. From the potential axis and unit activity, the vitamins that may serve as antioxidants for the several ROS shown in Figure 1 are established by D|C—–B|R where . The antioxidant is D and R is the ROS that D reduces. Potential differences between E1/2 for D|C and B|R of about 180 mV (EB|R1/2 − E1/2D|C ≳ 180 mV) are energetically sufficient to allow near complete reduction of R. Vitamins B2, B3, and B7 are only reduced and do not serve as antioxidants for these ROS. Vitamin B2 can oxidize HO•2 and to some extent, superoxide. Vitamins that have appropriate E1/2 to serve as antioxidants for peroxide, oxygen, and superoxide, peroxyl, and hydroxyl radicals are summarized in Table II. Only native vitamins are shown, but Eox has a sufficient E1/2 value to fully reduce O• −2, HO •2, H2O2, and HO• and partially react with O2.
Table II. Table of Vitamin Antioxidants for Selected ROS. Complete reaction is noted as ✓ and at least partial reaction as ○, based on differences in E1/2 values as plotted on the potential axis. Conditions are unit activity at pH 7.
H2O|HO• | H2O|H2O2 | H2O2|HO•2 | H2O2|O• −2 | H2O|O2 | ||
---|---|---|---|---|---|---|
E1/2 | 2.31 | 1.35 | 1.06 | 0.94 | 0.81 | |
C|Cox | 0.39 | ✓ | ✓ | ✓ | ✓ | ✓ |
B5|B5ox | 0.5pH4 | ✓ | ✓ | ✓ | ✓ | ✓ |
B1|B1ox | 0.6 | ✓ | ✓ | ✓ | ✓ | ✓ |
B9|B9ox | 1.0 | ✓ | ✓ | ○ | ||
A|A' | 1.0 | ✓ | ✓ | ○ | ||
B6|B6ox | 1.1 | ✓ | ✓ | ○ | ||
E|Eox | 1.2 | ✓ | ✓ | |||
A'|A'ox | 1.3 | ✓ | ○ | |||
D|Dox | 1.45 | ✓ |
pH dependence
Available proton impacts many redox reactions. Square schemes (Scheme 1) chart proton dependent redox reactions. For a PCET reaction , the Nernst equation is
. For a formal potential at fixed pH,
. When dissociation reactions occur to sufficient extent, varied degrees of protonation can arise and formal potentials will vary. The impact of protonation on species is illustrated in the distribution diagram for niacin in Subsection Vitamin B3 - Niacin. In proton coupled electron transfer (PCET) redox mechanisms, protons as well as electrons can be transferred and availability of protons will affect the reaction path.
In Table I, pH dependence of the reactions is noted as either observed experimentally or anticipated based on available and predicted acid and base dissociation constants, pKa and pKb. For studies in aprotic solvents where proton concentration is low, proton impacts are better identified from characteristics such as dissociation constants and charge of the native vitamin. Dissociation constants, either measured or predicted, are shown for each vitamin in Section Synopsis for Each Vitamin. When pKa and pKb values exceed about 15, no significant dissociation is anticipated. A charged native vitamin can indicate a proton dependent process. In species with both a carboxylic acid group or other oxygen containing groups with reported pKa and a nitrogen that can be protonated (pKb), dissolution of the neutral solid can yield a zwitterion in solution. Zwitterions at physiological pH, such as B3, are proton dependent despite no net charge. Charge at physiological pH is presented in the Table of General Properties for each vitamin.
From Table I and tabulated dissociation constants and charge, some dependence on proton concentration is anticipated for all vitamins with a few caveats. The native vitamins with zero charge are B3, B6, A, D, E, and K. B3 is a zwitterion. B6 has both a pKa and pKb and the pyridine nitrogen is easily protonated. Vitamin A as retinol (A) has an extreme pKa and pKb and may tend to proton independence. Vitamin D has reported pKa and pKb and anticipated proton dependent. Vitamin E has one reported pKa of 10.8 and a quinone-like ring structure that will likely be proton dependent, however the pKa is sufficiently high that little proton dependence is anticipated at physiological pH. K has three pKa values. In Table I, B1 is reported to be proton independent for ranges of pH. B1 has essentially one pKa around 5, so pH independence for pH ≲ 3 and pH ≳ 7, is consistent with the reported pH independence.
Cells are composed of aqueous and lipid domains where interfaces between the domains are established. Lipid soluble vitamins at interfaces may access protons in the aqueous phase and so redox potentials may differ from those in aprotic environments that include lipid domains. Vitamin K voltammetry is dependent on water content so that vitamin K at interfaces with different water content could be positioned in the interface to establish a range of potentials that varies over a 700 mV range. The E1/2 for vitamin D is perhaps tuned by water content at aqueous lipid interfaces. Based on possible proton dependent electrochemistry, lipid soluble vitamins D and E may shift E1/2 with proximity to aqueous lipid interfaces. Similarly, for water soluble vitamins present in lipid domains with few to no protons, E1/2 values may shift.
Stability: air, acid and water
Numerous reports in the literature discuss vitamin stability under various conditions such as solids and solution and processing such as extrusion, formulation, and cooking.13–19 Vitamins may be unstable to heat and light as well as exposure to acid, alkali, air, and moisture. Review of four References 13–16 flagged vitamins unstable in acid, alkali, and on exposure to air (oxygen) and moisture. In Table III, literature reports of instabilities are noted. If data were found in two or more of the four references, ✓✓ is shown and if high instability was noted, ✓✓✓ is shown. If reference to instability was found in only one reference, ✓ is marked. With the exception of vitamin C, instability in moisture was noted in only one Reference 15. In Table III, native vitamins are ordered by E1/2 values.
Table III. Table of Vitamin Stability. For acid, alkali, air, and moisture, vitamins instability is noted for native vitamins. Vitamins are tabulated by E1/2 from negative to positive values.
Acid | Alkali | Air/O2 | Moisture/humidity15 | |||||
---|---|---|---|---|---|---|---|---|
E1/2 | Literature | Pot. Axis | Literature | Pot. Axis | Literature | Pot. Axis | Literature | |
Ared|A | −1.95 | ✓✓ | ✓✓ | ○ | ||||
B3red|B3 | −1.4 | |||||||
A'red|A' | −1.1 | ✓✓ | ✓✓ | ○ | ||||
B7red|B7 | −1.0 | ✓✓ | ✓✓ | ○ | ||||
B6red|B6 | −0.8 | √− | √√− | ○ | ○ | |||
Kred|K | −0.6 | ✓ | ✓✓✓ | ○ | ✓ | |||
B1red|B1 | −0.38 | •• | √√− | •○ | ✓ | •○ | ✓ | |
B9red|B9 | −0.3pH5.2 | ✓✓ | •• | ✓ | •○ | ○ | ||
B2red|B2 | −0.22 | • | ✓✓✓ | ••○ | ||||
B5red|B5 | −0.2pH6 | ✓✓✓ | • | ✓✓✓ | ••○ | •○ | ✓ | |
B12red|B12 | 0.21 | ✓✓ | ✓✓✓ | ••○ | ✓ | |||
C|Cox | 0.39 | ✓✓ | ✓✓✓ | ✓✓✓ | •○ | ✓✓ | ||
B5|B5ox | 0.5pH4 | ✓✓ | • | ✓✓✓ | ••○ | •○ | ✓ | |
B1|B1ox | 0.6 | •• | ✓✓✓ | •○ | ✓ | •○ | ✓ | |
B9|B9ox | 1.0 | ✓✓ | •• | √− | •○ | ○ | ||
A|A' | 1.0 | ✓✓ | ✓✓ | ○ | ||||
B6|B6ox | 1.1 | √− | √√− | ○ | ○ | |||
E|Eox | 1.2 | ✓✓ | ✓✓ | ○ | ||||
A'|A'ox | 1.3 | ✓✓ | ✓✓ | ○ | ||||
D | ||||||||
Dox | 1.45 | ✓ | ✓ | ✓✓ |
Vitamins identified as stable in Reference 14 are B3 ≳ E, B7 > B1, B9 where B1 is unstable in alkali and B9 in acid and base. Unstable vitamins are A, B5, C, and B12. These patterns are reflected in the literature summaries in Table III.
The potential axis allows an assessment of vitamin stability to redox reactions from a thermodynamic view. It does not address kinetic effects where despite thermodynamic possibility for reaction, kinetics are slow and the reaction does not proceed substantially. From the potential axis where D|C—–B|A and spontaneous reaction that lies to the right, redox stability of vitamins can be anticipated. The water window at pH 7 noted in Figure 1 is marked in Table III by double bars, bracketed by B1. At values of pH other than 7, the water window shifts. The water window shifts with pH as follows. The pH dependent formal potential for H2|H+ is expressed as
where
V vs NHE, so that as the system is less acidic, formal potential shifts negative. For H2,OH−|H2O, formal potential is
where
V vs NHE, so as the system is less basic, formal potential shifts positive. The hydrogen evolution reaction is expressed in acid as
(H2|H+) and in base as
(H2,OH−|H2O). At pH 7, the voltage is −0.42 V vs NHE for the two equivalent reactions. For the native vitamins, E1/2 for the vitamins may also shift, dependent on the vitamin acid and base dissociation constants. The details of vitamin E1/2 shift with pH are not characterized here so that discussion of instability at pH other than 7 are framed with the constraint that vitamin E1/2 does not shift substantially with pH.
The potential axis is used to predict redox stability in air, acid, base, and water. Predicted redox instability estimated from the potential axis is marked in Table III at pH 7 with •, where •• indicates a predicted high instability. At pH other than 7, where predicted redox instability is assessed without consideration of shifts in vitamin E1/2, instability is denoted with ○.
• Stability in Acid: Stability in acid at pH 7 is set by H2|H+ at −0.42 V. Based on the potential axis in Figure 1, native vitamins with E1/2 negative of about −0.6 V vs NHE are expected redox stable in acid. Vitamins B1 and B9, and to a lesser extent, B2 and B5, will be somewhat acid unstable as E1/2 values for the four Bred|B are within about 180 mV of H2|H+ at pH 7. As pH drops, H2|H+ shifts to −0.12 V at pH 2 and 0.0 V at pH 0. At lower pH, if E1/2 for the four B vitamins does not shift substantially with pH, the B vitamins are more redox stable. In very strong acid, B12 and perhaps C may react with proton as H2|H+ is about 0 V vs NHE. All reduction products of vitamins, Xred, with E1/2 < −0.6 V may be converted back to the native species at pH 7 if the reduction is chemically reversible. In general, redox instability in acid is predicted within the water window at pH 7 for water soluble vitamins. Predicted redox instability for B1 and B2 is not noted in the literature and observed acid instability for B7 and fat soluble A were not predicted as redox instability.
• Stability in Base: At pH 7 for H2,OH−|H2O at −0.42 V, vitamins B1 and B9 are predicted somewhat unstable to base; B2 and B5 are more unstable; and B12 and A' may exhibit substantial instability, although A' is not water soluble. At pOH 0, V and provided shifts of vitamin E1/2 are small, vitamins B6, K, B1, B9, B5, B2, B12, and perhaps B7 may be unstable in strong base. Compared to the literature reported instabilities, the potential axis predicted redox instability for all but vitamins E and C. In general, vitamins are predicted less stable in base than acid.
• Stability in Air: Stability in air or oxygen in water at pH 7 where no peroxide is present is based on H2O|O2 at 0.81 V vs NHE. The native vitamins where reaction with oxygen is thermodynamically possible are C|Cox at 0.39 V; B5|B5ox at 0.5 V; and B1|B1ox at 0.6 V. At lower pH, H2O|O2 shifts to more positive potentials and A, B9, B6, E, and A' are susceptible to instability in air provided the E1/2 values for the native vitamins do not shift substantially with pH. In the stomach, at pH 2, H2O|O2 is at 1.18 V vs NHE, such that C, B5, B1, A, and B9 may be less stable to oxygen and B6, E, and A' may be at least partially unstable to oxygen on ingestion, provided the vitamins do not shift E1/2 substantially with pH at lower pH. All reduction products of vitamins, Xred, may be converted back to the native species in air at pH 7 if the reduction is chemically reversible. Redox stability is predicted based on no ROS present, but in literature reports, ROS may be present. Compared to the literature reports, predicted redox instability is found for all observed instabilities in the literature except for vitamin D, which is only oxidized by high energy ROS such as HO•. Predicted redox instabilities that are not noted in the literature are for B6, B9, B5, and B1. These four native B vitamins each undergo both an oxidation and a reduction, where the reductions Bred|B are clustered near H2, OH−|H2O and B|Box are clustered near H2O|O2. A mechanism for air stability of these four B vitamins is B reacts with O2 to make Box and B reacts with OH− to make Bred. Because these are set as Bred|B—–B|Box with well separated potentials, . This would account for no observed redox instability in the literature and illustrate how the potential axis can be applied to handle more complex reactions.
• Stability in Water: All of the vitamins should be redox stable in water near physiological pH, based on the potential axis. Literature reports of moisture instability are found for B1, B5, B12, and C. These all fall within the water window and are based on exposure to water vapor in air. For B1, B5, B12, and C instability in air is predicted. Likely the literature values of moisture instability are for conditions where water and oxygen are present, which would lead to redox instability as predicted from the potential axis.
Reports of instabilities through vitamin vitamin interactions
In Reference 14, several instances of vitamin vitamin interactions are noted where the interactions degrade at least one vitamin. Decomposition products of B1 breakdown both B9 and B12, which can proceed by B1red (B1red|B1) reacting with B9 or B12 to generate B9red and B12red. Reactions with B2 are said to oxidize B9, B1, and C, in some cases with light. Based on the E1/2 for B2red|B2, riboflavin will not oxidize these three vitamins. B2 may lead to partial reduction of B1 and B9 because the E1/2 values are similar. Superoxide O − •2 and O2 oxidize C, B1, and B9 to the oxidized forms. If B2 is reduced, B2red can reduce O2 to peroxide where H2O| H2O2 at 1.35 V vs NHE at pH 7 has sufficient potential for peroxide to oxidize C, B1, and B9, but the reaction would likely be indiscriminant and peroxide would oxidize all vitamins that can be oxidized except vitamin D. Further, the potentials to reduce B2 are clustered together with reduction of B9, B1, and B5 so that it is not clear why B2 would have a unique effect. Light may excite higher energy states of B2 that have more positive E1/2 values, perhaps > 1.2 V.
Cooperative effects between vitamins
Several cooperative effects between pairs of vitamins have been reported. Because potential is well mapped across 3 V by the array of vitamins, coupling between the redox states of two vitamins to tailor a redox potential is possible. Direct reaction between the vitamins may occur or the reaction may be mediated by a species that is not a vitamin.
Vitamins C and E interactions
In Alzheimer's disease, elevated levels of lipid peroxidase are found in the brain, plasma, and cerebral spinal fluid.20 Vitamin E decreases the levels of lipid peroxidase to form the radical cation E+ •, which is mapped as E|Eox near 1.2 V on the potential axis. Because vitamin E maps as Eox|Eox, 2 —–E|Eox, Eox can disproportionate to form Eox, 2 + E so that half the native E is regenerated. When vitamin C is also present, vitamin C can convert Eox, 2 to Eox and then convert Eox to E so that all E is regenerated. Vitamin C is a sacrificial electron donor that is irreversibly oxidized to Cox. Because E|Eox reduces lipid peroxidase, the E1/2 for lipid peroxidase is likely at least 1 V vs NHE. Vitamin E is a lipophilic antioxidant. Vitamin C is thermodynamically able to reduce lipid peroxidase directly.
Vitamins C and K3 interactions
Synergistic activity between vitamins C and K3 is used to generate free radicals that destroy tumor cells by autoschizis.21 Vitamin C is commonly used as an antioxidant but here serves as a prooxidant. Vitamin K3, menadione, is a synthetic provitamin with electroactive naphthoquinone structure as for other K vitamins but with no side chain. Adams and coworkers report that E1/2 for vitamin K3 is +70 mV of E1/2 for vitamin K1.22 Reduced forms of vitamin K commonly serve as antioxidants, but can also serve as prooxidants. A mechanism generates Kred and Cox that are recycled to K and C and generate ROS that destroy the tumor intracellular structures. The process uses reactions that are not well separated but have E1/2 values sufficiently close that some degree of reaction occurs. Components of the process may be as follows, based on the potential axis. C|Cox and H2O2|O2 are within ≈ 100 mV and will generate some Cox and peroxide. Reference 21 reports a ratio of vitamin C to K of 100:1, which will shift the potential for C|Cox closer to E1/2 of K3red| K3. Peroxide undergoes disproportionation as H2O2|HO•2 —–H2O|H2O2 or similarly to form superoxide. The potentials for K3red|K3 and HO•2|O2 are within 100 mV, so peroxyl reacts with the semiquinone K3red to regenerate K3. Cox is reduced to C by HO•2 and O• −2. Or, K3red and Cox can regenerate to leave reactive species such as H2O2, O• −2, and HO•2 to react to other radicals, some of which are highly energetic ROS, such as hydroxyl radical HO•.
