Abstract
The microchannel heat sink (MCHS) is a robust cooling technique that ensures the efficiency and reliability of compact electronic devices by dissipating a large amount of heat because of its high surface area-to-volume ratio. This study proposes a novel modification of the pin-fins geometry in MCHS, and geometric optimization using response surface methodology (RSM) to build a low thermal resistant MCHS with enhanced heat transfer efficiency with low-pressure drop. Three dimensional numerical simulations using ANSYS FLUENT 2021 R2 are performed on three pin-fins configurations, i.e., MC-BW (pins mounted transversely to the bottom wall), MC-SW (pins mounted transversely to the side wall), and MC-Mixed (pins mounted transversely to the bottom and side wall). The thermal and flow characteristics are investigated using a laminar conjugate heat transfer model at Reynolds numbers 100–1000. Results show that introducing pin-fins significantly enhances heat dissipation as it continuously breaks the boundary layer and generates flow separation downstream of the pin-fins, which enhances fluid mixing and increases heat transfer augmentation inside MCHS. Among different configurations, the MC-Mixed gives the highest improvement of 50% in the convective heat transfer coefficient at Re = 1000. The highest thermal enhancement factor of η = 1.4 is obtained for the MC-Mixed configuration at Re = 600. For the base wall pin fin configuration RSM yields optimized values of 2.50 mm, 0.25 mm, and 0.045 mm for transverse pitch, longitudinal pitch, and diameter of pin respectively, and for the mixed pin fin configuration it gives 1.0 mm, 0.150 mm, 0.035 mm and 1.250 mm values for transverse pitch, longitudinal pitch, diameter of pin and pitch of side wall pins respectively for the maximum heat transfer and minimum pressure drop.
Export citation and abstract BibTeX RIS
Nomenclature
Dh | Hydraulic Diameter |
Hch | Height of Channel |
Wch | Width of Channel |
Havg | Heat Transfer Coefficient |
Nu | Nusselt Number |
Nuo | Nusselt Number of Straight Micro-channel |
f | Friction factor |
fo | The friction factor of a Straight Micro-channel |
η | Thermal Enhancement Factor |
MC-SC | Smooth Microchannel |
MCHS | Microchannel Heat Sink |
MC-SW | Microchannel with side wall pins |
MC-BW | Microchannel with Base wall Pins |
MC-MIXED | Microchannel with both base and side wall pins |
![]() | Pressure Drop |
Rth | Thermal Resistance |
Re | Reynold Number |
RSM | Response Surface Methodology |
MEMS | Micro electro-mechanical systems |
Px | Transverse Pitch |
Py | Longitudinal Pitch |
Pside wall | The pitch of Sidewall Pins |
Dpin | Diameter of Pin |
Havg | Heat Transfer Coefficient |
Ns | Entropy Augmentation Number |
R2 | The coefficient of determination |
1. Introduction
Over the last few decades, there has been a significant advancement in integrated circuit technology. However, better cooling systems are required, as electronic components become more efficient. This is due to the packing density of elements and their thermal power, which dramatically rises as these devices develop rapidly. The extreme temperature rise damages components in these compact devices, and high operating temperatures can significantly shorten component life and reduce reliability. Therefore, controlling their maximum temperature using efficient cooling systems is essential. The microchannel heat sink has emerged as an excellent technique for addressing heat removal issues. Over the years, various techniques have been developed to enhance the thermal performance of microchannel heat sinks. Some of the most widely used approaches include modifying the geometry, increasing surface roughness and turbulence, selecting appropriate materials, and incorporating multi-layer designs. These advancements can lead to more efficient cooling in microchannel heat sinks. In 1981, Tuckerman and Pease [1] proposed microchannel heat sinks (MCHS) to remove heat generated by MEMS. In the early stages of microchannel research, researchers experimented with different channel geometries to improve thermal performance. Weisberg et al [2] used a two-dimensional numerical model to examine temperature fields in fluid and solid substrates, assuming fully developed laminar flow in MCHS. They found that the average heat transfer coefficient and average Nusselt number decreased along the flow due to increased boundary layer thickness.
One method of thermal enhancement in the microchannel is introducing ribs into the channel. Researchers have conducted several studies to analyze the impact of different rib shapes, sizes, and configurations on the performance and efficiency of microchannel heat sinks. Examining these factors aims to understand better heat transfer and fluid flow in microchannel systems with fins and fan-shaped cavities of various shapes and sizes. Akhtar et al [3] studied three configurations of symmetrical ogive-shaped ribs on the walls of the MCHS to enhance their thermal performance with minimum pressure loss. They concluded that the MC-SAWR (Ribs attached on all channel walls.) configuration is the best for better cooling performance as it reduces MCHS thermal resistance by 46% and enhances its Nusselt number by 2.04 times compared to the smooth channel. Ali et al [4] investigated the effect of trefoil-shaped ribs in three different configurations on the thermohydraulic characteristics of MCHS. The highest thermal enhancement factor of 1.6 was obtained for MC-SWTR at Re = 1000. Using CFD, Vatsa et al [5] investigated a microchannel with a two-sided wedge shape at the base. The Nusselt number and the friction factor were analyzed for different wedge angles (3° to 15°) and Reynolds numbers. Thermo-hydraulic performance parameters were evaluated, indicating maximum performance for the 15° wedge angle and minimum for the 3° angle. Dash et al [6] investigated the impact of an extended surface design using cylindrical ribs with variable sector angles on the heat transfer rate in the microchannel. Saha et al [7] studied the right triangular grooves to enhance heat removal from electronic components. The microchannel design incorporates five variable groove angles ranging from 15° to 75°. MCHS, which has a 15° groove angle, demonstrates superior Nusselt number and thermohydraulic performance. The effect of geometrical parameters on the grooved MCHS was studied by Ahmed et al [8]. They obtained the optimal thermal design of MCHS with the Nusselt number enhancement of 51.59% and friction factor improvement of 2.35%. Xu et al [9] numerically investigated the microchannel with dimples. The results show that the average Nusselt number rises by nearly 15% when there are dimples, while the average pressure decreases by approximately 2%. With dimples, the performance factor rises by 2% to 16% with aspect ratios. Chai et al [10] also compared five different offset rib shapes with a straight microchannel and found that the Nusselt number and friction factor were 1.42–1.95 and 1.93–4.57 times higher than that of the smooth channel without ribs. Ahmad et al [11] examined midline ribs in channels. They found that modifying the rib surface with a 45-degree extrusion to form a conical structure helped to reduce pressure loss and improve thermal enhancement. Zhu et al [12] conducted numerical simulations to evaluate the influence of rib shape, size, and arrangement on heat transfer and fluid flow in microchannel heat sinks with fan-shaped cavities to determine the optimal fin shape and setup.
Another method for enhancing the thermal performance of MCHS is by using pin fins of different shapes. Culham and Yovanovich [13] examined the effect of different fin geometries on overall thermal performance and numerically analyzed the thermal performance of pins with square, circular, and elliptical geometries. Entropy generation minimization allowed the combined effect of thermal resistance and pressure drop to be considered simultaneously. Qidwai and Hasan [14] performed a three-dimensional numerical simulation on a rectangular cross-sectional microchannel with cylindrical pin fins by adjusting the pin height along the direction of flow. They showed that thermal performance increased with an increase in pin fin height toward flow. Li et al [15] reported that fin geometry had a greater impact on the thermal and hydraulic performance of MCHS than cavity geometry. In the past, most researchers used ribs on the sidewalls only in the cross-flow direction so that each rib covered the entire channel height. However, in an alternative approach, the ribs were employed at the centerline of the wall. Kadam et al [16] discussed the feasibility of both open-and-closed microchannel heatsinks. However, it is worth noting that open micro-channels have advantages for both single-phase coolant flow and boiling flow. Therefore, the main objective of their work was to identify the heat transfer potential and overall thermal performance of open microchannel heatsinks that consist of square pin profiles and compare the results with the closed microchannel. Soleymani et al [17] studied thermal resistance and mean absolute temperature difference (MATD) in the hotspot zone as performance indicators and examined the effects of various pin-fin configurations and pin-fin angles. They found that rectangular pin-fins with rounded edges performed better thermally than the National Advisory Committee (NACA) airfoils. Sertkaya and Sari [18] studied the impact of axial and radial fins added to a circular pipe at inclination angles of 0°, 30°, 60°, and 90° concerning natural convection. Compared to an unfinned heat exchanger, all finned surfaces and inclination angles result in higher heat transfer capabilities.