Vitamins B12 and B9 interactions
Deficiencies of B12 and B9 lead to neurological symptoms and disorders. Both vitamins participate in conversion of homocysteine to methionine where B12 and folate derivatives are regenerated.23–25 Folate is oxidized to tetrahydrofolate (THF). Methyl tetrahydrofolate (Me-THF) is converted to THF as the methyl group binds to the Co(I) form of B12 to form methylated Co(III) B12 that transfers a methyl to homocysteine to form the amino acid methionine and regenerate Co(I) B12.24 A rough, qualitative assessment for the process is made if THF is taken to have E1/2 of oxidized B9ox. The active form of B12 is the Co(I) derivative, which is analogous to B2red, 2. When the methyl is transferred, Co(I) B12 is oxidized to Co(III) B12 and THF is reduced to B9. B12 as Co(I) is regenerated as the methyl is transferred to homocysteine and THF is oxidized to Me-THF for the next turnover. No quantitative assessment is available, but the qualitative processes of electron transfer are consistent with the E1/2 for B12 and B9. Formation of the Co(II) B12 deactivates the B12 catalyst.
Perspective summary
These and other reaction possibilities are derived from the collective data for the vitamins summarized in Table I and Figure 1. Application of the potential axis to identify thermodynamically feasible reactions can be extended to reactions with other intermediates and cofactors.
Mechanistic and E1/2 data in Table I and Figure 1 are compiled from a critical assessment of the literature data for the individual vitamins. Literature for the individual vitamins was identified by a search in SciFinder and Google Scholar between May and July 2017 with search terms such as electrochemistry and voltammetry of a specific vitamin (e.g., vitamin B3). Identified papers were reviewed to extract voltammetric and electrochemical information from the most rigorous of the identified papers. Additional information summarized for each vitamin was collected from several common sources from the National Institute of Health (NIH).1,26,27 Few reviews of vitamin electrochemistry were found. Most focused on electroanalysis.28–30 Webster wrote an excellent review of lipid soluble vitamin electrochemistry based on careful voltammetric studies from his group.31 Biochemistry of vitamins is well reviewed in Reference 32. Buettner reviewed electrochemical potentials for some important biochemical radicals.11
Follows is a summary of the voltammetry literature for each vitamin where reports are drawn from a critical assessment of the available literature. These data provide more details about the electrochemistry of each vitamin.
Synopsis for Each Vitamin
Vitamins are classified as water soluble and lipid soluble. The water soluble vitamins, C and B vitamins are shown in Figure 2. The lipid soluble vitamins, A, D, E, and K, are shown in Figure 3. The water soluble vitamins are described first. For each vitamin, a brief history and description of actions and deficiency in humans is followed by a description of voltammetry and the determination of electrochemical formal or half wave potential.
Figure 2. Water Soluble Vitamin Structures.26
Figure 3. Fat Soluble Vitamin Structures.26
Vitamin C - Ascorbic Acid
Foods rich in vitamin C have been used to prevent scurvy since at least the 16th century.34 In 1907, Axel Holst and Theodor Frolich, two Norwegian physicians, observed that scurvy is not unique to humans. They noticed that guinea pigs fed a diet of foods free of vitamin C exhibited classical symptoms of scurvy. They later showed that the symptoms are relieved by a diet of foods rich in vitamin C.35 The discovery of vitamin C occurred ca. 1930, when Albert Szent-Gyogyi successfully isolated hexuronic acid that was later determined to be vitamin C.36 Szent-Gyogyi was later awarded the Nobel Prize in medicine for this discovery.37 The structure of Vitamin C is shown in Figure 2a. General chemical characteristics are listed in Table IV.
Table IV. Chemical Characteristics of Vitamin C. Unless otherwise noted, chemical characteristics for the individual vitamins are drawn from References 3, 26, 27, and 33.
IUPAC Chemical Name | (2R)-2-[(1S)-1,2-dihydroxyethyl]- |
3,4-dihydroxy-2H-furan-5-one | |
Alternative Names | Nicotinic acid; Niacin; Pyridine-3-carboxylic acid |
Chemical Formula | C8H8O6 |
Molecular Weight (g mol− 1) | 176.124 |
Solid at room temp | Solid pale yellow powder |
Density (g/cm3) | 1.65 |
Melting Point (![]() |
191 (decomposes) |
Boiling Point (![]() |
decomposes |
Flash Point (![]() ![]() |
193![]() ![]() |
pKa | 4.7 |
Physiological Charge | −1 |
Solubility in Water | 0.4 g/mL of water at 40°C |
Stability | stable in air |
Chemical Class | Ester |
Action in humans and signs of deficiency
Vitamin C deficiency results in scurvy, a disease commonly associated with 18th century sailors. Scurvy symptoms include body sores, fatigue, malaise, and inflammation of the gums. If left untreated, scurvy will result in death.1
In the human body, vitamin C has many functions that include vitamin C as an antioxidant that donates electrons in both enzymatic and nonenzymatic reactions. For example, the synthesis of carnitine relies on an enzyme dependent on vitamin C.38 A more comprehensive review can be found in the paper by Levine, et al.38
Voltammetry and electrochemistry
Cheng et al.39 report cyclic voltammetry of L-(+)-ascorbic acid on a graphite/epoxy working electrode at pH 7.4. See Figure 4.
Figure 4. Cyclic voltammogram for Vitamin C recorded on polished graphite/epoxy electrodes at 50 mV/s in pH 7.4 phosphate buffer. Potentials shown are vs. SCE.39 Permission from ACS.
The cyclic voltammograms appear to show an electrochemical step followed by a chemical reaction consistent with an mechanism for vitamin C. Kuwana et al.40 report cyclic voltammetry focused on the oxidation of ascorbic acid at active and inactive glassy carbon electrodes; see Figure 5. Active glassy carbon refers to a specific surface treatment method illustrated by Kuwana et al. in previous reports41 where glassy carbon electrodes are activated via polishing and vacuum heat-treatment.
Figure 5. Cyclic voltammogram of 0.1 mM ascorbic acid on active (a) and inactive (b) glassy carbon electrodes at pH 2.0 (phosphate buffer) at 100 mV/s. The oxidative response is enhanced on active glassy carbon electrodes consistent with a mechanism dependent on electrode surface condition.40 Permission from ACS.
Li et al.42 report cyclic voltammetry of ascorbic acid at bare and poly(caffeic acid) modified glassy carbon disk electrodes. The cyclic voltammetric data are consistent with an mechanism with a fast following chemical step. Additional cyclic voltammetric reports are consistent with the
mechanism. Hart et al.43 report cyclic voltammetry of ascorbic acid on graphite/epoxy electrodes, both unmodified and modified with cobalt phthalocyanine powder. To maintain stability of the vitamin, all studies were done at pH 5.
Consistent with an mechanism, the E1/2 is ≈ 0.1 V vs. SCE.43 The peak current increases and the peak potential shifts positive with a decrease in pH.
Formal potential E0'
The formal potential for the mechanism is estimated from the peak potential of the oxidation. Table V summarizes the estimated formal potentials taken from cyclic voltammograms recorded on the unmodified electrodes at various pH.
Table V. Collection of Estimated Formal Potentials for Vitamin C as a Function of pH.
Estimated Formal Potential (vs. NHE) | pH | References |
---|---|---|
0.824 V | 2.0 | 40 |
0.594 V | 5.0 | 43 |
0.1494 V | 7.4 | 39 |
0.299 V | 7.4 | 42 |
Buettner11 notes two one electron reactions of ascorbate that are ascorbate anion C−|C− • at 0.28 V and DHA|C− • at −0.17 V vs NHE, where DHA is dehydroxyascorbate. Both reactions are too rapid to be captured separately by voltammetry and the cyclic voltammetrically estimated potentials are for the 2 electron 2 proton process.
Vitamin C: summary comments on electrochemistry
Vitamin C undergoes a two electron transfer at ∼ 0.39 V vs. NHE at pH 7. that is followed by an apparently irreversible chemical step, an mechanism. Voltammetry reported for a range of pH (Table V) demonstrates the electron transfers are coupled 2 e 2 H+ processes.
Vitamin B1 - Thiamine
Vitamin B1 was identified in 1897 by Christiaan Eijkman at Utrecht University. His interest in beriberi, also known as thiamine deficiency, grew during his time in the Army in the Dutch East Indies. His experiments on chickens showed the dietary root of the disease is vitamin B1 deficiency. Eijkman was awarded the 1929 Nobel Prize in Physiology or Medicine for his discovery.45 The structure of thiamine, B1 is shown in Figure 2d. General chemical characteristics are listed in Table VI.
Table VI. Chemical Characteristics of Vitamin B1.3,26,27,33
IUPAC Chemical Name | 3-[(4-amino-2-methylpyrimidin-5- | |
yl)methyl]-5−(2-hydroxyethyl)-4-methyl-1,3-thiazol-3-ium | ||
Alternative Names | Aneurin, Antiberiberi factor | |
Chemical Formula | C12H17N4OS | |
Molecular Weight (g mol− 1) | 265.355 | |
Solid at room temp | Solid, slight odor | |
Melting Point (![]() |
248![]() |
|
pKa | (1) 15.5 (strongest acidic); 5.54 (strongest basic); (2) pKa1 = 19; pKa2 = 2 ∼ 5 | 44 |
Physiological Charge | 1 | |
Solubility in Water | 500 mg/mL of water | |
Solubility in lipids/fats | insoluble | |
Chemical Class | Diazines (pryimidines and primidine derivatives) |
Action in humans and signs of deficiency
Thiamine is used to synthesize thiamine pyrophosphate, a cofactor for enzymes involved in carbohydrate and branched chain amino acid.46 Deficiency in thiamine causes the metabolic disease, beriberi, and multiple neurological disorders. Beriberi, which is often associated with malnourishment, affects the peripheral nervous system, cardiovascular system, and digestive system. The resulting Wernicke's encephalopathy causes metabolic lesions that lead to unsteady gait and vision loss in extreme vitamin B1 deficiency. Korsakoff syndrome from vitamin B1 deficiency causes anterograde and retrograde amnesia and other memory impairments. B1 deficiency often occurs in conjunction with long term alcohol abuse.47
Voltammetry and electrochemistry
Vitamin B1 undergoes distinct oxidation and reduction processes. Peak potentials, kinetics, and mechanisms vary with electrode material and solution pH.
Thiamine oxidation
Nyokong et al.48 studied detection of vitamin B1 on modified carbon paste electrodes at pH = 10. They characterized the oxidation reaction as a two electron process (Equation 2). The voltammetric curve in Figure 6 is characteristic of an process, an electron transfer step followed by a rapid chemical reaction. The peak potential reported in the literature is ∼ 0.4 V vs Ag|AgCl ( ∼ 0.6 V vs NHE). Tsang et al.49 found for an oxidative sweep at glassy carbon electrodes, the cathodic peak potential is about 0.330 V vs Ag|AgCl (0.527 V vs NHE), a peak potential that did not vary with pH for pH 9.0 to 13.0.
![Equation ([2])](https://content.cld.iop.org/journals/1945-7111/165/2/G18/revision1/d0002.gif)
Figure 6. Cyclic voltammograms of vitamin B1 at a carbon paste electrode with scan rate of 0.1 V s− 1 at pH 10. The peak potential is ∼ 0.4 V vs Ag/AgCl. Concentrations are (a) 0, (b) 2.5, (c) 5.0, (d) 8.0, and (e) 10.0 × 10− 5 M.48
Thiamine reduction
Sutton and Shabangi44 presented cyclic voltammograms for the reduction of thiamine pyrophosphate in 1 M KCl on a platinum electrode. Thiamine pyrophosphate undergoes a quasireversible electron transfer centered at −0.58 V vs Ag|AgCl (−0.38 V vs NHE). Peak potentials for thiamine were noted within 60 mV of peak potentials for thiamine pyrophosphate. As pH increases, the cathodic and anodic peaks decrease, but the peak potentials do not shift. The result is consistent with deprotonation of the thiamine pyrophosphate to an electroinactive form, but thiamine pyrophosphate reduction is not pH dependent. The pKa of the pyrimidine nitrogen is estimated as ∼ 5.50,51 Protonation of B1 is thought required to observe thiamine reduction.52 Sutton and Shabangi provide a multistep dimerization mechanism.44 The voltammetry of thiamine pyrophosphate reduction is consistent with a quasireversible electron transfer reaction that occurs when the ring nitrogen is protonated. The mechanism is described as an electron transfer or a pre-equilibrium and electron transfer (
).
Formal potential E0'
Thiamine undergoes both oxidation and reduction. The oxidation peak potential occurs at 0.60 V vs NHE. For the reduction of thiamine pyrophosphate, the peak occurs at −0.38 V vs NHE.
Vitamin B1: summary comments on electrochemistry
Vitamin B1 undergoes distinct oxidation and reduction processes as found voltammetrically. The oxidation mechanism is where the following chemical reaction is rapid. No peak current is found on the return sweep to negative potentials. The anodic peak for the oxidation is observed at ∼ 0.60 V vs NHE. For the reduction of thiamine pyrophosphate, a quasireversible electron transfer is observed where the average of the cathodic and anodic peak potentials Eave is −0.38 V vs NHE. The voltammetric response diminishes as the solution is made alkaline, but the Eave value is invariant with pH. The reduction process about −0.38 V vs NHE is best described as an electron transfer,
.
Vitamin B2 - Riboflavin
Chemical characteristics of riboflavin are listed in Table VII. The main structure is a tricyclic heterocycle isoalloxazine modified with a ribityl side chain, shown in Figure 2g.
Table VII. Chemical Characteristics of Vitamin B2.3,26,27,33
IUPAC Chemical Name | 7,8-dimethyl-10-[(2S,3S,4R)-2,3,4, | |
5-tetrahydroxypentyl]benzo[g]pteridine-2,4-dione | ||
Alternative Names | Vitamin G | |
Chemical Formula | C17H20N4O6 | |
Molecular Weight (g mol− 1) | 376.369 | |
Solid at room temp | Yellow or yellow orange solid; slight odor, bitter | |
log P | −1.46 | |
Melting Point (![]() |
280![]() ![]() |
|
pKa | 6.97 (strongest acidic); -0.76 (strongest basic) | |
Physiological Charge | −1 | |
Solubility in Water | 84.7 mg/L of water | |
Solubility in lipids/fats | Slightly soluble in cyclohexanol, amyl acetate and benzyl alcohol, phenol Insoluble in ether, chloroform, acetone, benzene | |
Chemical Class | Flavins (Pterdines and derivatives) | 53 |
The earliest report of the water soluble yellow green fluorescent pigment now known as riboflavin and found in milk, dates to 1879. Bleyer and Kallmann concentrated the pigment and determined some of its properties. In 1933, Richard Kuhn et al., successfully isolated riboflavin. The term riboflavin was set in 1937.54
Action in humans and signs of deficiency
Riboflavin functions as a coenzyme. The active forms are flavin mononucleotide (FMN) and flavin adenine dinucleotide (FAD). FMN and FAD are cofactors in a variety of flavoprotein enzyme reactions in sugar, protein, and fat metabolism. Specifically, FAD participates in redox reactions in the electron transport chain. B2 promotes growth and cell regeneration.55 Vitamin B2 serves as an electron transfer mediator. Riboflavin is essential for healthy skin, nails, hair, and general good health that includes regulating thyroid activity. There is no upper intake level for vitamin B2, partially because it is less soluble than the other B vitamins. Vitamin B2 is found in eggs, green vegetables, milk, and meat.
Riboflavin deficiency is marked by inflammation of the corners of the mouth (chelosis) and the mucosa of the mouth (angular stomatitis), ocular symptoms, and visual impairment. Riboflavin intake during pregnancy is important to prevent birth defects.
Voltammetry and electrochemistry
The electrochemical behavior of vitamin B2 is complicated as represented in an interconnected square scheme mechanism (Scheme 2). Similar schemes are known for quinones.56 There are three oxidation states: the fully oxidized flavoquinone (Flox, vitamin B2), flavosemiquinone radical (Fl. −) and the two electron reduced flavohydroquinone (Fl2 −red), each with three states of protonation. Scheme 2 outlines the square scheme for vitamin B2 in water. In aprotic solvents, lower degrees of protonation are common. Voltammetry of vitamin B2 has been evaluated in water and aprotic solvents.