Another effective method for improving thermal performance is to optimize the geometry of the microchannel heat sink. Elmi et al [19] conducted a multi-stage optimization of geometric parameters, including the number, height, and diameter of pins, and the transverse pitch, resulting in a 17% improvement in the performance of the pin-fin heat exchanger. Parejo et al [20] used the metaheuristic optimization (FOM) framework to optimize the number of fins, determining an optimal value for diameter to length for a certain number of fins.
Pakrouh et al [21] studied PCM-based pin fin heat sinks numerically, using aluminum for the heat sink's base, fins, and paraffin RT44HC as the PCM (Phase Change Material). Fins were employed to improve thermal conductivity, and using the Taguchi approach, the number of fins, fin height, thickness, and base thickness were optimized. Aly et al [22] optimized the Nu and pressure drop in MCHS using the response surface methodology (RSM). The pin diameter, transverse pitch, and longitudinal pitch were varied within certain bounds, and the numerical values for various geometries were compared to the values predicted by RSM, which showed coefficients of determination of 97.51% and 98.74% for Nu and P, respectively. Deriase et al [23] utilized DOE (Design of Experiments) to identify the ideal independent variables. They employed a collection of statistical techniques called design experts for optimization, with RSM being one methodology used. This procedure examines the response of various independent variables using statistical techniques and reducing entropy generation. The endogenous grid method (EGM) is the most reliable method for heat sink optimization. Furukawa and Yang [24] performed numerical simulations to predict the optimal finned heat sink design in natural convection and showed that the results were close to their EGM predictions. Shih and Liu [25] proposed an EGM method to optimize a finned heat sink for electronic cooling under forced convection. Li et al [26] conducted a performance analysis of the water-cooled microchannel with pin-fin and dimple, which were all inspected. In addition, the pattern search algorithm is used to optimize the geometrical parameters to achieve the best configuration for the best thermal performance. The heat transfer capacity noticeably increased. Additionally, Bhandari and Prajapati [27] presented a comprehensive comparative analysis of different heatsinks to determine the optimal fin height value for improving heat transfer and liquid flow properties.
Due to the increase in the heat flux removal requirements, different techniques have been adopted to remove this heat flux efficiently. These involve active techniques, i.e., vibration, variable roughness, and flow pulsation, and passive techniques, i.e., changing the geometry, ribs, cavities, dimples, pin fins, and secondary flow. Gao et al [28] investigated the thermodynamics of microchannels, with different structures mainly focused on numerical methods. They reviewed different shapes and curvatures of microchannels like rectangular, circular, Trapezoidal, double layered, etc, and adopted different numerical methods for solving governing equations and optimizing data. Ramesh et al [29] reviewed all the possible techniques to enhance the thermal performance of microchannel heat sinks by geometric modifications and jet impingement. They thoroughly studied Nanofluids, flow boiling, and Magneto-hydrodynamics. Bordbar et al [30] conducted a comprehensive review of a study on hydrodynamic features and practical applications of liquid–liquid or gas–liquid slug flow in microchannel. They discussed different methods of numerical simulation that are capable of capturing slug flow, especially the thin liquid film of the carrier liquid around the bubbles or droplets.
Additionally, these modifications are challenging to manufacture due to their small dimensions. Previous studies were mainly focused on enhancing heat transfer by using intricate ribs, cavities, and grooves but all of these modifications increase the pressure drop which increases pumping power requirements. Multi-objective optimization has been analyzed in literature but design parameter optimization using statistical methods like Response Surface Methodology (RSM) is also a significant research gap. Other research gaps include analyzing the unconventional pin fin geometries, the use of advanced materials, and the effect of fluid-structure interaction on microchannel heat sink performance, and also an exploration of fluid flow characteristics phenomena, i.e., flow separation, wake formation, and heat transfer distribution. The integration of microchannel heat sinks with electronic products is a significant research gap that needs to be addressed.
This study proposes a new modification to the MCHS that significantly improves its performance. Full-length pin fins are inserted at the bottom (MC-BW), side channel wall (MC-SW), and both bottom and side channel walls (MC-Mixed). The length of the pin is equal to the channel width in the MC-SW configuration and the channel height in the case of the MC-BW configuration. These full-length pin fins disturb the entire flow throughout the channel, improving heat dissipation inside the MCHS. Response surface methodology (RSM) is also utilized to find the optimal pin fin geometrical parameters that minimize pressure drop while maximizing thermal performance. The diameter of the pin (), transverse pitch (
), and longitudinal pitch (
) are used as independent variables for the optimization of MC-BW configuration. Another parameter, i.e. pitch of the side wall pin fin, is also added for the optimization of MC-MIXED configuration.
The utilization of full-length pin fins for heat transfer augmentation in MCHS and the RSM methodology for performance optimization are unique features of this study. Pin fins modifications are selected because they are simple to manufacture and very effective compared to other geometric modifications [31]. Pin fins are attached to the walls of MCHS to increase the heat transfer in the MCHS by increasing the surface area for heat transfer and generating flow disturbance and mixing in the fluid. The thermal enhancement criterion is used to evaluate the impact of pin fins on the overall hydrothermal performance of the MCHS by comparing the improvement in the Nusselt number with a rise in friction factors. Moreover, the thermal performance of the MCHS with configurations of full-length pin fins is evaluated based on the thermal enhancement factor, pressure drop, friction factor, Nusselt number, thermal resistance, entropy generation rate, and thermal transport efficiency. Also, the flow characteristics of pins fins MCHS are discussed in detail. The objective of this study is to analyze the thermal and flow characteristics of MCHS with a three-pin fin configuration MCHS, pin fins inserted at the bottom (MC-BW), side channel wall (MC-SW), and both bottom and side channel walls (MC-Mixed) using three-dimensional steady laminar flow simulations. Among different pin fin configurations, MC-MIXED is found to be most efficient in terms of heat transfer with the highest thermal enhancement factor of η = 1.4 and nusselt number 2.3 times greater than that of the smooth microchannel, which means it will perform best in terms of heat transfer with reduced pressure losses. Another objective is to find the optimum design parameters for the base and mixed wall configuration of pin fins which provides maximum nusselt number and reduced pressure drop using Response surface methodology.
2. Materials and methods
2.1. Physical model description
The rectangular cross-section MCHS has been chosen for this study as it exhibits the least thermal resistance[32]. The computational domain and geometry of the microchannel heat sink with three pin-fin configurations used are shown in figures 1 and 2. The diameter of the pin is kept constant in all configurations. The geometrical details of the computational domain and pin fins are described in table 1. The dimensions used are consistent with the experimental data of [1]. The thermophysical properties of the solid (copper) and liquid (water) are given in table 2. Constant heat flux has been applied to the base of the MCHS. Cold water enters the MCHS inlet at 293.15K with different velocities corresponding to the Reynolds Number range of 100–1000. All the walls of the MCHS that are exposed to the surroundings are perfectly insulated, except the base wall where the heat flux has been applied.
Figure 1. Computational Domain of MCHS.
Download figure:
Standard image High-resolution imageFigure 2. MCHS Geometry Parameters.
Download figure:
Standard image High-resolution imageTable 1. Physical dimensions [1].
S.no | Symbol and description | Values (mm) |
---|---|---|
1 |
![]() | 1 |
2 |
![]() | 0.25 |
3 |
![]() | 0.035 |
4 |
![]() | 0.35 |
5 |
![]() | 0.5 |
6 |
![]() | 10 |
7 |
![]() | 0.6 |
8 |
![]() | 1.5 |
Table 2. Material properties [1].
Material properties | Copper | Water |
---|---|---|
Density ![]() | 8978 | 998.2 |
Dynamic Viscosity ![]() | — | 0.001003 |
Specific Heat ![]() | 386 | 4184 |
Thermal Conductivity ![]() | 387.6 | 0.6 |
2.2. Assumptions
The following assumptions are made to simplify the numerical modeling. The fluid is considered Newtonian and incompressible with a no-slip boundary condition at the wall. Due to the low Reynold's number, the flow is laminar'[33]. The thermal and physical properties of the solid and fluid domains are considered constant. Heat transfer and flow rate are analyzed under steady-state conditions. Additionally, natural convection and radiation modes of heat transmission are disregarded, and the impact of body force, gravity, and viscous heat dissipation are neglected. Walls are assumed to be smooth to ignore the effects of surface roughness and thermal slip length.
2.3. Governing equations and boundary conditions
The following governing equations have been used for the numerical modeling based on the assumptions discussed earlier.
The continuity equation for steady, incompressible flow [34] is given as

and
are components of fluid velocity in x, y, and z directions, respectively
The momentum equation for steady, incompressible flow [34] is given as

The energy equation for steady, incompressible flow[34] is as follows;

The energy equation in the solid domain is

Where is the velocity at the inlet of the microchannel,
is the density of water,
is the pressure and
Is the specific heat at constant pressure.
The boundary conditions to solve the governing equations for three configurations of MCHS are given in table 3.
Table 3. Boundary condition for MC-BW, MC-SW, MC-Mixed.
Inlet Flow Velocity |
![]() |
Inlet Temperature |
![]() |
Outlet Pressure |
![]() |
Constant Heat Flux at Bottom Wall |
![]() |
At Solid–liquid Interface |
![]() |
![]() | |
Adiabatic wall Boundary |
![]() |
2.3.1. Calculation parameters
The relevant expressions are provided in this subsection to calculate the heat transfer properties and fluid flow in MCHS. The Reynolds number (Re) can be defined as:

is the average or mean velocity of the fluid and
is the dynamic viscosity of the fluid. Where the hydraulic diameter is given as:

The friction factor represents the fluid's pressure loss when interacting with the surface and is used to find the thermal enhancement factor.

Where denotes the whole length of the channel, and ∆p denotes the pressure decrease throughout its length.
The average heat transfer coefficient is calculated as follows:

is heat transfer through the base,
is the area of the base

Average channel wall temperature while
the average temperature of the fluid.
Nusselt number is the ratio of convective heat transfer to conduction at the fluid boundary.

The overall performance of the microchannel is calculated by finding the thermal enhancement factor that defines the ratio of the increase of Nusselt number against an increase in friction factor.

The total thermal resistance is written as,


The average wall temperature is given as follows:

The average base temperature is calculated by:

Average bulk fluid temperature is given by:

Net Total rate of entropy generation:

Entropy generation due to heat transfer:

Entropy generation due to pressure losses:

denotes the total augmented entropy generation rate. This is the ratio of the MCHS's overall volumetric generation rates with various configurations of trefoil ribs.