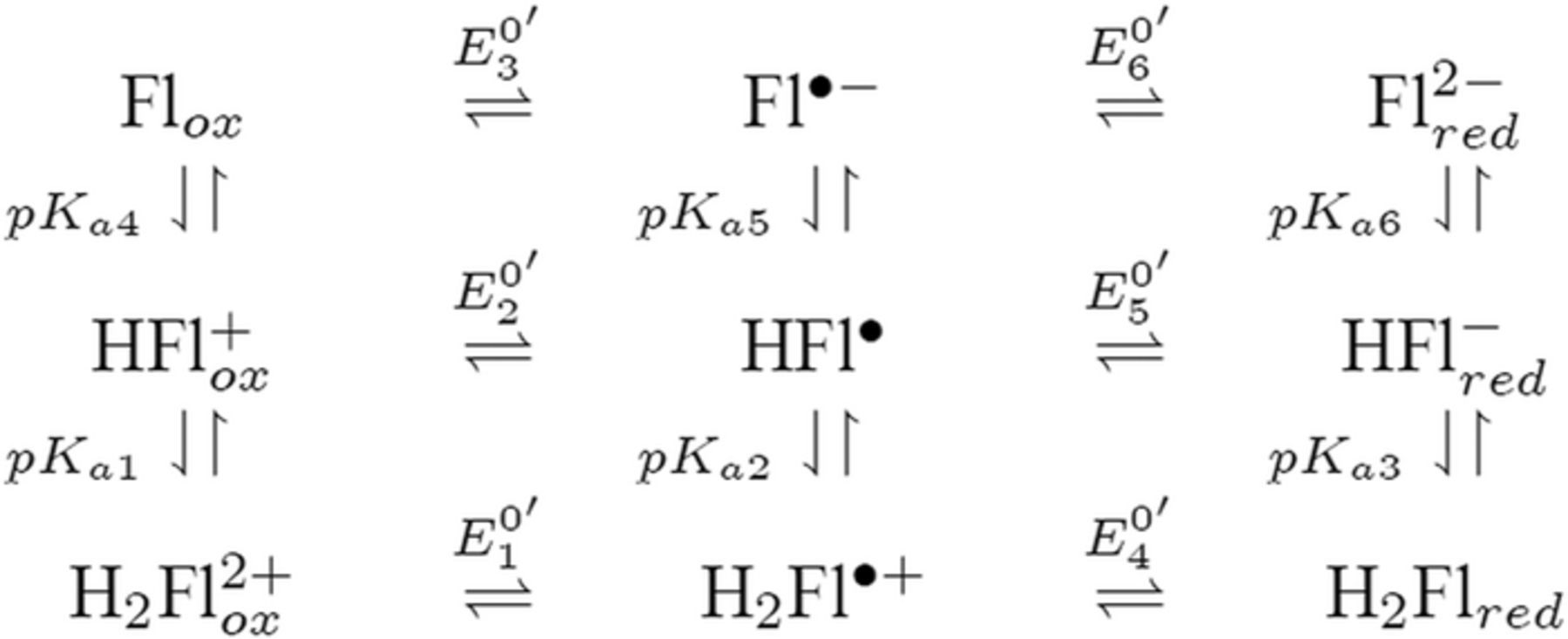
Scheme 2. Proposed flavin species conversion in aqueous solution.57
Aprotic solvents
Voltammetry in aprotic organic media allows access to potentials outside the potential window of water. The experiments shown in Figure 7 used a platinum disk working electrode, platinum auxiliary electrode, and silver wire quasireference electrode in dimethyl sulfoxide (DMSO) with tetrabutylammonium hexafluorophosphate as the supporting electrolyte. Vitamin B2 has a higher solubility in DMSO than the other common organic electrochemical solvents. Ferrocene was used as the internal potential standard. Webster et al. assigned the peaks based on scan rate analysis as well as controlled potential electrolysis (CPE), UV vis, and electron paramagnetic resonance (EPR) spectroscopy. Simulation of an mechanism fit well with the experimental data. The related formal potentials in DMSO are listed in Reference 57: Flox/Fl. −rad at −0.54 V; Fl. −rad/Flrad2 − at −1.30 V; FlH.rad/FlredH − at −0.37 V and Fl− .ox/Flrad2 − . at −0.94 V vs NHE. In Reference 57, additional parameters such as electron transfer rates and equilibrium constants associated with Scheme 2 are also reported. Vitamin B2 undergoes a reversible net one electron reduction. The reduction is not direct but results from a comproportionation reaction between the two electron reduction product and the reactant to form Fl−rad, assigned wave 1 in Figure 7.
Figure 7. Cyclic voltammograms of the reduction of 1 mM riboflavin at a 1 mm diameter planar Pt electrode in dimethyl sulfoxide with 0.5 M nBu4NPF6 at scan rate 0.100 V s− 1. Scan range (a) from −1.6 V to −0.5 V vs Fc|Fc+ and (b) −2.2 V to −0.5 V vs Fc|Fc+.57 Permission from ACS.
Aqueous solution
Electrochemistry of riboflavin in aqueous solution has been studied under various conditions of electrodes, electrolytes, pH, and buffer solutions.58–62 The apparent mechanism for the reduction of vitamin B2 in aqueous solution is a two electron, two proton process, where formal potential is dependent on proton concentration (pH).
Most studies recognized the electron transfer process for B2 as reversible or quasireversible, consistent with . Voltammetry of B2 at various electrodes and various pH are summarized in Table VIII. The general pattern of peak splittings in Table VIII is less than 60 mV with some as small as 30 mV. For reversible electron transfer, ΔEpeak ∼ 0.059V/n.4 Voltammetry of B2 in water is consistent with a two electron, two proton reduction as shown in Scheme 3.
Table VIII. Vitamin B2 Voltammetry at Various Electrodes Yields E0' = Eave.
Electrode | E0' vs NHE at pH | Mechanism | Reference |
---|---|---|---|
Bi film | − 0.07 V vs NHE at pH = 4.0 | ![]() |
58 |
GCE | − 0.20 V vs NHE at pH = 6.8 | ![]() |
59 |
BDD | 0.04 V vs NHE at pH = 2 | ![]() |
60 |
MnO2 CPE | ![]() |
![]() |
61 |
IL + C | ![]() |
![]() |
62 |
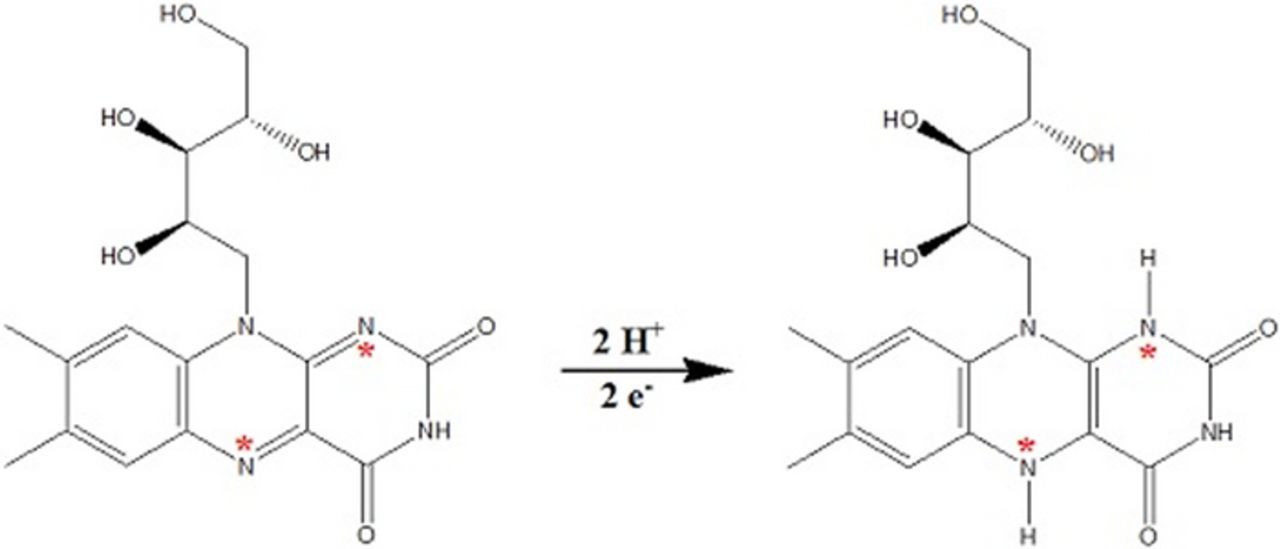
Scheme 3. Possible mechanism for Reduction of vitamin B2.62
Formal potential E0'
In Table VIII, reported formal potentials are noted for vitamin B2 in water at several electrodes and different values of pH. The potentials are linear with pH as E0' = −(0.050 ± 0.004)pH + (0.14 ± 0.01) with R2 = 0.98. The data suggest a ratio of 1 proton per 1 electron in the reaction. The estimated formal potential at pH 0 is +0.14 ± 0.01 V vs NHE. Near physiological pH 7, the formal potential is −0.21 V vs NHE for the two electron reduction of vitamin B2. In Scheme 2, this corresponds to the pathway between Flox and FlredH2. It is noted that at higher pH, riboflavin may degrade in ambient light.62
In aprotic DMSO, the degree of protonation differs from that in water, as shown in Scheme 2.
B2: Summary comments on electrochemistry
In general, the mechanism for the vitamin B2 in aprotic solution is an process for cyclic voltammograms recorded between −0.5 and −1.6 V vs Fc |Fc+ (+0.2 and −0.9 V vs NHE). For larger potential windows, additional electron transfer and chemical processes are observed.57 In aqueous solution a quasireversible or reversible electron transfer
is more applicable with a two electron, two proton. The formal potential at physiological pH is −0.21 V vs NHE.
Vitamin B3 - Niacin
Niacin was first described in 1873 by Hugo Weidel when he oxidized nicotine with nitric acid.65 Casimir Funk specified the term vital amines or vitamines on identification of the amine group on niacin.66 In 1937, Conrad Elvehjem extracted niacin from liver67 and later found niacin effective against pellagra. Tom Spies identified nicotinic acid (Figure 2b) as the agent effective against pellagra.68 The word niacin is derived from nicotinic acid and vitamin.69
Action in humans and signs of deficiency
Niacin includes both nicotinic acid and nicotinamide. Niacin is a precursor for NAD, nicotinamide adenine dinucleotide. NAD can be phosphorylated to form NADP, nicotinamide adenine dinucleotide phosphate. NAD and NADP can be reduced to form NADH and NADPH. NAD/NADH, and NADP/NADPH are principle redox mediators in cellular metabolism. Ingestion of niacin can induce a harmless and characteristic niacin flush.
Symptoms of B3 insufficiency include dermatological and digestive symptoms as well as dementia. Mild deficiencies impact metabolism with increased sensitivity to cold and can yield psychiatric symptoms of fatigue, poor concentration, and anxiety. Pellagra, characterized by dementia, dermatitis, and diarrhea is treated by administration of niacin. Nicotinic acid has also been tried clinically to lower serum cholesterol.
Voltammetry and electrochemistry
The electrochemistry of nicotinic acid is complicated by its acid base chemistry. The acid dissociation and base dissociation constants for nicotinic acid are 2.79 and 4.19, which correspond to the deprotonation of the carboxylic acid and the protonation of the pyridine nitrogen. On dissolution of nicotinic acid in water, the zwitterion is formed as the carboxylic acid proton dissociates and the pyridine is protonated. For HA the zwitterion form, H2A+ the fully protonated form where both carboxylate and pyridinium are protonated, and A− the fully deprotonated form, the fractional concentrations for nicotinic acid with pH are shown in Figure 8. The several protonated forms of nicotinic acid shift formal potentials and complicates interpretation of kinetic mechanisms. Thermodynamics are often mapped by square schemes that include dissociation constants and formal potentials. In Figure 8, the dominant species at pH = 1 is H2A+, and at pH = pKa1 = 2.79, the concentrations of HA and H2A+ are equal. For the majority of cases summarized in Table IX, the dominant species is the zwitterion ion, HA, prevalent at physiological pH.
Figure 8. Fractional concentrations in water for forms of nicotinic acid with pH; pKa1 = 2.79 (carboxylic acid) and pKa2 = 9.81 (pyridinium). The zwitterionic form HA (solid) is dominant at physiological pH, where the carboxylic acid is deprotonated and the pyridinium is protonated. At pH below 4, substantial fractions of H2A+ (dot) are observed. The pyridinium A− is shown as dashed.
Table IX. Chemical Characteristics of Vitamin B3.3,26,27,33
IUPAC Chemical Name | Pyridine-3-carboxylic acid | |
Alternative Names | Nicotinic acid; Niacin; Pyridine-3-carboxylic acid | |
Chemical Formula | C6H5NO2 | |
Molecular Weight (g mol− 1) | 123.11 | |
Solid at room temp | Odorless, white crystalline powder; mild acid taste | |
log P | 0.36 | |
Density (g/cm3) | 1.473 | |
Melting Point (![]() |
236.6 | |
Boiling Point (![]() |
decomposes | |
Flash Point (![]() ![]() |
193![]() ![]() |
|
pKa | 2.79a | |
pKb | 4.19 | |
Physiological Charge | 0 | |
Solubility in Water | 18 g/L of water at 25 °C slightly soluble alcohols ( ∼ 1 g/60 mL) | 63 |
Solubility in lipids/fats | insoluble | |
Stability | stable in air | |
Chemical Class | carboxylic acid | |
Biological half life | 20 to 45 minutes |
asimilar to values for isonicotinic acid 1.75 and 4.9.64
pH dependent behavior of nicotinic acid is mapped by differential pulse voltammetry (DPV) at a glassy carbon electrode (GCE) in phosphoric acid buffers.70 The peak potential Ep, DPV varies linearly with pH for 2.5 < pH < 10 as Ep, DPV vs NHE = − (0.67 ± 0.02)pH − (0.82 ± 0.02) with R2 = 0.992. In this range where the zwitterion is dominant, one proton is transferred per electron. At pH < 5, a second set of Ep, DPV is sometimes observed where Ep, DPV is pH invariant at −1.20 V vs NHE.
Voltammetry and polarography
Lingane and Davis71 note that the first report of nicotinic acid reduction was made polarographically by Shikata and Tachi72 in 1927, where the Ep1/2 = −1.6 V vs SCE (−1.36 vs NHE) at pH = 8.4 in sodium bicarbonate and the reduction was chemically irreversible. Lingane and Davis71 report Ep1/2 values for nicotinic acid at a dropping mercury electrode (DME) that shift with pH: in phosphate buffer at pH = 7.2 (−1.56 V vs SCE; −1.32 V vs NHE), in tetramethyl ammonium borate buffer pH = 9.0 (−1.6 V vs SCE; −1.36 vs NHE), and in unbuffered 0.1 N KCl (−1.7 V vs SCE; −1.46 vs NHE) for the nicotinate). At pH = 5.8 in biphthalate buffer, hydrogen evolution masks nicotinic acid polarography. In strongly alkaline solution, no polarographic response is observed.71,72
The early works of Shikata and Tachi and Lingane and Davis were undertaken at mercury electrodes and demonstrate the pH dependence of nicotinic acid reduction as summarized in Table X by Ep1/2 values. Reduction of nicotinic acid at a mercury pool electrode in degassed electrolyte at pH = 5.1 yields Ep1/2 of −1.47 V vs SCE (−1.23 vs NHE).73 At a hanging mercury drop, Ep1/2 of −1.5 V vs SCE (−1.26 vs NHE) is found at pH = 7.25.74
Table X. Summary of Voltammetric and Polarographic Results for Nicotinic Acid Reduction.
Electrode–-electrolyte; ref. method | E1/2 (V vs NHE)a estimated | References |
---|---|---|
Hg (DME)–-NaHCO3 (aq); SCE; Polarography | − 1.4 V = Ep1/2 at pH = 8.4 | 72 |
Hg (DME)–-0.1 M buffer (aq); SCE; Polarography | − 1.32 V = Ep1/2 at pH = 7.2 | 71 |
− 1.4 V = Ep1/2 at pH = 9.0 | ||
− 1.5 V = Ep1/2 in 0.1 M KCl | ||
+ nicotinate | ||
Hg pool–-acetate buffer (aq); SCE; linear sweep | − 1.23 V = Ep1/2 at pH = 5.1 | 73 |
Hg (DME)–-phosphoric acetic buffer; SCE; DPP | − 1.3 V = Ep, DPP at pH = 7.25 | 74 |
BDD–-HCl (aq); Ag|AgCl; CVb | − 1.22 V = Ep/2 at pH = 1 | 75 |
− 1.60 V = Ep/2 at pH = 2 | ||
− 1.75 V = Ep/2 at pH = 3 |
aPotentials measured vs references of Ag|AgCl and saturated calomel electrode (SCE) are converted to the normal hydrogen electrode (NHE) as ENHE = EAg|AgCl + 0.197 V; ENHE = ESCE + 0.2412 V. bEp/2 are reported for CV as the reduction wave is close to the solvent background so that Ep was more difficult to estimate. See Table AI for abbreviations.
The formal potentials for nicotinic acid are sufficiently extreme that nicotinic acid reduction is not accessible under conditions where hydrogen evolution is facile. For example, cyclic voltammetry at a platinum electrode in a nitrogen degassed nicotinic acid in KCl electrolyte yields no voltammetric signal in the range from +0.3 to +0.8 V vs Ag|AgCl.76 Electrodes such as mercury and boron doped diamond have large overpotentials for hydrogen evolution and allow access to nicotinic acid electrochemistry.
Santos and Rangel75 report cyclic voltammetric responses at boron doped diamond (BDD) for nicotinic acid as a function of pH as shown in Figure 9. The voltammetric morphologies are typical of literature reports. Reductions waves are observed at potentials negative of − 1 V vs Ag|AgCl. On the return positive sweep, no corresponding oxidation wave is observed. This is consistent with an electron transfer followed by a chemical reaction that consumes the reduction product (). The data also illustrate the pH dependence. As proton concentration increases, nicotinic acid is more readily reduced as indicated by the shift of potential at half maximum current to more positive potentials and the sharper increase in current magnitude with potential.
Figure 9. Cyclic voltammograms for 2 mM nicotinic acid in aqueous HCl at a 3 mm boron doped diamond electrode at 0.100 V s− 1 with Ag|AgCl reference. The reduction waves at potentials < −1 V vs Ag|AgCl are characteristic of nicotinic acid voltammetry. The oxidation waves near 2 V are likely due to electrolysis of the electrolyte.75
In voltammetric studies where nicotinic acid reduction is accessible, the general pattern for voltammetry is a reduction at negative potentials that is chemically irreversible and pH dependent. In cyclic voltammetry, no oxidation wave is observed on the return sweep, consistent with a chemical step following reduction. Reported results vary with electrode material and pH. Several mechanisms have been reviewed for carboxylic acid isomers of isonicotinic acid73 for nicotinic acid reduction. A dimerization on mercury has been suggested.74 Comparison of dimerization and catalytic hydrogen generation by nicotinic acid has been made,70 although no clear evidence of catalysis is found in reported cyclic voltammograms. Some mechanistic studies may have been complicated by concomitant hydrogen evolution.
From cyclic voltammetric data, the mechanism for nicotinic acid reduction is summarized as an electroreduction at negative potentials (−1.3 to −1.4 V vs NHE at physiological pH), followed by a rapid chemical reaction of the reduced form to a product that is not electroactive in the water potential window. The reduction of nicotinic acid involves protons because reduction occurs at less extreme potentials as proton concentration increases. The general classification of nicotinic acid reduction is , likely
, and proton dependent.
Oxidation of B3 on 1.6 mm gold disk is reported77 for air saturated saline phosphate buffers (1.5 ⩽ pH ⩽ 5.2). On an oxidative cyclic voltammetric scan from 0.0 to 0.8 V vs SCE, the oxidation peak is pH invariant at 0.75 ± 0.02 V vs NHE and characteristic of a solution species (peak current ip plotted versus square root of scan rate, v1/2 is linear). For the return cathodic scan, the peak potential of a gaussian wave (characteristic of adsorbed reactant, ip versus v linear) varies with pH as Ep vs NHE = −(0.0620 ± 0.009)pH + (0.79 ± 0.03). Note, this voltammetric morphology is similar to that reported for oxygen on gold78 and about the standard potential of 0.70 V vs NHE for the two electron, two proton reduction of oxygen. No similar oxidation processes are observed on Hg and BDD.
Formal potential E0'
No reports of standard or formal potentials were found for nicotinic acid. Crudely estimated from the voltammetric and polarographic Ep1/2 data in Table X, a formal potential of about −1.4 V vs NHE is appropriate for 7 ≲ pH ≲ 9. At lower pH, formal potential shifts positive.
Vitamin B3: summary comments on electrochemistry
Nicotinic acid, Vitamin B3, undergoes proton dependent reduction at negative potentials. The reduction is followed by a chemical reaction that consumes the reduced nicotinic acid and no oxidation wave is observed. The general mechanistic class for nicotinic acid reduction is . The formal potential is estimated as −1.4 V vs NHE about physiological pH.
Vitamin B5 - Pantothenic Acid
Pantothenic acid was discovered in 1933 by Roger J. Williams.79 The D isomer of pantothenic acid is bioactive as vitamin B5. The structure is shown in Figure 2e and the common chemical characteristics are listed in Table XI. In commercial formulations, the more stable provitamin panthenol is often used.
Table XI. Chemical Characteristics of Vitamin B5.3,26,27,33
IUPAC Chemical Name | 3-(2,4-dihydroxy-3, |
3-dimethylbutanamido)propanoic acid | |
Alternative Names | Vitamin B5, calcium pantothenate, dexol, pantothenic acid, zinc pantothenate |
Chemical Formula | C9H17NO5 |
Molecular Weight (g mol− 1) | 219.235 |
Solid at room temp | Yellow, viscous oil |
log P | −1.1 to −1.4 |
pKa | 4.35 |
pKb | −2.8 |
Physiological Charge | −1 |
Solubility in Water | l 1000 mg/mL of water at 25 °C |
Soluble in ethyl ether, ethyl acetate, dioxane, glacial acetic acid | |
Insoluble in benzene and chloroform | |
Solubility in lipids/fats | insoluble |
Stability | stable in air |
Chemical Class | carboxylic acid |
Action in humans and signs of deficiency
Vitamin B5 is needed to form coenzyme-A (CoA), important in metabolism through fatty acid oxidation and cellular respiration. The vitamin also has a role in manufacturing red blood cells and sex and stress hormones. Deficiency is rare but can induce fatigue, insomnia, depression, irritability, vomiting, stomach pains, burning feet, and upper respiratory infections.80
Voltammetry and electrochemistry
Few electrochemical studies of vitamin B5 (pantothenic acid) are reported. In the two studies presented here, D-panthenol was used as the analyte, not pantothenic acid. D-panthenol is the alcohol analog of pantothenic acid (vitamin B5) used in electrochemical studies because D-panthenol is more stable in aqueous solution. D-panthenol is reported as biologically active as pantothenic acid.81
Reduction
Wang and Tseng utilized carbon paste electrodes (CPE) modified with cobalt oxide catalyst to characterize D-panthenol in phosphate buffer at pH 6.08 by cyclic voltammetry, differential pulse voltammetry, and linear sweep voltammetry.82 The cyclic voltammetric curves are consistent with an electron transfer followed by a chemical reaction because no wave is observed on the reverse sweep. From the linear sweep voltammograms, the reduction E1/2 is near −0.4 V vs Ag|AgCl (−0.2 V vs NHE).81
Oxidation
Oxidation of D-panthenol was undertaken by Nayak and Shetti at unmodified glassy carbon electrodes in phosphate buffer for a range of pH. Voltammograms recorded at pH 4.2 are shown in Figure 10, where no wave is observed on the reverse sweep across a range of scan rates. Absence of a corresponding reduction wave is consistent with a following chemical reaction identified by the authors as two electrons, two protons.82 Plots of peak potential vs pH indicated the number of electrons and protons in the oxidation process are equal. For 7.0 ⩽ pH ⩽ 9.2, peak potential varies linearly with pH, following the equation Ep = 0.049pH + 0.686 (R2 = 0.990).82
Figure 10. Cyclic voltammograms of 1.0 mM D-panthenol in pH 4.2 buffer solution. Peak currents increase with scan rate where the scan rates are 0.006, 0.02, 0.03, 0.05, 0.07, 0.09, and 0.1 V/s.82 Permission from Springer.
Formal potential E0'
From linear sweep data from Wang and Tseng, the reduction of D-panthenol occurs at −0.4 V vs Ag|AgCl (−0.2 V vs NHE), and is an electron transfer followed by an irreversible chemical step.81 Nayak and Shetti report that oxidation occurs at approximately 0.32 V vs Ag|AgCl (0.52 V vs NHE) at pH 7.82 Ep for the forward scan for each serves as diagnostic for estimation of E1/2, as −0.2 V vs NHE for the reduction and 0.5 V vs NHE for the oxidation at pH 7.
Vitamin B5: summary comments on electrochemistry
Electrochemistry of pantothenic acid (vitamin B5) has been evaluated by voltammetry of its provitamin D-panthenol, which is both more stable in aqueous media and as biologically active as pantothenic acid. The reduction occurs at −0.2 V vs NHE with a following chemical reaction (). The oxidation occurs near 0.5 V vs NHE and also undergoes a following reaction (
). The reduction of D-panthenol occurs as a two electron, two proton reduction at −0.2 V vs NHE at pH 6.08 and is a diffusion controlled, electrochemically irreversible process. Oxidation occurs at approximately 0.52 V vs NHE and is a two electron, two proton transfer.
Vitamin B6 − Pyridoxine
The effect of vitamin B6 on dermatitis was first observed by Paul György in 193485 while studying skin disease in rats. Vitamin B6 represents six structures that readily interconvert. Pyridoxine (PN) was discovered first.86 Related structures include pyridoxamine and pyridoxal, where the CH2OH at position 4 of pyridoxine is replaced with either CH2NH2 or C=O, respectively.87 The structure of PN is shown in Figure 2h. The biologically active form pyridoxal 5'-phosphate (PLP) is most similar to pyridoxal but CH2PO4H2 replaces CH2OH at the 5 position. The chemical characteristics of Vitamin B6 are listed in Table XII.
Table XII. Chemical Characteristics of Vitamin B6.3,26,27,33
IUPAC Chemical Name | 4,5-bis(hydroxymethyl)-2- | |
methylpyridin-3-ol | ||
Alternative Names | Pyridoxine | |
Chemical Formula | C8H11NO3 | |
Molecular Weight (g mol− 1) | 169.18 | |
Solid at room temp | ||
Density (g/cm3) | 1.4 | |
Melting Point (oC) | 159–162 | |
Boiling Point (oC) | Sublimes | 83 |
pKa | 9.63 (Predicted) | 84 |
pKb | 5.01 (Predicted) | 84 |
Physiological Charge | 0 | |
Solubility in Water | 28 g/L of water | |
Solubility in lipids/fats | insoluble | |
Stability | stable in air | |
Chemical Class | carboxylic acid | |
Biological half life | 20 to 45 minutes |
Action in humans and signs of deficiency
In humans, vitamin B6 is involved in normal cell growth, cell function and development. Further, B6 plays a role in over 100 enzyme catalyzed reactions.88 In the body, pyridoxine is oxidized to pyridoxal-5'-phosphate, the metabolically active form of vitamin B6. Commercially available supplements commonly contain pyridoxine hydrochloride.
The clinical manifestations of vitamin B6 deficiency include microcytic anemia (too few and too small red blood cells), dermatitis, glossitis (inflammation of the tongue), and weakened immune function.1 Vitamin B6 is in vegetables and protein rich foods.89
Voltammetry and electrochemistry
Of the six related forms of vitamin B6, PN electrochemistry is described here.
Cyclic voltammograms recorded for 2 mM PN in CH3CN, DMF, and DMSO with 0.2 M nBu4NPF6 are shown in Figure 11.88 A chemically irreversible oxidation is observed near +0.5 V vs Fc|Fc+ (1.123 V vs. NHE). For pyridoxine oxidation in DMF and DMSO, peak potentials lie close to the solvent potential limit and are poorly resolved; pyridoxine oxidation in CH3CN is well resolved. The product of pyridoxine oxidation undergoes a chemical reaction and a negligible wave for reduction is observed on the return cathodic sweep. In all solvents an independent electrochemical process is observed, a chemically reversible reduction centered around −1.5 V vs Fc|Fc+ (−0.9 V vs NHE).
Figure 11. Cyclic voltammetry of 2 mM pyridoxine and nBu4NPF6 in CH3CN, DMF, and DMSO. Data are recorded at a 1 mm platinum disk at 0.1 V/s versus a Fc|Fc+ Reference 88. Data Provided by Richard Webster.
Variable scan rate studies were conducted by Webster and coworkers to investigate the chemical step that follows the initial pyridoxine oxidation around +0.5 V vs Fc|Fc+. See Figure 12 where both the first and second cycles are shown. The initial oxidation has a peak at +0.8 V vs Fc|Fc+ (1.4 V vs NHE) that is chemically irreversible. The normalized peak height of (i/ν)1/2 is fairly constant with scan rate ν. It is noted that as the scan rate slows, the wave shifts negative toward the starting potential. The shift can arise as the oxidation product is consumed, with larger shifts as more product reacts away at slower scan rates. A second, small reduction wave is observed at the end of the first cycle with a peak at −0.7 V vs Fc|Fc+ (1.4 V vs NHE). At the start of the second cycle, the reverse of this process, the oxidation is observed with a peak near 0.6 V vs Fc|Fc+ The smaller wave is chemically reversible, centered near −0.65 V vs Fc|Fc+ and coupled to the oxidation near +0.8 V vs Fc|Fc+ (1.42 V vs NHE). The coupled redox process suggests an mechanism for the oxidation of pyridoxine.
Figure 12. Cyclic voltammetry of 2 mM pyridoxine in CH3CN and nBu4NPF6 at a 1 mm platinum disk as a function of scan rate. The voltage range is narrower than in Figure 11. The current is normalized by ν1/2. The first two scans are shown where the initial scan is shown as a solid line and the second as a dotted line. The invariance of (i/v)1/2 across scan rates is consistent with a diffusional component to the kinetics.88 Data provided by Richard Webster.
Formal potential E0'
Pitre and coworkers report an E1/2 for pyridoxine at −1.7 V vs SCE (−1.46 V vs NHE) in Britton-Robinson buffer at pH = 6.5,5 measured by direct current polarography at a Hg drop. The polarogram is described as representing a reversible, one electron transfer process.
Webster and coworkers report the cyclic voltammetry of pyridoxine in acetonitrile at a platinum disk.88 Here, E1/2 for the first electron step is estimated to be 0.5 V vs Fc|Fc+ (1.12 V vs NHE). The E1/2 for the second electron transfer step is estimated to be −1.4 V vs Fc|Fc+ (−0.8 V vs NHE).
Vitamin B6: summary comments on electrochemistry
In organic solvents, vitamin B6 undergoes a one electron oxidation followed by a chemical step, and then a second electron transfer step (an mechanism). In an independent process, Vitamin B6 undergoes a one electron reduction without significant chemical reaction (
). Both voltammetry and polarography are reported for vitamin B6. For the oxidation of vitamin B6, the E1/2 for the first electron transfer step was estimated to be 0.5 V vs Fc |Fc+ (1.1 V vs NHE). For the reduction of vitamin B6, the E1/2 was estimated as −1.4 V vs Fc|Fc+ (−0.8 V vs NHE). Little voltammetry of vitamin B6 is reported in water as the redox processes lie near the edge of the water potential window.
Vitamin B7 - Biotin
Biotin was co-discovered by Dean Burk and Paul György c.a. 1934.93 Because biotin serves as a strongly bound ligand for the protein avidin, biotin avidin linkages are used to couple proteins to electrodes. György demonstrated that biotin avidin complexation in egg whites depleted biotin bioavailability. Biotin, B7 chemical characteristics are in Table XIII. The structure is in Figure 2c.
Table XIII. Chemical Characteristics of Vitamin B7.3,26,27,33
IUPAC Chemical Name | 5-[(3aS,4S,6aR)-2-oxo- | |
1,3,3a,4,6,6a-hexahydrothieno[3,4-d]imidazol-4-yl]pentanoic acid | ||
Alternative Names | Biotin | |
Chemical Formula | C10H16N2O3S | |
Molecular Weight (g mol− 1) | 244.32 | 90 |
Solid at room temp | ||
Density (g/cm3) | 1.3 | 90 |
Melting Point (![]() |
224-226 | 91 |
Boiling Point (![]() |
574 (predicted) | 90 |
pKa | 4.4 (predicted) | 92 |
pKb | −1.9 (predicted) | 92 |
Physiological Charge | −1 | |
Solubility in Water | 36.6 mg/L of water | 92 |
Stability | stable in air | |
Chemical Class | carboxylic acid |
Action in humans and signs of deficiency
Vitamin B7 acts as a cofactor for carboxylases through the catalysis of various steps in major metabolic pathways (e.g., amino acid catabolism, gluconeogenesis, lipogenesis) in mitochondria and the cytoplasm. Moreover, B7 functions in the regulation of gene expression and in cell signaling pathways. Deficiencies in B7 lead to dermatological and neurological problems that include delays in growth and development.94 Food sources of B7 include meats, cereals, and vegetables.89
Voltammetry and electrochemistry
Webster and coworkers94 investigated voltammetry of biotin in DMF and DMSO and reported anodic to cathodic peak-to-peak separations for B7 that are substantially larger than for a reversible one electron transfer process; the peak potential splittings are larger than 0.350 V. Webster and coworkers identify biotin reduction as a slow (irreversible) heterogeneous electron transfer . One electron is transferred in the complete reduction of biotin. Further evidence of a one electron reduction was reported by Serna et al.95 and Pitre et al.5
Cyclic voltammetric data are shown in Figure 13 for biotin in DMF at a platinum disk. The Ag|AgCl miniature reference electrode used in DMF does not provide a true reference; potentials are reported relative to an internal ferrocene reference (Fc|Fc+). In the top portion of the Figure, as scan rate increases, the current and peak splitting increases while the area under the curves for the forward and reverse sweeps remain equal. This is consistent with mechanism , an electron transfer and no significant chemical steps.