2.4. Numerical approach
The ANSYS FLUENT 2021 R2 software, based on the finite volume method, is used to conduct three dimensional steady laminar flow numerical simulations. The QUICK and second upwind interpolation schemes solve the diffusive and convective components of the momentum, energy, and continuity equations. Additionally, the SIMPLE algorithm is utilized for the pressure-velocity coupling. The numerical solution is truncated when the residual of momentum, energy, and continuity equations are reduced to 10−6 level.
2.5. Grid independence test
A tetrahedral mesh is created to divide the solid and fluid computational domain into finite elements. Four alternative element sizes are evaluated to determine whether the results depend on the number of elements. For the computational domain with four different element sizes, the temperature distribution profile is plotted along the height of the channel. The number of elements in coarse, medium, fine, and very fine grids is 696673, 760310,948704, and 1008215, as illustrated in table 4. It can be seen that the results using a fine grid (984704 elements) and a very fine grid (1008215 elements) overlap, as shown in figure 3. Since results do not change beyond a fine grid, hence mesh size of 98470 elements is used throughout this study.
Table 4. Grid independence test for MC-SC at Re = 400.
S.no | Grid type | Element size (mm) | Number of elements |
---|---|---|---|
1 | Coarse | 0.058 | 686673 |
2 | Medium | 0.035 | 760310 |
3 | Fine | 0.020 | 948704 |
4 | Very Fine | 0.018 | 1008215 |
Figure 3. Temperature Distribution in Z-X Plane at X = 0. 6mm and Z = 0.25 mm.
Download figure:
Standard image High-resolution image3. Results and discussion
3.1. Validation of model
The Nusselt Number and friction factor obtained from numerical results are compared with the experimental data from Wang et al [35] for validation purposes, as shown in figure 4. The operating conditions, geometrical parameters, and materials used in the simulation are the same as the experimental data. The maximum error between the current numerical simulation and experimental data for the Nusselt number and friction factor is 0.85% and 0.95% respectively. It can be seen clearly that numerical results are in good agreement with the experimental results.
Figure 4. Validation of friction factor and Nu with Re.
Download figure:
Standard image High-resolution image3.2. Characteristics of fluid flow
When pin fins are added to the walls of MCHS, they act as barriers to the flow, which results in flow separation. The pressure difference between the high-pressure region upstream of the fin and the low-pressure region downstream causes wake formation. This wake formation creates regions of low velocity and increases turbulence, as shown in figure 5. Vortices are shed periodically in the wake region when the fluid flows past the pin. In the wake zone, vortices increase mixing, generate turbulence, and improve heat transmission in the microchannel. Vortices and flow recirculation regions increase the convective heat transfer characteristics, causing more heat dissipation from the heated surface.
Figure 5. Enhanced velocity streamlines depicting wake formation.
Download figure:
Standard image High-resolution imageFigure 6 displays the velocity contours of various pin-fin configurations. When the MC-SC is used, a fully developed boundary layer forms. When pin-fins are introduced in the microchannel, the boundary layer is disrupted before it is fully developed. The pin-fins disturb the flow dynamics, preventing the boundary layer from reaching its fully developed state. This disruption can affect heat transfer and flow behavior within the microchannel.
Figure 6. Velocity contours at Re = 400.
Download figure:
Standard image High-resolution image3.3. Characteristics of pressure drop
Figure 7 illustrates the pressure distribution within the channel for different MCHS configurations. A stagnation zone is visible upstream of the pin fins, and a low-pressure zone is evident downstream, forming a recirculation zone. The inclusion of pin-fins configuration results in a higher pressure drop as the fluid encounters a flow restriction.
Figure 7. Pressure distribution in MCHS.
Download figure:
Standard image High-resolution imageMixed pins increase the turbulence in the MCHS, increasing the energy dissipation in the fluid, thus leading to non-uniform flow distribution across the microchannel heat sink surface. The non-uniform flow distribution forms stagnant zones where the fluid velocity is lower and has a higher resistance to flow, leading to a higher pressure drop across the microchannel heat sink. Figure 8 displays the pressure drop for different MCHS configurations at varying Reynolds numbers. As the velocity increases, the wall shear stress increases, requiring more pressure to overcome it, leading to an increasing trend in pressure drop with the Reynolds number. Figure 8 demonstrates that the MCHS with pin-fin configurations has a higher pressure drop than MC-SC because of additional irreversible pressure losses due to wake formation downstream of the pin-fins and frictional losses. MC-MIXED has the highest pressure drop at all Reynolds numbers because the presence of pin-fins on both sides and the bottom of the channel wall creates more disturbance in flow.
Figure 8. Pressure drop(Pa) versus Re.
Download figure:
Standard image High-resolution imageIn an MCHS with pin fins, wall shear stress increases due to increased flow obstruction. Because of pin-fins, more interaction occurs between the fluid and the channel surface, which increases wall shear stress on the MCHS surface. Figure 9 shows the correlation between the Reynolds number range and wall shear for different microchannel pin-fin arrangements and a smooth microchannel (MC-SC). The results indicate that wall shear increases with an increase in Reynolds number. The smooth microchannel exhibits the lowest wall shear since there are no pins present to obstruct fluid movement. On the other hand, the MC-MIXED configuration has the highest wall shear due to pins on both the side and base walls of the microchannel, which restrict fluid passage and reduce its velocity along the flow direction. Among the pin-fin configurations, MC-SW exhibits the lowest wall shear, whereas MC-BW has a lower wall shear than MC-MIXED but a higher wall shear than the MC-SW configuration.
Figure 9. Wall shear versus Re.
Download figure:
Standard image High-resolution imageFigure 10 shows the relationship between the Reynolds number and Darcy friction coefficient [36] for various pin configurations and smooth channels. The graph indicates that the friction coefficient slightly increases in smooth channels as the Reynolds number decreases. This occurs because the velocity of the fluid is increasing, leading to a decrease in friction factor. Conversely, the highest increase in friction coefficient with the lowest Reynolds number occurs when pins are configured with their side walls. The friction factor is highest for all pin-fin configurations at Re = 200. Among different configurations, the MC-MIXED configuration exhibits the highest increase in friction factor with Re due to the presence of more pins contributing to the friction in the microchannel compared to other configurations. In contrast, the MC-SW has the lowest increase in friction factor with Re compared to other pin-fin configurations.
Figure 10. Friction factor versus Re.
Download figure:
Standard image High-resolution image3.4. Characteristics of heat transfer
This study aims to propose an efficient microchannel heat sink (MCHS) that can effectively dissipate heat from the channel. Figure 11 demonstrates that the MC-SC has a high temperature and non-uniform temperature distribution compared to pin-fin configurations due to its low Nu and high thermal resistance. On the other hand, MCHS with pin-fin configurations shows a lower temperature and a more uniform temperature distribution, as it dissipates a large amount of heat to the fluid due to low thermal resistance for convection. Non-uniform temperature distribution induces thermal stresses in both the MCHS and the host chip, harming electronic chip safety and integrity. By employing MCHS with pin-fin configurations, not only can a superior cooling performance be achieved by dissipating a large amount of heat from the electronic chip, but it also promotes the safe operation of these electronic chips by eliminating thermal stresses and high-temperature hot spots.
Figure 11. Temperature distribution in MCHS.
Download figure:
Standard image High-resolution imageHeat transfer effectiveness between the channel walls and fluid through convection is commonly measured using the Nusselt Number, a dimensionless parameter. Figure 12 depicts the relationship between the Nusselt Number (Nu) and the Reynolds number (Re). As the graph shows, the pin-fin configuration MCHS employs on its channel walls leads to a higher Nusselt number due to improved fluid mixing and flow disturbance. Furthermore, the convection heat transfer coefficient improves at higher flow velocities, increasing Nu with Re. Among the various pin-fin configurations, MC-SC exhibits the smallest increase in Nusselt number with Reynolds number. This is because of boundary layer development, which causes a decrease in local Nusselt numbers.
Figure 12. Nusselt number (Nu) versus Re.
Download figure:
Standard image High-resolution imageOn the other hand, the highest increase in Nusselt number is observed in the MC-MIXED configuration. As a result, MC-MIXED offers the highest convective heat transfer and can remove the most heat from the channel compared to the other configurations discussed. Meanwhile, MC-SW shows the lowest increase in Nusselt number with an increase in Re among the different pin-fin configurations.
Figure 13 illustrates the correlation between thermal resistance and Reynolds number. As thermal resistance declines, the rate of heat transfer increases. The mixed pins in MCHS increase the fluid turbulence, which breaks down the boundary layer and increases the mixing between the fluid and the channel walls, leading to more effective heat convection. As a result, the thermal resistance of the microchannel heat sink decreases. The trend in the graph suggests that thermal resistance decreases with increasing Reynolds number, primarily due to the increased velocity of the fluid, leading to an improvement in the convective heat transfer coefficient. Among the different configurations, MC-Mixed exhibits the lowest thermal resistance and, thus, the highest Nu due to its increased surface area for heat dissipation. In contrast, MC-SC has the highest thermal resistance due to its low Nusselt number and less wall surface area for heat dissipation, resulting in a lower heat transfer rate.
Figure 13. Thermal resistance versus Re.
Download figure:
Standard image High-resolution image3.5. Comprehensive performance
Although the pin-fins improve the thermal performance of MCHS, they also decrease hydraulic performance, owing to an increase in the pressure drop within the channel. To assess the overall performance enhancement of MCHS, the thermal enhancement factor is computed by comparing the Nusselt number improvement resulting from the pin-fins with the increase in pressure drop. The addition of pin fins increases the surface area available for heat transfer. It thus enhances the convective heat transfer coefficient, which increases the thermal enhancement factor. Figure 14 illustrates the variation of the thermal enhancement factor with Re. The thermal enhancement factor increases until Re = 600, indicating that the Nusselt number improvement outweighs the pressure losses. However, after Re = 600, the thermal enhancement factor declines because pressure losses surpass the Nusselt number improvement. Among the different configurations, the Mixed wall configuration has the highest thermal enhancement factor of η = 1.4 at Re = 600, and the MC-SW configuration has the lowest thermal enhancement factor.
Figure 14. Thermal enhancement factor versus Re.
Download figure:
Standard image High-resolution imageThe presence of the pin fins causes the fluid flow in MCHS to encounter greater resistance. As the Reynolds number increases, the frictional losses and pressure drop also increase. The MCHS with pin-fins has a greater surface area-to-volume ratio than the MCHS without pins, which leads to faster heat transfer rates. Hence, a higher surface area to volume ratio and greater resistance to fluid flow results in higher pressure losses, which in turn cause a more significant generation of entropy. Figure 15 illustrates the correlation between the Reynolds number and the entropy generated due to pressure drop within MCHS. Pressure losses inside MCHS lead to entropy generation [34]. Smooth channels have the lowest pressure drops, resulting in lower entropy generation. On the other hand, the presence of pin fins leads to more irreversibilities and greater pressure drop, resulting in higher entropy generation. MC-MIXED exhibits the highest entropy generation among the different configurations due to the maximum number of pins. At the same time, MC-SW shows the lowest entropy generation among the pin-fin configurations.
Figure 15. Entropy generation due to pressure drop (W/K) versus Re.
Download figure:
Standard image High-resolution imageEntropy generation due to temperature occurs due to irreversibilities in the heat transfer process, resulting in the dissipation of energy in the form of heat and an increase in the overall entropy of the system. Figure 16 illustrates the correlation between the Reynolds number and the entropy generation resulting from heat transfer. As the Reynolds number increases, the entropy generation due to heat transfer decreases. MC-MIXED exhibits the lowest entropy generation among the pin-fin configurations, while MC-SC has the highest entropy generation due to heat transfer among all pin-fin configurations.
Figure 16. Entropy generation due to temperature versus Re.
Download figure:
Standard image High-resolution imageFigure 17 shows that the total entropy generation decreases with an increase in the Reynolds number. The entropy generation due to temperature has a more significant impact on the overall entropy generation than the entropy generation due to pressure drop. MC-SC exhibits the highest rate of entropy formation and the slowest rate of heat transmission. Among the pin fin configurations, MC-SW displays the highest rate of entropy formation. In contrast, the MIXED configuration has the lowest entropy generation due to the highest heat transfer rate in MC-MIXED.
Figure 17. Entropy generation (W/K) versus Re.
Download figure:
Standard image High-resolution imageFigure 18 displays the relationship between the Reynolds Number and the augmentation entropy generation number (Ns
) for various pin-fins configurations. When is less than 1, the pin-fin configuration performs better regarding heat transfer [34]. Thus, different pin fin configurations should have
less than one to achieve better cooling performance than a smooth channel, which has a
value of 1. The addition of pin-fins in MCHS reduces the total entropy augmentation number by reducing the contribution of entropy generation caused by fluid friction. The entropy augmentation number decreases due to enhanced mixing, increased heat transmission, and less friction from pin fins. With an increase in Reynolds number, almost all pin fin configurations show a declining trend, reaching their maximum at Re = 200 for side wall pin fin configuration. After Re = 600, the
increases due to the rise in pressure drop. Among different configurations, the MC-Mixed pin fins arrangement exhibits the lowest
and gives the best performance in terms of heat transfer, while MC-BW has the lowest increase in
with Re.
Figure 18. Augmentation entropy generation number(Ns) versus Re.
Download figure:
Standard image High-resolution imageFigure 19 compares the Reynolds number and transport efficiency, indicating that the transport efficiency increases with an increase in the Reynolds number. Among the different configurations, the MC-MIXED configuration exhibits the maximum transport efficiency due to the least thermal resistance, resulting in higher heat transfer than MC-SC, which has the lowest transport efficiency.
Figure 19. Transport efficiency (%) versus Re.
Download figure:
Standard image High-resolution image3.6. Response surface methodology (RSM)
The RSM is a collection of statistical methods for creating experimental models with responses and independent variables. These techniques aim to evaluate and enhance a response that depends on several independent variables. In each experiment, the independent variables are changed to determine the reason for these variations in the results. Using this method, the most important variable may be found, and its interactions with other process factors on the examined responses are also conceivable. Before being broadened to mimic numerical trials, Box and Draper's RSM [37] was initially developed to simulate experimental reactions. The second-order polynomial has been demonstrated to be the best equation for fitting the studied data. Using this technique, experimental models are fitted.
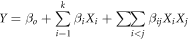