Figure 13. Cyclic voltammograms for 2 mM biotin in DMF with 0.2 M nBu4NPF6 at a 1 mm platinum electrode at scan rates from 0.1 to 20 V s− 1. Potentials are reported relative to an internal Fc|Fc+ reference. Overlayed cyclic voltammograms are shown in (a) for scan rates of 0.1, 0.2, 0.5, 1,2, 5, 10, and 20 V s− 1. Stacked cyclic voltammograms are shown in (b) after normalization of the current by the ν1/2.94 Data provided by Richard Webster.
In related studies, cyclic voltammograms recorded on planar glassy carbon electrodes in DMF and DMSO yield no signal.94 Platinum is electrocatalytic for biotin reduction. Data for platinum and glassy carbon electrodes in DMF and DMSO are presented in Figure 14. Webster and coworkers suggest dihydrogen is formed from either the reduction of two biotin molecules or from the dimerization of adsorbed hydrogen atoms on the platinum surface.
Figure 14. Cyclic voltammograms for 2 mM biotin and 0.2 M Bu4NPF6 in DMF (a) and DMSO (b) 1 mm platinum disk (solid) and 1 mm glassy carbon disk (dotted). CVs were recorded at 0.100 V s− 1 with a platinum counter and Ag|AgCl miniature reference electrode. Potentials are reported relative to an internal Fc |Fc+ reference. A one electron process was observed on the Pt electrode for both solvents. No voltammetry was observed on the glassy carbon electrode.94 Data provided by Richard Webster.
Formal potential E0'
Webster and coworkers reported the formal reduction potential of B7 as −1.6 V vs Fc| Fc+ in DMF and −1.8 V vs Fc|Fc+ in DMSO.94 This estimates the formal potential as between −0.9 and −1.1 V vs NHE.
Vitamin B7: summary comments on electrochemistry
Cyclic voltammetric studies of biotin in aprotic media (DMSO and DMF) yield electron transfer as the dominant process. Voltammetry is observed at platinum electrodes but not glassy carbon electrodes. This suggests a mechanism for electron transfer dependent on electrocatalytic platinum surface processes that include adsorbed hydrogen. The mechanism for biotin reduction is with cyclic voltammetric peak splitting that increases with scan rate, but an Eave invariant about −1.03 V vs NHE estimates E1/2.
Vitamin B9 - Folic Acid
Folic acid was first discovered and isolated at Lederle Laboratories of the American Cyanamid Company by Herschel K. Mitchell, Esmond E. Snell, and Roger J. Williams in 1941 from several tons of spinach leaves.96 Subsequent research led to the synthesis of anticancer drugs and links between vitamin B9 deficiency and birth defects.97 Chemical properties of vitamin B9 are summarized in Table XIV. The chemical structure of folic acid (Figure 2f) has an unusually high ratio of heteroatoms (13) to carbon (19), C19H19N7O6.
Table XIV. Chemical Characteristics of Vitamin B9.3,26,27,33
IUPAC Chemical Name | (2S)-2-[[4-[(2-amino-4-oxo-1H- | |
pteridin-6-yl)methylamino]benzoyl]amino]pentanedioic acid | ||
Alternative Names | Vitamin B9 | |
Folic acid | ||
Folate | ||
Folvate | ||
Folvite | ||
Pteroylglutamic acid | ||
Vitamin M | ||
Chemical Formula | C19H19N7O6 | |
Molecular Weight (g mol− 1) | 441.404 | |
Solid at room temp | Odorless, tasteless solid; Yellowish-orange crystals | 63 |
Density (g/cm3) | ||
Melting Point (![]() |
250 | |
pKa | 3.5, 4.3 | |
Physiological Charge | −2 | |
Solubility in Water | 1.6 mg/L | |
Solubility in lipids/fats | insoluble | |
Stability | stable in air | |
Chemical Class | Glutamic acid derivatives |
Action in humans and signs of deficiency
Folic acid is important in the stimulation of the hematopoietic system, which includes the organs and tissues responsible for blood production.98 At the cellular level, folic acid congeners are necessary for maintenence of normal erythropoiesis (red blood cells, erythrocytes), synthesis of the nucleic acids purine and thymidylate, interconversion of amino acids, and methylation of tRNA.33
Folic acid deficiency may lead to megaloblastic anemia, where bone marrow produces unusually large, structurally abnormal, immature red blood cells. Other symptoms of B9 deficiency include fatigue, paleness of skin, and lightheadedness.99 Abnormal B9 concentrations have been linked to rheumatoid arthritis, Alzheimer's disease, and fibromyalgia.33
Voltammetry and electrochemistry
Voltammetry of vitamin B9 (folic acid) has been evaluated at a variety of electrodes as strong adsorption of folic acid fouls most electrode surfaces. Adsorption through the benzene ring is reported.100
Reduction of Vitamin B9 is apparent in Figure 15. The gaussian wave morphology characteristic of adsorption in cyclic and linear sweep voltammetry is shown in the several reductions at a hanging mercury drop electrode as reported by Bjornsen and Jacobsen.8 Reliable voltammetric signals were only obtained when the drop was refreshed between voltammetric scans.
Figure 15. Cyclic voltammogram of the reduction of 100 nM folic acid at a hanging mercury drop electrode at a scan rate of 0.020 V s− 1 in seawater with acetate buffer at pH 5.2.9
For oxidation of folic acid, two oxidations at p-tert-butyl-calix[6]arene modified carbon paste electrodes are reported by Vaze and Shrivastava.100 Other modifications of gold electrodes to reduce adsorption are reported.101,102 Yang and Wan employed a gold working electrode modified with a self-assembled 2-mercaptobenzothiazole (MBT/SAM) film. Cyclic voltammograms in a pH 7.4 phosphate buffer solution provided clearly defined anodic and cathodic peaks with potentials at 0.298 V and 0.116 V vs SCE (0.539 V and 0.357 V vs NHE).102 At pH 7.4, Eave estimates E1/2 as 0.21 V vs SCE (0.45 V vs NHE). Some decrease in the peak current on the reverse sweep is noted, suggestive of a slow following reaction.102
Peak potentials are strongly dependent on pH, consistent with coupled electron proton transfer. Several reports note a linear relationship between anodic peak potential and pH.100–103 In general, anodic peak potentials shift negative as pH is increased. For the oxidation, Vaze and Shrivastava regressed peak potentials with pH to yield, Ep = −0.0648pH + 0.902 vs SCE (correlation coefficient, R2 = 0.9996) for pH 2 to 6.100 The near 60 mV / pH slope is consistent with one proton transferred for each electron and the intercept of 0.902 V vs SCE (1.14 V vs NHE) estimates the formal potential at pH = 0.
Formal potential E0'
Reduction of folic acid undergoes at least three separate reductions, as shown in Figure 15. Szczepaniak and Ren report four cyclic voltammetric reduction peaks at a hanging mercury drop electrode in aqueous NaClO4 at pH 4.0: −0.045, −0.55, −0.85, and −1.0 V vs Ag|AgCl (−0.25, −0.35, −0.65, and −0.8 V vs NHE).103 Le Gall and Van den Berg report reduction peaks at −0.5 V, −0.8 V, and −1.2 V vs Ag|AgCl (−0.3, −0.6, and −1.0 V vs NHE) in an acetate buffer (pH 5.2) at a HMDE. They suggest four steps in the reduction: first a two electron reduction to 5,8-dihydrofolic acid at −0.3 V vs NHE, followed by a tautomerization to 7,8-dihydrofolic acid, followed by another two electron reduction to 7,8-dihydro-6-methyl pterin, and a final two electron reduction to 5,6,7,8-tetrahydro-6-methyl pterin. This is an mechanism where each electron transfer is two electrons, two protons. In acidic media, all reductions were chemically irreversible and peak potentials shift negatively with increase in pH.9
Oxidation of folic acid at a multi-walled carbon nanotube modified gold electrode in phosphate buffer at pH 2.5 was reported by Zeng and coworkers to proceed via a two electron process at 0.83 V vs SCE (1.07 V vs NHE) with an estimated standard potential of 0.79 V vs SCE (1.03 V vs NHE). The linear relationship between peak current and scan rate suggests the electrode process is adsorption controlled.101 Two separate oxidation peaks were resolved at p-tert-butyl-calix[6]arene modified carbon paste electrodes by Vaze and Shrivastava at 0.71 V and 0.86 V vs SCE (0.95 V and 1.1 V vs NHE) in pH 5.0 Britton-Robinson (BR) buffer.100 The estimate of E1/2 by Eave yields 0.79 V vs SCE (1.0 V vs NHE) that agreed well. Folic acid oxidation is reported as two electron, two proton, as depicted in Scheme 4.100,104
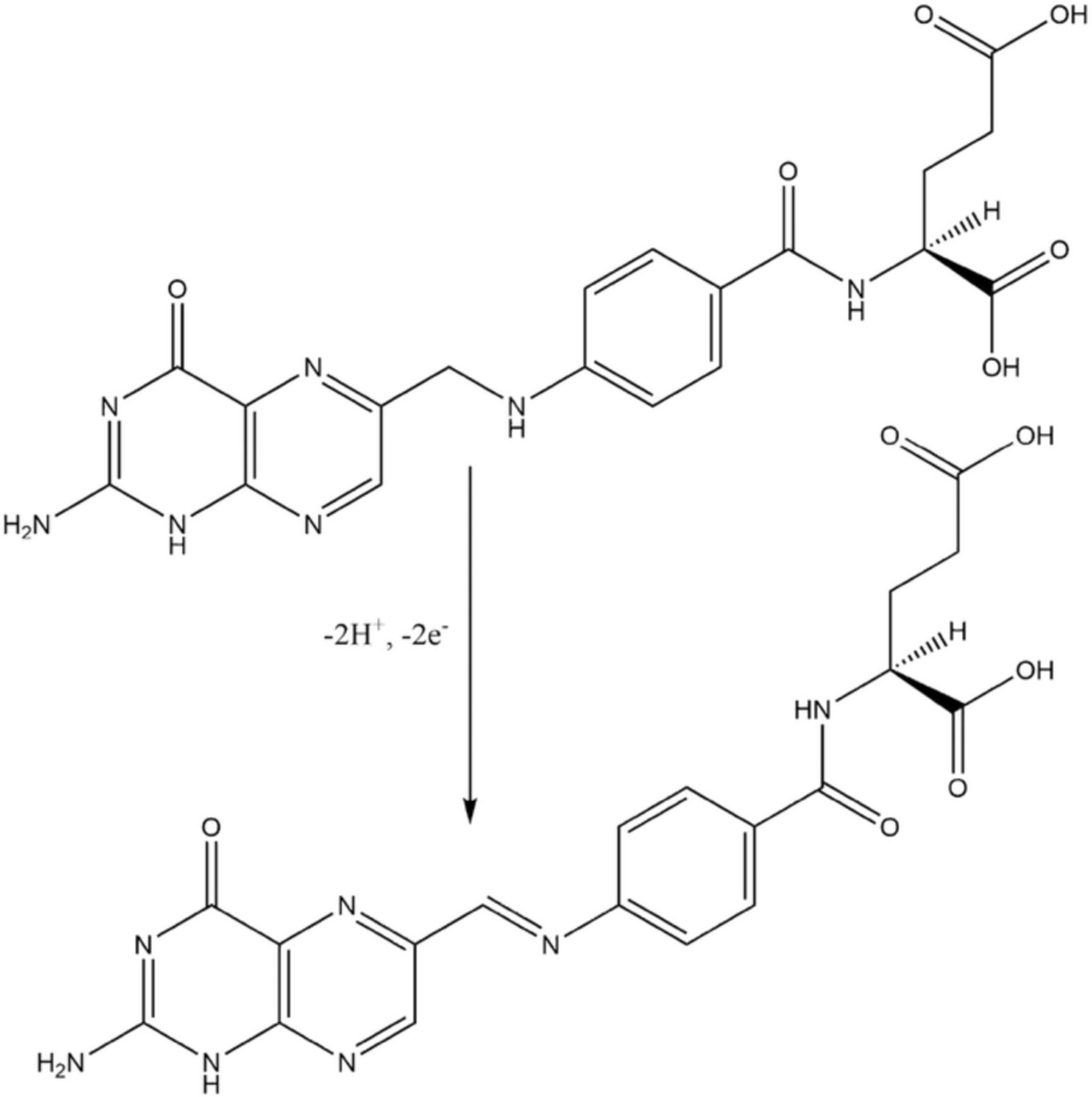
Scheme 4. A proposed mechanism for the two electron, two proton oxidation of folic acid centered at secondary amine between the two rings, which is reported to mimic biological oxidation.100,104
Vitamin B9: summary comments on electrochemistry
Folic acid adsorbs strongly to electrode surfaces. To maintain electrochemical measurement integrity, DMEs and various surface-modified electrodes are needed. Reduction of vitamin B9 yields at least 3, chemically irreversible waves with peak potentials that are pH dependent in an apparent mechanism. Oxidation of folic acid exhibits one or two pH dependent peaks in the vicinity of 1 V vs NHE.
Vitamin B12 - Cobalamin
William P. Murphy, George Whipple, and George Minot shared the 1926 Nobel Prize for liver therapy that cured anemia, a terminal illness at the time. The results of their work found liver fed to anemic patients reduced and cured anemia symptoms. It was later determined that vitamin B12 deficiencies were the cause of pernicious anemia in humans.105 In 1947, American (Karl Folkers (US, Merck)) and British (Alexander Todd (GB)) researchers isolated and determined the chemical nature of B12. For B12,105 the chemical structure is shown in Figure 2i and chemical characteristics are listed in Table XV. Dorothy C. Hodgkin solved the crystal structure of B12 in 1956. Hodgkin won the Nobel Prize for her work on important biochemical substances, which included the most complex structure of all vitamins, B12.106 The name of vitamin B12 varies based on the coordinated ligands because it is a cobalt coordination complex.
Table XV. Chemical Characteristics of Vitamin B12.3,26,27,33
IUPACChemicalName | ||
Cyanocobalamin: cobalt(3+);[(2R,3S,4R)-5-(5,6-dimethylbenzimidazol-1-yl)-4-hydroxy-2-(hydroxymethyl)-oxolan-3-yl][(2R)-1-[3-[(1R,2R,3R,5Z,7S,10Z,12S,13S,17S,18S,19R)-2,13,18-tris(2-amino-2-oxoethyl)-7,12,17-tris(3-amino-3-oxopropyl)-3,5,8,8,13,15,18,19-octamethyl-2,7,12,17-tetrahydro-1H-corrin-24-id-3-yl]propanoylamino]propan-2-yl] phosphate; cyanide; carbanide. | ||
Methylcobalamin: cobalt(3+);[5-(5,6-dimethylbenzimidazol-1-yl)-4-hydroxy-2-(hydroxymethyl)oxolan-3-yl]-1-[3-[(4Z,9Z,14Z)-2,13,18-tris(2-amino-2-oxoethyl)-7,12,17-tris(3-amino-3-oxopropyl)-3,5,8,8,13,15,18,19-octamethyl-2,7,12,17-tetrahydro-1H-corrin-21-id-3-yl]propanoylamino]propan-2-yl phosphate. | ||
Hydroxocobalamin: cobalt(3+);[(2R,3S,4R,5S)-5-(5,6-dimethylbenzimidazol-1-yl)-4-hydroxy-2-(hydroxymethyl)oxolan-3-yl][(2R)-1-[3-[(1R,2R,3R,4Z,7S,9Z,12S,13S,14Z,17S,18S,19R)-2,13,18-tris(2-amino-2-oxoethyl)-7,12,17-tris(3-amino-3-oxopropyl)-3,5,8,8,13,15,18,19-octamethyl-2,7,12,17-tetrahydro-1H-corrin-21-id-3-yl]propanoylamino]propan-2-ylphosphate; hydroxide. | ||
Aquacobalamin: cobalt(3+);[5-(5,6-dimethylbenzimidazol-1-yl)-4-hydroxy-2-(hydroxymethyl)oxolan-3-yl]-1-[3-[(4Z,9Z,14Z)-2,13,18-tris(2-amino-2-oxoethyl)-7,12,17-tris(3-amino-3-oxopropyl)-3,5,8,8,13,15,18,19-octamethyl-2,7,12,17-tetrahydro-1H-corrin-21-id-3-yl]propanoylamino]propan-2-yl phosphate; hydroxide; hydrate. | ||
Formulas | C63H88CoN14O14P; C63H91CoN13O14P; C62H90CoN13O15P; C62H91CoN13O15P | |
MW (g mol− 1) | 1355.38; 1344.40; 1347.38; 1348.93 | |
State at 25![]() |
Solid, dark red-orange crystals or an amorphous or crystalline red-orange powder, tasteless, odorless | |
log P | 3.57 | |
Melting Pt (![]() |
> 300 | |
pKa | 1.84 | 92 |
pKb | 8.77 | 92 |
Physiological Charge | 2 | |
Water Solubility | 12.5 g/L | |
Chemical Class | Octahedral transition metal (Cobalt, Co) complex with 6 total coordination sites when Co(III), 4 coordination sites to a corrin ring, 1 to a dimethylbenzimidazole group (branched off the corrin ring), and 1 which varies (CH−3, CN−, H2O, etc.), this coordination site provides all unique behavior between all B12 forms. |
Action in humans and signs of deficiency
Gut bacteria in humans and other animals synthesize vitamin B12, a vitamin critical for cellular division, growth, and DNA replication. Specifically, methylcobalamin is essential for the function of methionine synthase, an enzyme that catalyzes the synthesis of methionine.46 5-Deoxyadenosylcobalamin is a cofactor for the enzyme involved in the conversion of L-methylmalonyl-coenzyme A to succinyl-coenzyme A.46 The majority of plant species do not produce B12 and although humans can self produce B12 in the colon, self produced B12 is not absorbed because absorption occurs in the ileum above the colon. Animal products or dietary supplements are necessary to avoid B12 deficiencies.107
Vitamin B12 deficiencies result in anemia, peripheral nerve disease (peripheral neuropathy), and eventual death with unresolved deficiency.107 Symptoms of vitamin B12 deficiencies are numerous and include: fatigue, weakness, constipation, loss of appetite, weight loss, difficulty maintaining balance, depression, confusion, dementia, poor memory, and soreness of the mouth or tongue.1
Voltammetry and electrochemistry
Aquocobalamin, with water axially coordinated, displays two one electron reduction waves for the metal center (Co(III) to Co(II) to Co(I)) in Figure 16. One two electron reduction wave is observed (Co(III) to Co(I)) when the axial ligand is a strong base, i.e., a poor leaving group, such as cyanide in cyanocobalamin in Figure 17 and the methyl anion in methylcobalamin in Figure 18. As the electron withdrawing nature of the axial ligand increases, the reduction potential for the B12 derivative shifts negative.109
Figure 16. Cyclic voltammetry of 5 mM aquocobalamin in pH 4 Britton-Robinson buffer at 0.100 V s− 1. A vitreous carbon electrode (dashed) displays an , two electron transfer mechanism while the gold electrode (solid) only displays a one electron transfer as hydrogen evolution dominates after −0.7 V vs. SCE.108 Permission from ACS.