Where the polynomial coefficients are
and
The inputs of the model are given the values.
and
The number of model inputs and the examined response are indicated, respectively, by the letters k and
[36]
3.7. Optimization of base wall pins using RSM
With the addition of the pin-fins, thermal performance increases; to find the optimized values of transverse pitch (), longitudinal pitch (
), and the diameter of the pin (
) for base wall pins, RSM is used. The range of three independent variables and the goal of optimization are shown in table 5. To find optimal geometry, RSM optimization is used at Re 300, which resulted in the geometry of transverse pitch (
) = 2.50 mm, longitudinal pitch
= 0.25 mm, and the diameter of the pin (
) = 0.045 mm, with optimal values of Nusselt number and pressure drop with the desirability of 85.1%, as shown in table 6. To analyze the performance of the pin fin design, the Design Expert provided twenty comparisons using six center points. Table 7 demonstrates the Nusselt number and pressure drop values, calculated using numerical calculations and RSM model predictions. It is observed that the values predicted by the RSM models and numerical calculations are approximately identical, indicating that the values predicted by the RSM model are correct. The most significant Nusselt number is at row 1 (
= 1.00 mm,
= 0.15 mm, and
= 0.045 mm) while the most significant pressure drop is at the 4th row (
= 2.50 mm,
= 0.25 mm, and
= 0.045 mm), as shown in table 8. In the first row, the number of pins is more due to a low transverse pitch, which improves the convection heat transfer coefficient by providing a greater exposed area resulting in a higher Nu. In contrast, in the 4th row, the transverse pitch is large; hence, the pins are less, resulting in a lower pressure drop. To examine the optimal performance of a microchannel, we must find a geometry that gives the optimum values of Nusselt number (Nu) and pressure drop (ΔP).
Table 5. Range of independent variables.
Name | Goal | Lower limit | Upper limit |
---|---|---|---|
A-![]() | Is in range | 1 | 2.5 |
B- ![]() | Is in range | 0.15 | 0.25 |
C: ![]() | Is in range | 0.035 | 0.045 |
Nu | Maximize | 7.45037 | 10.4 |
P drop | Minimize | 1495.75 | 3489.74 |
Table 6. Solutions.
S.no |
![]() |
![]() |
![]() | Nu | P drop | Desirability |
---|---|---|---|---|---|---|
1 | 2.50 | 0.25 | 0.045 | 9.77974 | 1661.36 | 0.851(Selected) |
2 | 2.49 | 0.25 | 0.045 | 9.77623 | 1662.19 | 0.850 |
3 | 2.50 | 0.25 | 0.045 | 9.76345 | 1659.68 | 0.848 |
4 | 2.46 | 0.25 | 0.045 | 9.75823 | 1667.08 | 0.846 |
5 | 2.50 | 0.25 | 0.045 | 9.75839 | 1669.57 | 0.845 |
6 | 2.50 | 0.25 | 0.045 | 9.73851 | 1657.11 | 0.844 |
7 | 2.45 | 0.25 | 0.045 | 9.7501 | 1669.62 | 0.844 |
8 | 2.50 | 0.25 | 0.045 | 9.74004 | 1676.54 | 0.840 |
9 | 2.50 | 0.25 | 0.045 | 9.69844 | 1652.97 | 0.838 |
10 | 2.50 | 0.25 | 0.044 | 9.66369 | 1649.36 | 0.832 |
Table 7. RSM values for P AND Nu.
Run | Nu | P | ||
---|---|---|---|---|
Numerical | RSM | Numerical | RSM | |
1 | 10.4 | 10.1661675 | 3489.77 | 3504.178797 |
2 | 7.55124 | 8.620763 | 1956.607 | 1955.420606 |
3 | 9.983579 | 10.4276525 | 2956.066 | 2947.094297 |
4 | 10.2156 | 9.780175 | 1647.628 | 1661.063685 |
5 | 8.756802 | 9.221341 | 2318.546 | 2310.612653 |
6 | 8.305202 | 8.33658 | 1748.514 | 1732.804536 |
7 | 7.45037 | 7.925262 | 1838.255 | 1803.981811 |
8 | 8.831956 | 8.9517625 | 2946.808 | 2911.31391 |
9 | 7.692492 | 7.51184 | 1495.739 | 1530.028322 |
10 | 9.293111 | 8.921052 | 2210.841 | 2219.121662 |
11 | 9.293111 | 8.921052 | 2210.841 | 2219.121662 |
12 | 8.502079 | 9.3071255 | 2373.153 | 2412.433457 |
13 | 9.293111 | 8.921052 | 2210.841 | 2219.121662 |
14 | 8.283417 | 8.5349785 | 2068.023 | 2019.622867 |
15 | 9.293111 | 8.921052 | 2210.841 | 2219.121662 |
16 | 9.6617 | 9.38028 | 3093.918 | 3066.462636 |
17 | 9.293111 | 8.921052 | 2210.841 | 2219.121662 |
18 | 9.293111 | 8.921052 | 2210.841 | 2219.121662 |
19 | 10.4 | 10.1661675 | 1965.309 | 1932.217572 |
20 | 7.55124 | 8.620763 | 3489.77 | 3504.178797 |
Table 8. Set of runs given by RSM for MC-BW.
Run | Variables | ||
---|---|---|---|
![]() |
![]() |
![]() | |
1 | 1.00 | 0.15 | 0.045 |
2 | 1.66 | 0.25 | 0.040 |
3 | 1.00 | 0.15 | 0.035 |
4 | 2.50 | 0.25 | 0.045 |
5 | 1.66 | 0.15 | 0.040 |
6 | 2.50 | 0.20 | 0.040 |
7&8 | 1.66 | 0.25 | 0.035 |
9 | 1.00 | 0.25 | 0.045 |
10 | 2.50 | 0.15 | 0.035 |
11 | 1.66 | 0.20 | 0.040 |
12 | 1.66 | 0.20 | 0.040 |
13 | 1.66 | 0.20 | 0.045 |
14 | 1.66 | 0.20 | 0.040 |
15 | 1.66 | 0.20 | 0.035 |
16 | 1.66 | 0.20 | 0.040 |
17 | 1.00 | 0.20 | 0.040 |
18 | 1.66 | 0.20 | 0.040 |
19 | 1.66 | 0.20 | 0.040 |
20 | 2.50 | 0.15 | 0.045 |
3.8. Analysis of variance (ANOVA)
The ANOVA provides the relationship between the independent variables and responses under study. It also determines the significance of variables among each other. Additionally, it is used to find the reliability and relevance of the regression models. Tables 9 and 10 give Nusselt number and Pressure drop results generated using ANOVA. The 'F-value' shows the significance of the model's terms, and the 'P-value' is the probability of the F-test. The lower the P-value, the more effective the term. According to the ANOVA results, the term is statistically significant when the P-value is less than 0.05. In the case of MC-BW, Nusselt number (Nu), are independent variables that could significantly affect the value of Nusselt number due to 'P-value' less than 0.05. The 'P-value' for Pressure drop (ΔP) is less than 0.05, indicating that A (Px), B (Py), C (Dpin), AB, AC, BC, A2, and B2 can all have a significant impact on the ΔP. Within the case of MC-Mixed, for Nusselt number (Nu),
and AB have a 'P-value' less than 0.05 suggesting that these independent variables in the model could significantly influence Nu. Furthermore, the 'P-value' becoming less than 0.05 for Pressure drop (P),
have a more significant effect on the dependent variable. Table 11 provides the four functions to determine Nusselt number (Nu) and pressure drop (ΔP) data accuracy. The coefficient of determination (
) describes how well the regression model fits the derived data. The R-squared and adjusted R-squared values indicate a better fit for the model. The coefficient of determination of 71.34% and 99.72% is acquired for Nusselt number (Nu) and pressure drop (ΔP), respectively. This means 71.34% of Nu and 99.72% of the ΔP data fit the regression model.
Table 9. ANOVA'S results for Nu for MC-BW.
Sources | Sum of squares | DF | Mean square | F Value | P-Value |
---|---|---|---|---|---|
Model | 9.98 | 6 | 1.66 | 5.39 | 0.0054(significant) |
A(![]() | 2.05 | 1 | 2.05 | 6.65 | 0.0229 |
B (![]() | 0.42 | 1 | 0.42 | 1.37 | 0.2632 |
C(![]() | 1.55 | 1 | 1.55 | 5.02 | 0.0432 |
AB | 2.80 | 1 | 2.80 | 9.09 | 0.0099 |
AC | 0.32 | 1 | 0.32 | 1.03 | 0.3289 |
BC | 0.69 | 1 | 0.69 | 2.22 | 0.1598 |
Residual | 4.01 | 13 | 0.31 | ||
Correlation | 13.99 | 19 |
Table 10. ANOVA'S Data for ∆P for MC-BW.
Source | Sum of squares | DF | Mean Square | F Value | p-value Prob > F |
---|---|---|---|---|---|
Model | 4.838E+006 | 9 | 5.376E+005 | 392.53 | < 0.0001 |
A-![]() | 3.266E+006 | 1 | 3.266E+006 | 2384.54 | < 0.0001 |
B-![]() | 2.385E+005 | 1 | 2.385E+005 | 174.17 | < 0.0001 |
C-![]() | 3.111E+005 | 1 | 3.111E+005 | 227.14 | < 0.0001 |
AB | 34796.89 | 1 | 34796.89 | 25.41 | 0.0005 |
AC | 8066.46 | 1 | 8066.46 | 5.89 | 0.0356 |
BC | 15343.89 | 1 | 15343.89 | 11.20 | 0.0074 |
A^2 | 1.972E+005 | 1 | 1.972E+005 | 144.00 | < 0.0001 |
B^2 | 21519.70 | 1 | 21519.70 | 15.71 | 0.0027 |
C^2 | 27.78 | 1 | 27.78 | 0.020 | 0.8896 |
Residual | 13694.70 | 10 | 1369.47 | ||
Lack of Fit | 13694.70 | 5 | 2738.94 | ||
Pure Error | 0.000 | 5 | 0.0 | ||
Correlation | 4.852E+006 | 19 |
Table 11. The Results of the statistical criteria for assessing the RSM model's accuracy.
Error function | RSM | |
---|---|---|
Pressure drop | Nu | |
R-Squared | 0.9972 | 0.7134 |
Adj R-Squared | 0.9946 | 0.5811 |
Pred R-Squared | 0.9509 | −0.1929 |
Adeq Precision | 75.445 | 8.877 |
3.9. Results of ANOVA
RSM method is used to give the best polynomial equations for Nusselt number (Nu) and pressure drop (ΔP) as