Figure 17. Cyclic voltammetry (80 A scale) of 0.50 mM cyanocobalamin in 0.1 M EDTA, pH 9 at 0.250 V s− 1 (SCE). The dashed line represents run 2 immediately after run 1 (solid line).109
Figure 18. Cyclic voltammetry (200 A scale) of 1.0 mM methylcobalamin in 0.1 M KCl, pH 7 at 0.250 V s− 1 (SCE).109
Formal potential E0'
The measured formal potentials for vitamin B12 in various derivatives are reported in Table XVI. The potentials for aquacobalamin are reported in Table XVI and Figure 16.
Vitamin B12: summary comments on electrochemistry
The three main formal oxidation states of cobalamin: Co(III), Co(II), and Co(I) each display different chemical properties. The Co(III) is electrophilic, Co(II) acts as a radical, and Co(I) is nucleophilic.111 For the reduction of Co(II) to Co(I), the electron transfer slows with increasing axial ligand coordination strength.112 As the nucleophilicity of the axial ligand increases (H2O < OH− < CN− < CH−3), the potential of the primary reduction wave shifts negative.109 Solvent, electron flux (current density), and pH affect the rate of the oxidation-reduction processes of vitamin B12.112–114 At pH 2.8 and higher, the 5,6-dimethylbenzimidazole branch of the cobalamin decouples from an axial positions of the cobalt nucleus.113 The electrochemistry of vitamin B12 derivatives are unique among the water soluble vitamins (). The thermodynamic and kinetic behavior of the electron transfers are largely controlled by the strength of the axially coordinated ligand. To access electrochemical reactions of vitamin B12 in humans, the axial ligand may exchange to a less electron withdrawing ligand so the cobalamin redox potentials fall into a physiologically useful range.
Vitamin A - Retinol
Vitamin A is a lipid soluble vitamin that exists in several structural forms that range from the alcohol retinol through the aldehyde retinal to retinoic acid. β -Carotene is a conjugated, symmetric molecule that serves as the provitamin precursor to vitamin A. The structures of vitamin A in the common retinol and retinal forms along with the provitamin precursor β-carotene are shown in Figure 3a, Figure 3b, and Figure 3c, respectively. The chemical characteristics are noted in Table XVII.
Table XVII. Chemical Characteristics of Vitamin A.3,26,27,33
IUPAC Chemical Name | Retinol:3,7-dimethyl-9-(2,6,6-trimethyl-1-cyclohexen-1-yl)-2,4,6,8-nonatetraen-1-ol, | |
(all-E)-Isomer | ||
Retinal:(2E,4E,6E,8E)-3,7-dimethyl-9-(2,6,6-trimethylcyclohexen-1-yl)nona-2,4,6,8-tetraenal | ||
β-carotene: 1,3,3-trimethyl-2-[(1E,3E,5E,7E,9E,11E,13E,15E,17E)-3,7,12,16-tetramethyl-18-(2,6,6-trimethylcyclohexen-1-yl)octadeca-1,3,5,7,9,11,13,15,17-nonaenyl]cyclohexene | ||
Alternative Names | Retinol, Retinal, all-trans-Retinol, 11-cis-Retinol, Retinoic acid, Vitamin A, Vitamin A1, Aquasol A, alpha-carotene, beta-carotene, gamma-carotene | |
Chemical Formula | Retinol: C20H30O β-carotene: C40H56 Retinal:C20H28O | |
Molecular Weight (g mol− 1) | Retinol: 286.459 β-carotene: 536.888 Retinal: 284.443 | |
Solid at room temp | Retinol: Yellow crystals or orange solid β-carotene: Deep orange crystals Retinal: Solid | |
log P | Retinol: 5.68 β-carotene: 9.72 Retinal: 6.38 | |
Melting Point (![]() |
Retinol and Retinal: 63.5 α-carotene: 187.5 | |
Boiling Point (![]() |
Retinol: 279 to 280° F at 1 × 10− 06 mm Hg (NTP, 1992) 137-138 °C at 1 × 10− 6mm Hg | |
pKa | Retinol: 16.44 | 92 |
pKb | Retinol: -2.2 | 92 |
Physiological Charge | 0 | |
Solubility in Water | Retinol: 0.0076 mg/mL β-carotene: 0.6 mg/mL Retinal: 0.0042 mg/mL | |
Solubility in lipids/fats | Practically insoluble in water or glycerol; soluble in absolute alcohol, methanol, chloroform, ether, fats and oils | |
Stability | stable in air | |
Chemical Class | Organic retanoids and carotenoids |
In 1912, English biochemist Frederick Gowland Hopkins discovered unknown factors in milk important to growth of rats. These factors were not classifiable as fats, carbohydrates, or proteins. In 1917, Elmer McCollum (University of Wisconsin-Madison) and Lafayette Mendel and Thomas Burr Osborne (Yale University) further characterized one of the components. By 1920, these fat soluble factors were called vitamin A. Hopkins was awarded the Nobel Prize for his discovery in 1927.115
Action in humans and signs of deficiency
Vitamin A is essential for proper function of the retina in humans as well as bone growth, reproduction, immunity, and growth and differentiation of epithelial tissue. Retinal in the retina binds to the protein opsin to form the complex rhodopsin. When stimulated by visible light, the retinal component of the rhodopsin complex undergoes isomerization at the 11-position of the double bond to the cis-form; this is reversed in "dark" reactions to return to the native trans configuration. The reversible isomerization of retinal in rhodopsin is critical to color and low light vision.33 Stored trans-retinol is oxidized to trans-retinal which is converted into a biologically active form by retinaldehyde dehydrogenases.
Vitamin A deficiency in humans impacts vision, skin, bone, and immunity.26 Disorders of vitamin A insufficiency include kidney dysfunction and bladder, thyroid, and other cancers.33
Voltammetry and electrochemistry
Reductions of β-carotene and retinal are chemically reversible whereas reduction of retinol is chemically irreversible; all are one electron processes. Oxidation of β-carotene is a chemically reversible, two electron process.116 Oxidation of retinol and retinal are chemically irreversible. Retinol oxidizes to retinal with the transfer of two electrons. Retinal undergoes one electron oxidation.
β-carotene
Voltammetry for β-carotene is shown in Figure 19, where β-carotene oxidation at a platinum electrode in aprotic dichloromethane occurs by a chemically reversible, two electron transfer, such that the oxidations of neutral β-carotene and its product cation radical occur near simultaneously.31 The near simultaneous oxidation steps manifest as the single oxidation peak in cyclic voltammograms.
Figure 19. Cyclic voltammogram of 1x10− 3 M -carotene in CH2Cl2 containing 0.2 M nBu4NPF6 at a 1 mm diameter GCE at a scan rate of 0.1 V/s.31 Data provided by Richard Webster.
Reductions of β-carotene are fast, one electron transfer processes.117. From the cyclic voltammogram in Figure 19, Webster and coworkers report reductions at −2.3 V vs Fc|Fc+ (−1.58 V vs NHE) at a glassy carbon electrode in dichloromethane, which is water immiscible.31 Mairanovsky, et al. report as many as four separate reduction waves at a hanging mercury drop in dimethylformamide (DMF)+benzene (2:1 vol.) where an aqueous SCE reference provides trace water to the water miscible DMF. The peaks of the reduction waves are at −1.68, −1.85, −2.49, and −2.83 V vs SCE (−1.44, −1.61, −2.25, and −2.59 V vs NHE).118 The different voltammetric responses in dichloromethane and DMF are consistent with different water content in miscible and immiscible solvents.
For β-carotene in dichloromethane, the oxidation is a simultaneous process and reduction is an
mechanism. In miscible DMF+benzene with higher proton concentration, more numerous reduction processes are observed.
Retinol
Oxidation of retinol is reported by Wring, et al. at 0.8 V vs SCE (1.04 V vs NHE) in 95% methanol in 0.05 M acetate buffer as shown in Figure 20.119 The oxidation is assigned to the irreversible formation of retinal with the transfer of two electrons.120 Reduction of retinol is reported by Park to be a chemically irreversible, one electron process where the reduction product rapidly passivates the electrode so currents on second scans are markedly diminished.117 Webster and coworkers report two separate one electron reductions by cyclic voltammetry in Figure 22 beginning at −2.57 V vs Fc/Fc+ (−1.95 V vs NHE) at a glassy carbon electrode in acetonitrile; a second reduction is found at −2.13 V vs NHE.121
Figure 20. Cyclic voltammogram of all-trans-retinol in 95% methanol 0.05 M acetate buffer solution (pH 5.0 where a 1:1 acetic acid:acetate buffer in methanol has an effective pH of 6.74) at a glassy carbon electrode at 0.050 V s− 1. 1F and 2F are the first and second forward scans. The decrease in current in 2F is consistent with adsorption products fouling the electrode after the first scan.119
Figure 22. Cyclic voltammograms of 1 × 10− 3 M retinoic acid (2), retinol (3), retinyl palmitate (4), retinyl acetate (5), and retinal (6) (from top to bottom) in CH3CN containing 0.2 M nBu4NPF6 at a GC working electrode at 0.1 V/s.121 Permission from ACS.
Retinal
Oxidation of retinal in tetrahydrofuran (THF) with tetrabutyl ammonium perchlorate (TBAP) as supporting electrolyte is reported by Park to be a chemically irreversible, one electron process.117 In Figure 21, Webster and coworkers report oxidations in dichloromethane for six retinoid species. Retinal is oxidized at 0.68 V vs Fc|Fc+ (1.30 V vs NHE) at glassy carbon electrodes. For retinal and all but β-carotene, the oxidation is followed by a rapid chemical reaction for an mechanism.121
Figure 21. Cyclic voltammograms of 1 × 10− 3 M -carotene (1), retinoic acid (2), retinol (3) retinyl palmitate (4), retinyl acetate (5), and retinal (6) (from top to bottom) in CH2Cl2 that contains 0.2 M nBu4NPF6 at 295 K at 0.1 V/s.121 Permission from ACS.
The reduction of retinal at a glassy carbon electrode in acetonitrile ([H2O] = 50 mM) is shown in Figure 23. For the shorter voltammetric range where Eswitch is −1.8 V vs Fc|Fc+, a chemically reversible voltammetric wave is observed that Webster and coworkers report as a one electron transfer.121 As the potential sweep extends to more negative potentials, a second reduction occurs, followed by a chemical reaction that depletes the concentration of the species labeled Iox so IIox and the subsequent Iox currents are decreased, but a new wave IIIox is observed at more positive potentials. Species IIIox is generated when the scan proceeds past the first reduction process (I) and is related to the oxidation of the second product of reduction (IIred). Webster and coworkers confirmed Ired + Iox, a single electron for the first reduction of retinal by controlled potential coulometry.
Figure 23. Cyclic voltammogram of 2 × 10− 3 M retinal in CH3CN ([H2O] = 50 mM) with 0.2 M nBu4NPF6 at a 1 mm GCE at a scan rate of 0.1 V s− 1.122 Permission from ACS.
Formal potential E0'
The formal potentials for the three forms of vitamin A are considered.
β-carotene
Oxidation peak potential was reported by Webster and coworkers to be 0.16 V vs Fc|Fc+ (0.78 V vs NHE) at a glassy carbon electrode in acetonitrile. This is similar to the value reported by Mairanovsky et al. of 0.61 V vs SCE (0.86 V vs NHE) at a platinum disk electrode in Et4NClO4 in MeCN + benzene (2:1 vol). By simulation, fits to experimental data in dichloromethane, Lui, et al. determined the formal potentials for neutral carotene and the cation radical to be 0.540 V and 0.545 V vs SCE (0.781 V and 0.786 V vs NHE) for quasireversible electron transfers.116 Corresponding heterogeneous rate constants (k0) of 0.009 and 0.0030 ( ± 0.0002) (cm/s) are reported by Jeevarajan and Kispert. k0 was determined by Jeevarajan and Kispert by plotting 1/current vs (rotational rate)− 1/2 for rotating disk experiments. The result from the intercept of which yields 1/ik that determines by ik = nFAk(E)C. Plotting k(E) vs (E − E0) provides the intercept k0 by solving k(E) = k0exp [(1 − α)nF(E − Eo)]/RT.123, 124
Reductions of β-carotene occur at −2.3 V vs Fc|Fc+ (−1.67 V vs NHE) at a glassy carbon electrode in dichloromethane as reported by Webster and coworkers (Figure 19).31 In water miscible DMF+benzene (2:1 vol), Mairanovsky, et al. reports four reductions as noted above.
Retinol: Oxidation of retinol is reported at 0.8 V vs SCE (1.04 V vs NHE) in 95% methanol + 0.05 M acetate buffer (Figure 20).119 Reduction of retinol by cyclic voltammetry yields two, one electron peaks, with sequential peaks reported at −2.57 V vs Fc|Fc+ (−1.95 V vs NHE) and −2.75 V vs Fc|Fc+ (−2.13 V vs NHE) at a glassy carbon electrode in acetonitrile (Figure 22); a second reduction is found at −2.13 V vs NHE.121
Retinal: Retinal is oxidized at 0.68 V vs Fc|Fc+ (1.30 V vs NHE) at glassy carbon electrodes in dichloromethane.121 Retinal oxidation, shown in Figure 23 at a glassy carbon electrode in acetonitrile that contains 50 mM H2O with nBu4NPF6 is −1.75 V vs Fc|Fc+ (−1.08 V vs NHE) for formation of the anion radical and −2.15 V vs Fc|Fc+ (−1.48 V vs NHE) for the subsequent formation of the dianion.122
Vitamin A: summary comments on electrochemistry
β-carotene in water immiscible solvent dichloromethane undergoes chemically reversible oxidation at 0.16 V vs Fc|Fc+ (0.78 V vs NHE), mechanism (Figure 1931). Reduction of β-carotene in dichloromethane, also shown in Figure 19,31 has a peak potential at −2.15 V vs Fc|Fc+ (−1.43 vs NHE) and the reaction is chemically irreversible. In water miscible solvents, more reductions are reported.118
Retinol oxidation is a chemically irreversible process to form retinal at 0.8 V vs SCE (1.04 V vs NHE) in methanol (Figure 20) and similarly at 0.42 V vs Fc|Fc+ (1.04 V vs NHE) in dichloromethane (Figure 21). Reduction of retinol occurs as two separate, one electron steps. The reduction peak potentials for retinol are −2.57 V vs Fc|Fc+ (−1.95 V vs NHE) and −2.75 V vs Fc|Fc+ (−2.13 V vs NHE) at a glassy carbon electrode in acetonitrile (Figure 22).
Retinal oxidation is chemically irreversible at 0.68 V vs Fc|Fc+ (1.30 V vs NHE) in dichloromethane (Figure 21121). The first reduction of retinal in CH3CN ([H2O] = 50 mM) with 0.2 M nBu4NPF6 is chemically reversible (Figure 23122). A second, chemically irreversible reduction occurs at more negative potentials (Figure 23122). Potentials for the formation of the anion radical and subsequent formation of the dianion are Eave = −1.75 V vs Fc|Fc+ (−1.08 V vs NHE) and Ep = -2.15 V vs Fc|Fc+ (−1.48 V vs NHE).