3.10. RSM results
Figure 20 of the RSM perturbation graphs illustrates the effects of independent factors on the responses, including transverse pitch, longitudinal pitch, pin diameter, and side wall pitch. The impact of the variables on the response increases with the slope of the variable. According to the graph, the Nusselt number (Nu) decreases by 10.6% when the transverse pitch is changed from 1 mm to 2.5 mm while keeping
= 0.2 mm and
= 0.04 mm constant, 3.3% when the longitudinal pitch
is changed from 0.15 mm to 0.25 mm by keeping
= 0.04 and
= 1.75 constant, and the Nusselt number (Nu) increases by 8.23% when the
is changed from 0.035 mm to 0.045 mm by keeping the
= 1.75 and
=0.2 constant. In addition, the pressure drop (ΔP) decreases by 44.6% when the transverse pitch
is changed from 1 mm to 2.5 mm by keeping
= 0.2 mm and
= 0.04 mm constant, 20.8% when the longitudinal pitch
is changed from 0.15 mm to 0.25 mm have keeping
= 0.04 and
= 1.75 constant, and increases 35.4% when the
is changed from 0.035 mm to 0.045 mm by keeping the
= 1.75 and
=0.2 constant. According to the above numerical data, the transverse pitch
and
have a more significant impact on the Nusselt number (Nu) and pressure drop (ΔP) than the longitudinal pitch
Figure 20. (A) Perturbation Graph for Nu for MC-BW. (B) Perturbation Graph for P drop for MC-BW.
Download figure:
Standard image High-resolution imageFigure 21 indicates that the transverse pitch is more effective on the Nusselt number (Nu) and pressure drop (ΔP) compared to other factors. This means that it is possible to optimize the Nusselt number (Nu) and pressure drop (ΔP) by changing the value of the transverse pitch
The three-dimensional contours of Nusselt number (Nu) and pressure drop (ΔP), shown in figure 21, demonstrate the two-by-two response surface relationship between all three independent variables while keeping one constant. From figure 20, it is clear that the increase in transverse pitch increases Nu and pressure drop. At the same time, the longitudinal pitch and diameter of the pin have minor effects on Nusselt number (Nu) and pressure drop (ΔP). When the transverse pitch is low, the number of pin-fins will increase, increasing the surface area for convective heat transfer and Nu. Increasing the number of pin-fins also causes flow hindrances and pressure drop. Similarly, an increase in diameter increases Nusselt number (Nu) and pressure drop (ΔP), while an increase in longitudinal pitch decreases the pressure drop. To achieve the optimum pressure drop and Nu, we can use the geometry predicted by RSM, as shown in table 12.
Figure 21. 3D Surfaces Graph of (A)Nu with and
(B) Nu with
and
(C) Nu with
and
(D) P.Drop with
and
(E) P.Drop with
and
(F) P.Drop with
and
.
Download figure:
Standard image High-resolution imageTable 12. Optimized parameters for MC-BW.
![]() |
![]() |
![]() | ∆P | Nu | Desirability |
---|---|---|---|---|---|
2.50 | 0.25 | 0.045 | 1661.36 | 9.77974 | 0.851 |
3.11. Optimization of mixed pins configuration
For the optimization of mixed pins configurations, the range of four independent variables is given in table 13. The RSM optimization gives the geometry (= 1.0 mm,
= 0.150 mm,
= 0.035 mm and
) with optimum values of Nusselt number (Nu) and pressure drop (ΔP) with the desirability of 60.9%, shown in table 14. For the Nusselt number 2FI model is selected, and for the pressure drop linear model is selected by the RSM. For the Nusselt number from table 15 model F-value of 15.10 implies that the model is significant. There is only a 0.01% chance that an F-value of 15.10 could occur due to noise. P-values less than 0.0500 indicate that model terms are significant. A, B, C, D, and AB are significant model terms. Values greater than 0.10 indicate that the model terms are not significant. For the pressure drop from table 16 model F-value of 24.24 implies that the model is significant. This value indicates that there is only a 0.01% chance of noise. A P-value less than 0.05 indicates that model terms are significant. A, C, and D are significant model terms in this case. To examine the optimal performance of a mixed pin-fins configuration to find a geometry that gives the optimum Nusselt number (Nu) and pressure drop (ΔP) at Re 300. RSM provided twenty-seven runs for MC-MIXED, as given in table 17, with one central point used to find the optimized parameters. The predicted values of the Nusselt number and pressure drop by the RSM can be found using these equations.