Vitamin D - D3: Cholecalciferol; D2: Ergocalciferol
Two forms of the lipid soluble vitamin D are active in humans, D2 (ergocalciferol) and D3 (cholecalciferol). Vitamin D2 and D3 have similar structures (Figure 3f) and chemical characteristics (Table XVIII).
Table XVIII. Chemical Characteristics of Vitamin D.3,26,27,33
IUPAC Chemical Name | ||
D3:cholecalciferol (1S,3Z)-3-[(2E)-2-[(1R,3aS,7aR)-7a-methyl-1-[(2R)-6-methylheptan-2-yl]-2,3,3a,5,6,7-hexahydro-1H-inden-4-ylidene]ethylidene]-4-methylidenecyclohexan-1-ol D2: ergocalciferol (1S,3Z)-3-[(2E)-2-[(1R,3aS,7aR)-1-[(E,2R,5R)-5,6-dimethylhept-3-en-2-yl]-7a-methyl-2,3,3a,5,6,7-hexahydro-1H-inden-4-ylidene]ethylidene]-4-methylidenecyclohexan-1-ol | ||
Alternative Names | D3: activated 7-dejudrocholestrerol | |
D2: Viosterol | ||
Chemical Formula | D3: C27H44O | |
D2: C28H44O | ||
Molecular Weight | D3: 384.648 | |
(g mol− 1) | D2: 396.659 | |
Solid at room temp | D3: Solid, fine colorless needle crystals or cream colored powder, odorless | |
D2: Solid, odorless white/colorless crystals, metallic or bitter taste | ||
log P | D3: 7.5 | |
D2: 7.3 | ||
Melting Point (![]() |
D3: 84–85°C | |
D2: 116.5°C | ||
D3: Strongest Acidic: pKa = 18.38; pKa = 6.10 | ||
pKa | 125 | |
D2: Strongest Acidic: pKa = 18.38; pKa = 6.35 | ||
pKb | D3: Strongest Basic: pKa = -1.3 | |
D2: Strongest Basic: pKa = -1.3 | ||
Physiological Charge | 0 | |
Solubility in | D3: Practically insoluble in water. 0.00038 mg/mL | |
Water | D2: 50 mg/L 25 deg C; 0.000433 mg/mL | |
Freely soluble in ethanol, methanol and some other organic solvents. | ||
Solubility in lipids/fats | D3: Slightly soluble in vegetable oils. | |
Log Kow=10.2 | ||
Soluble in alcohol, chloroform, ether and fatty | ||
oils. | ||
D2: Solubility in acetone 69.5 g/L, benzene 10 g/L, | ||
hexane 1 g/L.(all in room temperature) | ||
Log Kow=10.44 | ||
Chemical Class | D2: Ergocalciferols |
In 1922, Elmer McCollum found a component in cod liver oil that cured rickets in rats. This "antirickets factor" was later named vitamin D. Adolf Windaus at the University of Göttingen in Germany received the Nobel Prize in chemistry in 1928 for his work on sterols. The work studied the multistep conversion of cholesterol into vitamin D3.126
Action in humans and signs of deficiency
Vitamin D3 (cholecalciferol) is formed from 7-dehydrocholerol present in the skin on reaction with UV light.31 The 7-dehydrocholerolis is converted to calcifediol in the liver and further to calcitriol in kidneys, where both reactions proceed by hydroxylation.31 Vitamin D2 (ergocalciferol) is formed by a mechanism similar to Vitamin D3.127 Once created, vitamin D is carried to various target organs including the intestines and pancreas and to the bone where vitamin D helps maintain calcium homeostasis.128 In the intestines, Vitamin D facilitates absorption of phosphorus as phosphate and dications of calcium, magnesium, and zinc.
Vitamin D facilitates calcium and phosphorus absorption.129 Signs of deficiency are rickets in children and osteomalacia in adults. Rickets is characterized by soft, bent bones and osteomalacia by bone pain and muscle weakness where bones are fragile and may fracture on impact.26 Vitamin D deficiency may also lead to cancer, heart disease, and immune system impairment. Deficiency arises through inadequate sun exposure and diet low in Vitamin D.
Voltammetry and electrochemistry
Voltammetric studies have been undertaken for the two forms of vitamin D, vitamin D2 and D3. Characterization by cyclic voltammetry yields an mechanism of an electron transfer followed by a fast, irreversible chemical step, see Figure 24.125,127,129,130 After the initial cyclic voltammetric sweep, adsorption fouls the electrode and leads to diminution of the current on subsequent sweeps.127,130
Figure 24. Cyclic voltammograms of (a) 1 mM vitamin D3 and (b) 1 mM vitamin D2 recorded at a scan rate of 0.1 V s− 1 at a glassy carbon electrode in CH3CN and CH2Cl2 with 0.2 M nBu4NPF6 at 293 K. Fc|Fc+ is used as internal potential standard. The voltammetric morphology is consistent with an process.127 Data provided by Richard Webster.
Electrochemical analyses used three electrodes: glassy carbon or Pt working electrode, Ag|AgCl reference electrode, and a Pt counter electrode. The solvent was either ethanol, acetonitrile (MeCN) or dichloromethane (CH2Cl2) with a supporting electrolyte (LiClO4).125,127,129,130 Ya et al.127 reported cyclic voltammetry of vitamin D. In Figure 24a, voltammetry is shown for vitamin D3; strikingly similar voltammetry for vitamin D2 is shown in Figure 24b. The oxidation peak occurs at 1.08 V vs Fc|Fc+ (1.4–1.5 V vs NHE) in both CH3CN and CH2Cl2 solvents. This is in fair agreement with the formal potential of 1.08 V vs Ag|AgCl (1.28 V vs NHE) found in ethanol by circular dichroism.130
The oxidation mechanism is characterized as oxidation of the triene to the diol. (Scheme 5)125,127,129,130 This is based on a comparison study of cholesterol that is structurally similar to vitamin D, but lacks a triene group and yields no CV signal.125 Square wave voltammetric morphology are the same for vitamin D2 and D3,127 consistent with a common site for oxidation in both vitamins. The product of the chemical oxidation for vitamin D yields an 1H NMR spectrum similarly lacking a double bond between carbons 7 and 8 in Scheme 5, although no diol product was identified.127
Formal potential E0'
The reported formal potential calculated by circular dichroic analysis for Vitamin D2 is 1.28 V vs NHE in ethanol.130 Based on voltammetry for both vitamin D2 and D3 in organic solvents CH3CN and CH2Cl2, the estimated formal potential is 1.4–1.5 V vs NHE.127
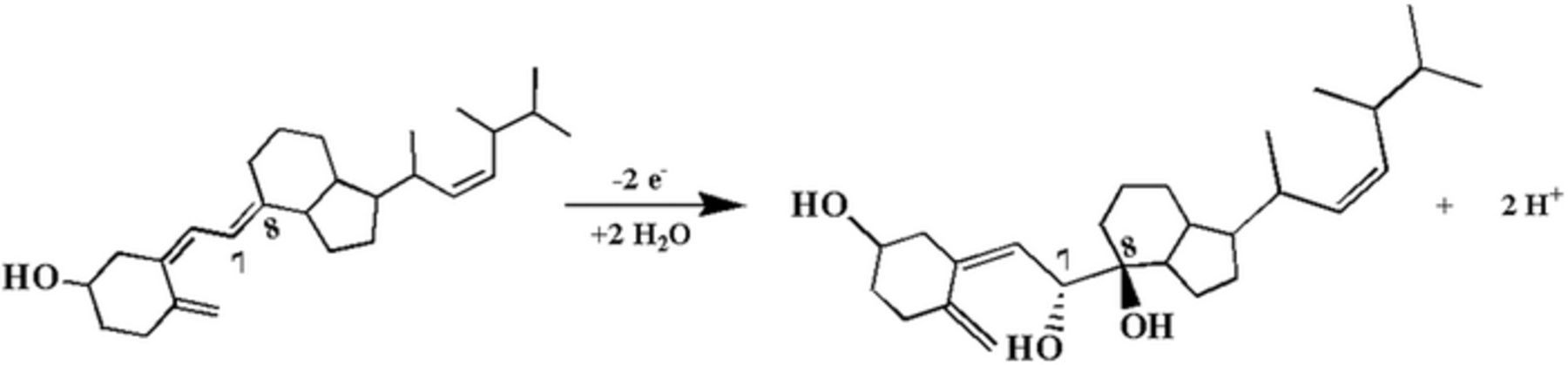
Scheme 5. Proposed mechanism for the oxidation of vitamin D2 where the triene moiety is oxidized to a diol product about the bond between carbons 7 and 8.130
Vitamin D: summary comments on electrochemistry
Vitamin D has two forms: vitamin D3 (cholecalciferol) and vitamin D2 (ergocalciferol). The voltammetry for both forms are similar and follows an mechanism, an oxidation followed by a fast chemical step where no reduction wave is found. The reported oxidation peak values for both vitamin D forms are 1.4 to 1.5 V vs NHE in CH3CN and CH2Cl2 solvents.127 A comparable value in ethanol of 1.28 V vs NHE is found by a circular dichroic method.130 The related proposed mechanism for the oxidation is a two electron transfer reaction.130
Vitamin E - α-Tocopherol
In 1922, Herbert M. Evans and Katherine S. Bishop from the University of California at Berkeley discovered the compound eventually identified as the fifth vitamin E. The compound, referred to as "Factor X", restored fertility in rats on a nutritionally restricted diet. The structure was determined by Erhard Fernholz in 1938131 as shown in Figure 3d. The chemical characteristics are summarized in Table XIX.
Table XIX. Chemical Characteristics of Vitamin E.3,26,27,33
IUPAC Chemical Name | (2R)-2,5,7,8-tetramethyl-2- | |
[(4R,8R)-4,8,12-trimethyltridecyl]-3,4-dihydrochromen-6-ol | ||
Alternative Names | α-Tocopherol | |
Chemical Formula | C29H50O | |
Molecular Weight (g mol− 1) | 430.717 | |
liquid at room temp | Liquid, pale yellow oil | |
log P | 10 | |
Density (g/cm3) | 0.950 | |
Melting Point (![]() |
3 | |
pKa | 10.8 | |
Physiological Charge | 0 | |
Solubility in Water | Insoluble in water, but water dispersable | |
Solubility in lipids/fats | Fat soluble. Soluble in alcohol, ether, acetone, chloroform | 6 |
Action in humans and signs of deficiency
Vitamin E exists in eight natural forms: α-, β-, γ-, and δ-tocopherol, as well as α-alpha, β-beta, γ-gamma, and δ-tocotrienol. α-tocopherol, the only form used by the human body, is an antioxidant. The liver takes up the various forms of vitamin E and secretes only the α conformation. Vitamin E blocks formation of reactive oxygen species formed during oxidation of fat. Vitamin E also affects cell proliferation and differentiation in smooth muscle cells, platelets, and monocytes. α -tocopherol helps regulate blood cell dilation and inhibits platelet aggregation. Vitamin E deficiency in healthy individuals is rare enough that no overt deficiency symptoms are available. Individuals with syndromes like Crohn's diseases and cystic fibrosis require supplemental doses of vitamin E.1
Vitamin E is found in a variety of common foods: sunflower seeds, almonds, peanuts, nuts as well as spinach, broccoli, and raw tomatoes. Natural and synthetic supplements of α-tocopherol are common. RRR-α -tocopherol (a dextro isomer) is the only naturally occurring stereoisomer of α-tocopherol. Laboratory synthesis of α-tocopherol is not stereoselective and produces a racemic mixture of all eight possible stereoisomers. The four dextro isomers of α-tocopherol are active in humans while the four levo isomers are inactive. A person must consume approximately 50% more of the synthetic supplement to equal the dosage of a natural supplement.1
Voltammetry and electrochemistry
α-tocopherol (vitamin E Figure 3d) is a fat-soluble compound found in vivo, typically located in the hydrophobic membranes of cells. For this reason, electrochemistry of vitamin E is commonly performed in aprotic, organic solvents; the absence of water drastically improves the stability of the oxidized forms of vitamin E.6 Cyclic voltammetric experiments by Webster and coworkers demonstrated that the addition of small amounts of water to nonaqueous electrolyte shifted α-tocopherol oxidation to less positive potentials.132
Webster and coworkers undertook detailed mechanistic evaluation of α -tocopherol by cyclic voltammetry at platinum disks in acetonitrile with 0.2 M nBu4NPF6.6 A typical voltammogram is shown in Figure 25, where the mechanism falls into the general class of . Greater detail on the pathway shown is presented in pathway 2 of Scheme 6 by Webster and coworkers.133
Figure 25. Cyclic voltammogram of 1.0 mM -tocopherol in CH3CN containing 0.2 M nBu4NPF6 recorded at a 1 mm Pt disk electrode at 0.1 V s− 1.133 Permission from ACS.
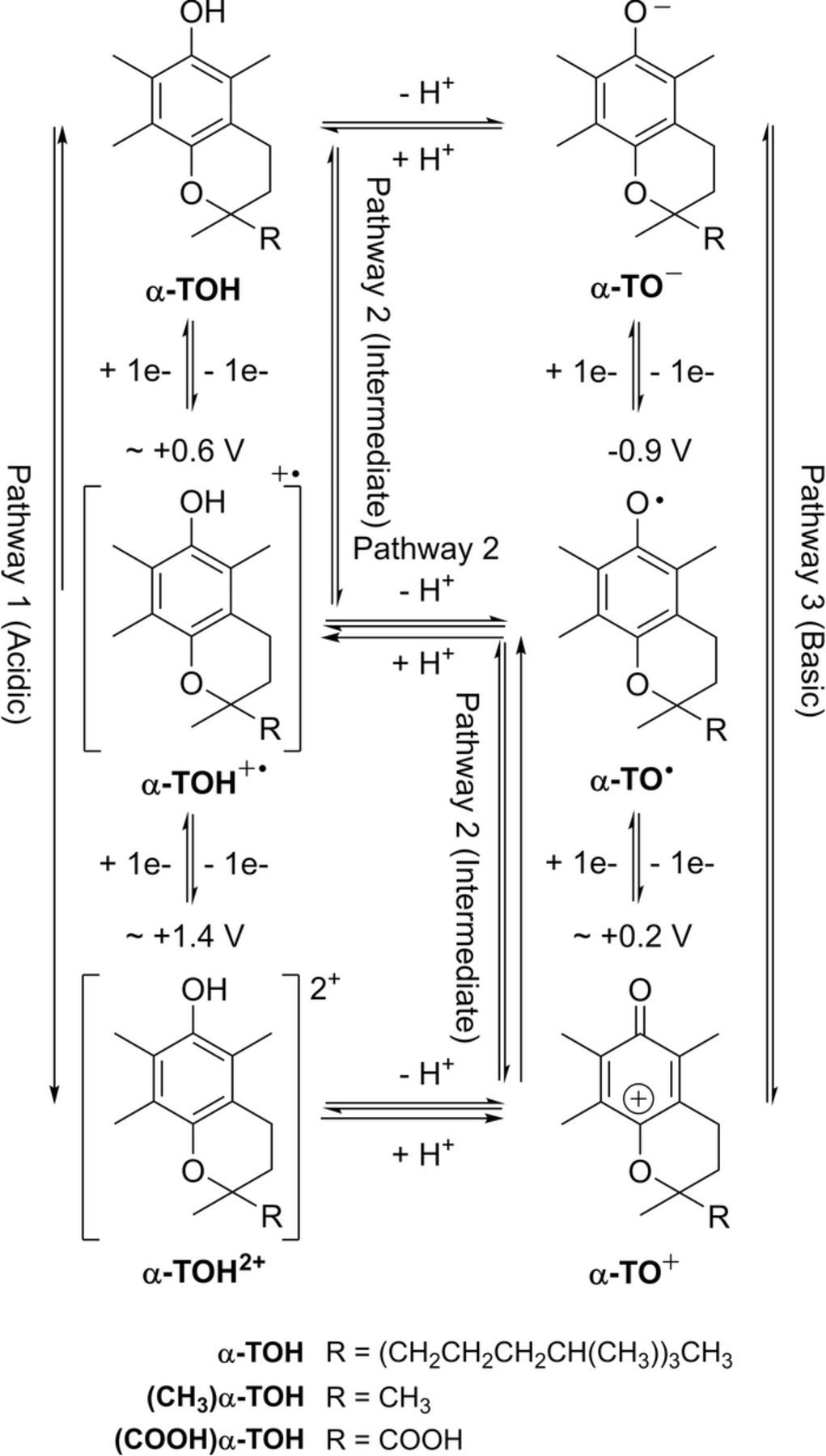
Scheme 6. Pathway for the electrochemically induced transformations of -tocopherol. The approximated potentials necessary to bring about oxidation of the phenolic compounds are listed vs Fc|Fc+, but they do not necessarily correspond to the formal potential. The counterions for the charged species are the supporting electrolyte cation Bu4N+ and anion PF−6, along with the H+ ions.6 Data provided by Richard Webster.
α-TOH is oxidized in a one electron transfer to α-TOH+, a radical which quickly dissociates to the radical α-TO• and H+. The α-TO• radical is immediately oxidized at the electrode as the potential necessary to oxidize the α-TOH is approximately 0.4 V more positive. The voltammogram shows a single process corresponding to a two electron oxidation. A reduction peak is evident as the unusually stable α-TO+ is able to be reduced back to α -TOH.6 Estimation of the half-wave potential E1/2 for the first forward sweep from Ep is not appropriate as the first electron transfer step occurs at a more positive potential than the second, following the transfer of the proton. The potentials of the first and second step lead to a disproportionation. Further, Hauchard and coworkers reported that it was not possible to analyze the separate species of tocols in samples of vitamin E voltammetrically as their potentials are too close to distinguish.134
In Scheme 7, α-TOH represents the unoxidized α -tocopherol undergoing oxidation (Eq. 4), then a chemical step deprotonation (Eq. 5), followed by a second oxidation (Eq. 6), that forms stable α -TO+. α-TO+ cation can be reduced back to α-TOH. As α -TO• radical can undergo a bimolecular reaction with itself, it is evident that the protonation step of the α-TO• radical to α-TOH• radical must be significantly faster than the bimolecular reaction. Voltages were recorded at a platinum disk electrode vs Fc|Fc+ (E0α − TOH= +1.2 V vs NHE, E0α − TO= +0.80 V vs NHE).
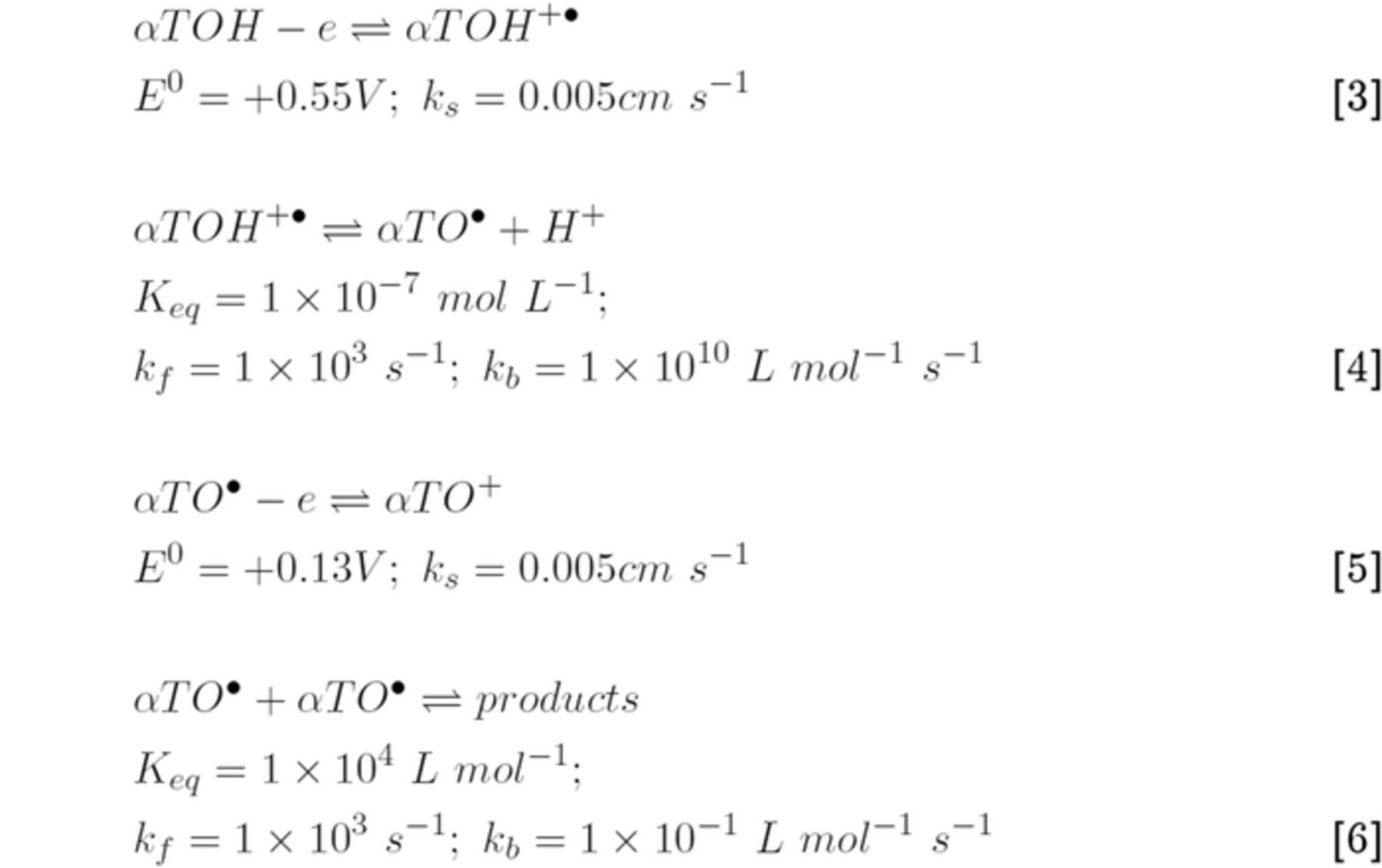
Scheme 7. Equations 3-5 correspond to pathway 2 in Scheme E.6. Listed potentials are vs Fc|Fc+. Kinetic values are derived by computer simulation. Equation 6 represents a bimolecular reaction of the α-TO• radical with itself.133
Formal potential E0'
Webster and coworkers report oxidation potential of α-tocopherol (vitamin E) at 0.5 V vs Fc|Fc+ (+1.12 V vs NHE) at a planar platinum electrode in acetonitrile with 0.2 M Bu4NPF6.6 This value is in good agreement with a second study. The half wave potential for oxidation of α-TOH, as determined by differential pulse voltammetry at platinum ultramicroelectrodes, was reported by Coatanea et al. as 1.04 V vs Ag|AgCl (+1.33 V vs NHE) in N-methyl-2-pyrrolidone (NMP) with 0.2 M nBu4NPF6 supporting electrolyte.134 These experiments were performed in fat/solvent solutions and vegetable oils to develop analytical methods to quantify vitamin E in oils and fats.
Vitamin E: summary comments on electrochemistry
Although vitamin E exists in eight conformations (four tocopherols and four tocotrienols), only α-tocopherol is used by the human body. α-tocopherol undergoes oxidation in a two electron process with chemical steps. With unusual stability, α-TO+ is chemically reversible to the unoxidized α-TOH species. The overall mechanism for the electrochemistry of vitamin E is . α-tocopherol (α-TOH) undergoes an oxidation, then a proton transfer, which is followed by an immediate second oxidation due to the approximately 0.4 V less positive potential for the second oxidation. The resulting cation is unusually stable and can be reduced back to α-TOH. The formal potential for the oxidation is +1.1 V vs NHE in acetonitrile.6
Vitamin K- Phylloquinone and Menaquinone
Lipid soluble Vitamin K was discovered in 1929 by Henrik Dam at the Institute of Biochemistry, Copenhagen University. During dietary experiments on chicks that eliminated cholesterol, unexpected hemorrhages in muscles and other organs as well as delayed coagulation were noted. In the 1930s at St. Louis University, Edward Dousy detailed much of the structure and chemistry of Koagulationsvitamin. Dam and Dousy were awarded the 1943 Nobel Prize in Physiology or Medicine for the discovery of vitamin K.137
Some chemical properties of Vitamin K are listed in Table XX and its structure is shown in Figure 3e.
Table XX. Chemical Characteristics of Vitamin K.3,26,27,33
IUPAC Chemical Name | 2-methyl-3-[(E)-3,7,11,15- | |
tetramethylhexadec-2-enyl]naphthalene-1,4-dione | ||
Alternative Names | Aquamephyton, Konakion, Phyllohydroquinone, Phylloquinone, Phytomenadione, Phytonadione | |
Chemical Formula | C31H46O2 | |
Molecular Weight (g mol− 1) | 450.707 | |
Solid or liquid at room temp | Yellow, viscous oil or solid; odorless | |
Density (g/cm3) | 0.964 | |
Melting Point (![]() |
−20 | |
Boiling Point (![]() |
140-145 | |
Index of Refraction | 1.5263 at 20 ![]() |
|
pKa | 6.67, 8.93 and 11.37 | 135 |
Physiological Charge | 0 | |
Solubility in Water | insoluble | |
Solubility in lipids/fats | soluble | |
Stability | Stable to air and moisture but decomposes in sunlight | 136 |
Chemical Class | metabolite/lipid |
Action in humans and signs of deficiency
The two main functions of vitamin K in humans are control of calcium levels and synthesis of proteins required for blood coagulation. Vitamin K has two natural forms, K1 (phylloquinone) and K2 (menaquinones). Phylloquinone is a redox mediator in photosynthesis that is found in leafy greens. The menaquinones include several compounds, structurally similar with varying numbers of isoprene units in the side chain. Vitamin K2 is produced by bacterial flora in the digestive tract from vitamin K1. Body produced vitamin K is unusable, however, because it is produced below the site of vitamin K absorption in the digestive tract.136 Vitamin K is sparingly stored by the human body and is recycled by a redox process called the vitamin K-epoxide cycle.46 Deficiency symptoms include hemorrhages, coagulation disorders, and malabsorption syndromes as well as adverse impacts on bone and cardiovascular health.136
Voltammetry and electrochemistry
Vitamin K is a naphthoquinone. Quinones typically exhibit voltammetry that varies with available proton. See Scheme 1. In Figure 26, cyclic voltammograms are shown for vitamin K in acetonitrile with low (0.05 M) and high (7.2 M) water content.10 In low water content where few protons are available, vitamin K voltammograms have two well formed and well separated, independent cyclic voltammetric waves. Vitamin K is reduced in two sequential steps, , with high rates of electron transfer. In the high water content acetonitrile, a single voltammetric wave is observed. The wave is chemically reversible as charge on the forward and reverse sweep are comparable and Webster and coworkers report the wave is pH dependent.10,31
Figure 26. Cyclic voltammograms of 1 mM vitamin K in CH3CN with 0.2 M nBu4NPF6 with different water concentration at 1 mm diameter glassy carbon working electrode at a scan rate of 0.1 V s− 1.10 Permission from ACS.
The mechanism is characterized by a square scheme similar to that shown in Scheme 1. The acid dissociation constants listed in Table XX as pKa reflect the impact of protonation of vitamin K before reduction within the square scheme. Each oxidation state of vitamin K may have different pKa values. For low water content acetonitrile, peak splitting ΔEp is approximately 60 mV, consistent with a rapid (reversible) electron transfer process. The first electron process reduces vitamin K to a semiquinone radical anion and the second electron process further reduces the anion radical to the dianion. In high water content acetonitrile, water provides proton and a single wave is observed, where the mechanism is 2 electron/2 proton. The shift in potential with water content is sufficiently reliable, the use of vitamin K to determine trace water content in aprotic solvents is reported.138–140
Webster and coworkers139 investigated the reductions of vitamin K in aprotic solvents, as shown in Figure 27. In aprotic solvents where two waves are observed, the wave about −1.2 V vs Fc |Fc+ (−0.6 V vs NHE) approaches electrochemically reversibility. The second wave is also chemically and electrochemically reversible in DMSO, butylnitrile, MeCN, and DCM. The mechanism is . A second wave is observed in dimethylformamide and dichloroethane, but chemical steps occur. In trichloroethane and propanenitrile, only the wave at −1.2 V vs Fc|Fc+ is observed; in trichloroethane, there is a rapid following reaction.
Figure 27. Cyclic voltammograms of 1 mM vitamin K were recorded at a 1 mm glassy carbon electrode in eight different solvents with a water concentration range of 0.01 to 0.05 M at 0.1 V s− 1 and 22 ± 2°C. The voltammograms are consistent with an mechanism in DMSO, PrCN, MeCN, and DCM. For the remaining solvents, the electron transfer processes have coupled chemical steps.139 Permission from ACS.
Formal potential E0'
The formal potential for vitamin K is highly dependent on the pH. As the water content increases, formal potential shifts positive. From Figure 26, for voltammetry in low water content acetonitrile, the formal potentials for vitamin K in 0.05 M H2O are approximately −1.23 V and −1.79 V vs Fc|Fc+ (approximately, −0.6 and −1.2 V vs NHE). As the water content increases to 7.2 M, the 2 electron 2 proton process consolidates into a single voltammetric wave near −1.1 V vs Fc|Fc+ (−0.5 V vs NHE).10,139
Vitamin K: summary comments on electrochemistry
Vitamin K exhibits electrochemical behavior characteristic of quinones, which are mapped by a proton dependent square scheme. In low water content aprotic solvents, vitamin K is reduced in two distinct reversible electron transfer steps (). The formal potentials are approximately −0.6 and −1.2 V vs NHE. In high water content solvents, a single voltammetric wave at more positive potentials is observed for the coupled two electron two proton reduction of vitamin K. Webster and coworkers report this limits at −0.5 V vs NHE in 7.2 M water.31 Because electrochemistry of the fat soluble vitamin K exhibits strong dependence on water content, the formal potentials in vivo will be highly dependent on local proton concentration.
Summary
Perspective on the electrochemical and voltammetric properties of the 13 vitamins currently identified by the US National Institutes of Health is based on critical review of the literature for each vitamin that is compiled and summarized in Figure 1 and Table I. These properties include formal potential to within about 100 mV near physiological pH 7 and broadly characterized redox mechanisms that include electron transfers (
) and chemical steps (
). The data are compiled for all 13 vitamins in Table I. The formal potentials are plotted along a potential axis as shown in Figure 1. The potential axis provides opportunity to examine relative energies (potentials) for the vitamins, the various mechanisms for the vitamins as well as chemical interactions that include redox cycling of the vitamins, use as antioxidants, stability in aqueous matrices, and chemical interactions between vitamins that degrade vitamins and synergistic actions to promote vitamins efficacy as redox mediators in medical applications.
The major outcomes of this review are:
- All 13 vitamins are electroactive and thus electron transfer agents.
- The formal potentials for the vitamins map between about −1.5 and + 1.5 V vs NHE. Examined across the potential axis in Figure 1, the potentials are fairly uniformly distributed across this range.
- The lipid soluble vitamins A, D, E, and K fall outside the water window. Vitamins A, D, and E can be oxidized and vitamins A and K can be reduced.
- Of the water soluble vitamin C and the eight B vitamins, all have formal potentials within the water window at pH 7 except B3 and B7.
- Vitamins fall into several classes of reactions that to some extend characterize their function and electrochemical (voltammetric) behavior.
- – Vitamins that undergo a single electron transfer process are B3, B7, and B2 that are reduced and C and D that are oxidized. The formal potentials for B2 and C fall within the potential window for water and can serve as sacrificial donors to stabilize species such as other vitamins in solution.
- – Vitamins that can be reduced and oxidized are B6, B1, B9, B5 and E. For the water soluble B vitamins, the potentials deploy on the potential axis as Bred|B—–B|Box so that when Bred and Box are both present, disproportionation serves to recycle the B vitamins back to the native state, B. For lipid soluble E, when present as Ered and Eox, reaction serves to regenerate E and its oxidation product Eox, 2.
- – Two vitamins undergo clean
electron transfers, B12 as aquocobalamin and K, both of which are reduced.
- – The vitamins that exhibit a simple electron transfer step (
) are the reductions of B1, B2, and B7. Retinal (A') and β-carotene (Aβ) undergo oxidations by the
mechanism. Aquocobalamin (B12) and vitamin K undergo well separated but successive electron transfer. There is evidence in the voltammetry of the remainder of the vitamin redox reactions of following chemical reactions.
The potential axis provides a tool to predict vitamin electrochemical stability in moisture, air, acid, and base; to identify vitamins as antioxidants and prooxidants; to identify electrochemical interactions between vitamins; and to identify synergistic effects of vitamin pairs in medical applications. Complex, multistep electron transfers can be charted on the potential axis. The perspective on vitamin redox chemistry developed here and drawn from compiled data taken from critical review of the literature for the individual vitamins may serve as a basis for characterizing yet more complex bioelectrochemical processes.
Acknowledgments
Well done work in the literature aided in compiling the data used to develop perspective on vitamin electrochemistry. The careful work of Richard D. Webster and his group at the Nanyang Technological University, Singapore are most especially appreciated. Professor Webster's sharing of his data is also gratefully acknowledged. The financial assistance of the National Science Foundation (NSF CHE-1309366) is gratefully acknowledged. University of Iowa Graduate College Summer Fellowships for Jacob Lyon and Matt Lovander were instrumental in completion of this CRES3T article.
ORCID
Matthew D. Lovander http://orcid.org/0000-0002-4867-101X
Jacob D. Lyon http://orcid.org/0000-0003-1553-5545
Daniel L. Parr IV http://orcid.org/0000-0003-1738-5106
Junnan Wang http://orcid.org/0000-0001-9445-8685
Brenna Parke http://orcid.org/0000-0003-4159-8726
Johna Leddy http://orcid.org/0000-0001-8373-0452