Table 13. Constraints.
Name | Goal | Lower limit | Upper limit |
---|---|---|---|
A:Px | is in range | 1 | 2.5 |
B:Py | is in range | 0.15 | 0.25 |
C:Dpin | is in range | 0.035 | 0.045 |
D:P(side wall) | is in range | 0.5 | 1.25 |
Nu | maximize | 11.056 | 15.5986 |
P drop | minimize | 3073.68 | 7936.09 |
Table 14. Optimized geometry of mixed pins by RSM.
No | Px | Py | Dpin | P(side wall) | Nu | P drop | Desirability |
---|---|---|---|---|---|---|---|
1 | 1.0 | 0.1 | 0.03 | 1.25 | 13.9 | 4877.1 | 0.609 (Selected) |
2 | 1.0 | 0.1 | 0.03 | 1.24 | 13.9 | 4880.6 | 0.609 |
3 | 1.000 | 0.150 | 0.035 | 1.240 | 13.906 | 4889.872 | 0.608 |
Table 15. ANOVA results for Nu.
Source | Sum of squares | df | Mean square | F-value | p-value |
---|---|---|---|---|---|
Block | 0.0267 | 1 | 0.0267 | ||
Model | 0.5624 | 10 | 0.0562 | 15.10 | <0.0001(Significant) |
A-Px | 0.2147 | 1 | 0.2147 | 57.63 | < 0.0001 |
B-Py | 0.0828 | 1 | 0.0828 | 22.22 | 0.0003 |
C-Dpin | 0.1145 | 1 | 0.1145 | 30.73 | < 0.0001 |
D-P(side wall) | 0.0833 | 1 | 0.0833 | 22.35 | 0.0003 |
AB | 0.0461 | 1 | 0.0461 | 12.38 | 0.0031 |
AC | 0.0020 | 1 | 0.0020 | 0.5291 | 0.4782 |
AD | 0.0030 | 1 | 0.0030 | 0.7963 | 0.3863 |
BC | 0.0058 | 1 | 0.0058 | 1.56 | 0.2310 |
BD | 0.0051 | 1 | 0.0051 | 1.38 | 0.2583 |
CD | 0.0051 | 1 | 0.0051 | 1.36 | 0.2614 |
Residual | 0.0559 | 15 | 0.0037 | ||
Lack of Fit | 0.0558 | 14 | 0.0040 | 49.02 | 0.1115 |
Pure Error | 0.0001 | 1 | 0.0001 | ||
Correlation | 0.6449 | 26 |
Table 16. ANOVA results for P.drop.
Source | Sum of squares | df | Mean square | F-value | p-value |
---|---|---|---|---|---|
Block | 121.99 | 1 | 121.99 | ||
Model | 1450.71 | 4 | 362.68 | 24.24 | <0.0001(Significant) |
A-Px | 922.22 | 1 | 922.22 | 61.64 | < 0.0001 |
B-Py | 50.13 | 1 | 50.13 | 3.35 | 0.0814 |
C-Dpin | 250.26 | 1 | 250.26 | 16.73 | 0.0005 |
D-P(side wall) | 229.29 | 1 | 229.29 | 15.33 | 0.0008 |
Residual | 314.17 | 21 | 14.96 | ||
Lack of Fit | 314.16 | 20 | 15.71 | 46229.55 | 0.0037(Significant) |
Pure Error | 0.0003 | 1 | 0.0003 | ||
Correlation | 1886.87 | 26 |
Table 17. Set of runs given by RSM.
Factor 1 | Factor 2 | Factor 3 | Factor 4 | Response 1 | Response 2 | |
---|---|---|---|---|---|---|
Run | A: Px | B: Py | C: Dpin | D:P(side wall) | Nu | P drop |
mm | mm | mm | mm | Pa | ||
1 | 2.5 | 0.25 | 0.045 | 0.5 | 14.5113 | 6351.02 |
2 | 1 | 0.15 | 0.035 | 0.5 | 14.501 | 6346.62 |
3 | 1.66 | 0.2 | 0.04 | 0.875 | 12.48 | 4407.33 |
4 | 1 | 0.25 | 0.045 | 1.25 | 12.8564 | 5581.59 |
5 | 1 | 0.25 | 0.035 | 1.25 | 11.96 | 4556.17 |
6 | 2.5 | 0.25 | 0.035 | 0.5 | 11.431 | 3382.59 |
7 | 2.5 | 0.15 | 0.035 | 1.25 | 11.431 | 3382.59 |
8 | 1 | 0.25 | 0.035 | 0.5 | 12.8162 | 5400.24 |
9 | 1 | 0.15 | 0.035 | 1.25 | 13.7567 | 5456.64 |
10 | 1 | 0.15 | 0.045 | 1.25 | 14.6945 | 6469.71 |
11 | 2.5 | 0.15 | 0.045 | 0.5 | 12.8487 | 4496.78 |
12 | 1 | 0.25 | 0.045 | 0.5 | 13.7929 | 6840.92 |
13 | 2.5 | 0.15 | 0.035 | 0.5 | 12.48 | 4186.34 |
14 | 2.5 | 0.25 | 0.045 | 1.25 | 11.567 | 3630.16 |
15 | 2.5 | 0.25 | 0.035 | 1.25 | 11.056 | 3073.68 |
16 | 2.5 | 0.15 | 0.045 | 1.25 | 12.4191 | 4147.15 |
17 | 1 | 0.15 | 0.045 | 0.5 | 15.5986 | 7936.09 |
18 | 1.66 | 0.2 | 0.04 | 0.875 | 12.4828 | 4407.33 |
19 | 2.5 | 0.2 | 0.04 | 0.875 | 11.7844 | 3707.03 |
20 | 1.66 | 0.2 | 0.03 | 0.875 | 11.5272 | 3769.47 |
21 | 1.66 | 0.2 | 0.04 | 0.875 | 12.3929 | 4403.87 |
22 | 1 | 0.2 | 0.04 | 0.875 | 13.826 | 5994.5 |
23 | 1.66 | 0.2 | 0.04 | 1.25 | 12.316 | 4213.23 |
24 | 1.66 | 0.15 | 0.04 | 0.875 | 12.924 | 4596.02 |
25 | 1.66 | 0.25 | 0.04 | 0.875 | 11.76 | 3991.3 |
26 | 1.66 | 0.2 | 0.04 | 0.5 | 12.88 | 4810.3 |
27 | 1.66 | 0.2 | 0.045 | 0.875 | 12.7654 | 3800.87 |
4. Limitations
In this study, our main focus was to propose a novel modification for MCHS that enhances the thermal performance with reduced pressure drop. Response surface methodology is used to optimize the design parameters of MCHS. For RSM results a case study at Reynolds number 300 is used, and it is established that RSM gives accurate results compared to laminar flow simulations, providing a foundation for researchers to apply this RSM method to obtain optimized geometrical variables at different Reynolds numbers. While this study focuses on thermal performance analysis of MCHSs with pin fin configurations, there is still a potential to expand geometric optimization across the entire Reynolds number range from 100–1000. We have not explored all Reynold numbers as it will increase the length of the paper. The decision to focus on Reynolds numbers within the 100–1000 Reynold number range is also due to the undesirability of turbulent flow beyond 1000 Reynolds numbers as we have assumed laminar flow in this study.
5. Conclusion
This study evaluates thermal enhancement in MCHS with novel pin-fins configurations using three-dimensional steady laminar flow numerical simulations. The impact of pin-fins on the thermal enhancement in MCHS, as well as pressure drop, is investigated. Various configurations are compared, and an optimized pin-fin configuration is found using RSM. The significant conclusions regarding the effectiveness of pin-fins for enhancing the thermal performance of MCHS are:
- Pin-fins reduce the thermal resistance of MCHS by increasing the surface area available for heat transfer. The MC-Mixed configuration exhibits the lowest thermal resistance and the Nusselt number of 24.84, 2.3 times greater than a smooth channel at Re = 1000.
- The thermal enhancement factor for all pin-fins configurations is greater than one for the Re range of 100–1000 due to the larger surface area-to-volume ratio and high convective heat transfer coefficient, which increases heat transfer efficiency. The MC-Mixed configuration exhibits the highest thermal enhancement factor (η = 1.4) at Re 600, which is 40% higher than the smooth channel.
- MC-MIXED has the highest transport efficiency because of increased exposed surface area for convective heat transfer, resulting in more effective heat dissipation. In contrast, MC-SW has the lowest transport efficiency among the investigated pin fin configurations.
- Pin fins increase the microchannel heat sink thermal performance at the expense of a rise in pressure drop. MC-MIXED has the highest pressure drop, and MC-SW has the lowest pressure drop at Re= 1000 among the studied pin fin configurations.
- Pin fins reduce the entropy generation rate associated with MCHS. Among all configurations, MCHS with MC-MIXED exhibits the lowest augmentation entropy generation value of 0.47. This indicates that it has the lowest entropy generation rate, 53% less than the smooth channel.
- MC-Mixed is the best pin-fin configuration based on the augmentation entropy generation number and thermal enhancement factor. However, the thermal enhancement factor decreases after Re = 600 due to a higher pressure drop than the Nusselt number improvement.
- For the mixed wall configuration, the optimized geometry using RSM is
= 1.0 mm,
= 0.150 mm,
= 0.035 mm, and
= 1.250 mm with a desirability of 60.9%. For the base wall configuration, the optimized geometrical parameters are
= 2.50 mm,
= 0.25 mm, and
= 0.045 mm, with a desirability of 85.1%
Acknowledgments
The authors acknowledge the support of UET Peshawar for providing the computational facilities to conduct this research.
Data availability statement
All data that support the findings of this study are included within the article (and any supplementary files).
Author contributions
KA, HA, and MAW contributed to the conception of the study. UG organized the database. KA, MAW, MW, and SS performed the numerical analysis. MAW and UG wrote the first draft of the manuscript. All authors contributed to the manuscript revision and reading and approved the submitted version.
Competing interests
The authors declare no conflict of interest.