Abstract
The effect of plasma-processed air (PPA) treatment with different conditions (time, power and flow rate) on the inactivation of Escherichia coli (E. coli) in button mushroom was evaluated. Response surface methodology (RSM) was applied to optimize PPA treatments on the E. coli of button mushrooms. According to the response surface analysis, the optimal treatment parameters were a treatment time of 12 min, treatment power of 90 W and flow rate of 1.2 l min−1. As with verifying tests from the optimization exercise, the number of E. coli reduced by 5.27 log CFU/g at the determined optimum conditions. The scanning electronic microscopy (SEM) micrography showed that the surface of the E. coli was significantly changed under the optimized PPA treatment. Quality parameters of button mushrooms treated at the determined optimum conditions were compared with untreated samples during the storage for 12 d at 4 °C ± 1 °C. The PPA treatment was found to be effective in inhibiting microbes and preserving postharvest quality in button mushrooms, and these results suggested PPA treatment may provide an alternative for the sterilization of foodborne and maintaining postharvest of fruits and vegetables.
Export citation and abstract BibTeX RIS
1. Introduction
Fresh fruits and vegetables are an essential part of the healthy diet, which we are recommended to consume at least five daily. The demand of fruits and vegetables has increased rapidly due to the beneficial health effects [1]. However, foodborne illnesses associated with fruits and vegetables have been widely reported in the last few years [2, 3]. Foodborne outbreaks were not only harmful for the health of consumers but also resulted in significant economic losses for both farmers and distributors [4]. Moreover, an outbreak of foodborne diseases caused a huge potential threat to the globalization of fruits and vegetables supplies [5]. Among all kinds of foodborne breaks, E. coli could cause serious foodborne diseases, which is one of the most common biological contaminants in fruits and vegetables [6, 7]. E. coli infections for fruits and vegetables could be contaminated while growing, harvesting, storage or distribution, which have been found quite normal in fresh fruits and vegetables. Therefore, the sterilization of foodborne bacterial strains has been one of the major challenges in the preservation of fruits and vegetables.
In recent years, a number of disinfection methods for fresh fruits and vegetables have been studied extensively by many researchers [4, 8–11]. All these methods have been proposed for the inhibiting of foodborne pathogens but also have their own drawbacks. For example, organic acids, such as citric acids, lactic, tartaric and acetic, have been applied for the inhibition of the growth of microbes like E. coli and Listeria monocytogenes on vegetables. These acids are generally harmless to the human body and easy to use. However, these acids have negative impacts on the sensory properties of vegetables after application [12, 13]. Some chemical agents, such as chlorine solutions, could leave some residues on the surface of fruits and vegetables and had the limitation of the sterilization of all of the microbial loads [14]. Consequently, alternative methods for the decontamination of fresh produces with microbials are needed to be exploited.
Currently, a number of nonthermal processing technologies for fruits and vegetables have the potential to address the demands of consumers. These methods included pulsed electric, ultrasound, pulsed light, irradiation, high-pressure processing, ozone and hydrostatic pressures [15, 16]. Non-thermal plasma or cold plasma, as a relatively novel technology, has been applied in agricultural production that aimed to reduce the amount of microorganism effectively meanwhile maintaining the postharvest quality for fresh produces. Cold plasma is produced by the excitation of gas in a strong electric field at ambient temperature. Cold plasma involved neutral ionized gases such as electrons, photons, positive and negative ions, atoms, on-excited molecules and free radicals. Cold plasma has the potential to be applied in sterilization for many different types of foodborne pathogens on fruit and vegetables. Antimicrobial effects on the surface of different fruits and vegetables have already been conducted [17–24]. Cold plasma exhibited high potential on fresh produce, however, it is still a very early phase of research and development. Baier et al [25] pointed out the small size of available plasma sources was a major problem, which is limited to distract from realistic appropriateness for scale-up. To meet this essential requirement, the indirect method of plasma-processed air (PPA) has great potential to be applied to disinfect larger workpieces. Furthermore, PPA treatment is also characterized by a very efficient antimicrobial action in fresh fruit and vegetables. Bußler et al [26] reported PPA treatment could reduce the numbers of E. coli on apples, cucumbers, carrots and tomatoes by 3.4, 1.2, 5.2 and 3.3 log cycles, respectively. Thus, PPA treatment showed a high potential on applications for fruits and vegetables.
Nonetheless, the sterilization efficiency strongly depends on the treatment parameters. The diversity of treatment parameters results in large variation in the sterilization efficiency of the respective plasma. To our knowledge, little research has focused on thesterilization efficiency of PPA treatment under different treatment conditions. Additionally, further investigation of the effect of PPA treatment on fresh produce quality should also be taken into account because cold plasma treatment under improper treatment conditions may cause notable damage in food surface [18]. Therefore, the aims of this study were to (1) investigate the effects of PPA treatment on the inactivation of E. coli in button mushrooms by including treatment time, treatment power and flow rate and (2) optimize the sterilization treatment levels and (3) assess the effect of PPA treatment on the post-harvest quality of button mushrooms during the storage period under optimized parameters.
2. Materials and methods
2.1. Button mushroom preparation
Button mushrooms were freshly harvested from Zibo City in Shandong province, China, and then stored at 4 °C for up to 24 h until use. The button mushrooms were selected for uniformity in shape, size, color and no physical injury. Prior to infection, the button mushrooms were immersed in a 300 mg l−1 sodium hypochlorite solution for 2 min in order to allow for decontamination. Thereafter, the decontaminated mushrooms were rinsed in sterilized deionized water and dried in the hood for 30 min at 20 °C ± 1 °C. For post-harvest quality analysis, the mushrooms were evaluated to determine the effects of PPA treatment on weight loss, firmness, cell membrane relative leakage rate, whiteness index and physical surface appearance.
2.2. Button mushroom inoculation
The button mushrooms were artificially inoculated with E. coli to obtain an initial microbial load of approximately 107 CFU/g. For this purpose, each mushroom was inoculated on the surface of the cap by a pure culture suspension of E. coli. [27]. The inoculated mushrooms with the caps facing up were air-dried in the hood for 1 h before PPA treatment.
2.3. Experimental set-up
Figure 1 showed the schematics of the plasma source for PPA treatment and experimental setup. The atmospheric-pressure dielectric barrier discharge (DBD) plasma source consisted of a ground electrode (copper; length: 30 mm) wrapping around the quartz tube. The concentric threaded stainless-steel rod acts as an inner electrode (diameter: 2.5 mm, length: 80 mm) and a quartz tube served as a dielectric material (diameter: 20 mm, length: 200 mm). The high voltage power supply (Beijing Institute of Technology, China) connected to the copper electrode. The winding of plasma source received input at 230 V, 50 Hz and delivered a high voltage output in the range of 0–15 kV. The fed gas was dried air atmospheric and the air flow rate was controlled by an air pump (Shenzhen Aim Technology CO., Ltd, China) at the range of 0–3 l min−1. The process chamber (30 cm (width) × 35 cm (height) × 40 cm (length)) is made of polypropylene and connected to the temperature and humidity controller. The controller consisted of a temperature and humidity sensor, cooling fan, heating apparatus and humidifier. The temperature and humidity sensor can detect the changes of temperature and humidity and adjust the temperature and humidity according to the cooling fan, heating apparatus and humidifier, which could simulate the real environment of the button mushroom. The air pump, DBD plasma source and process chamber were connected by a silicone tube (diameter: 10 mm), and the distance between DBD plasma and process chamber was 50 mm, which aimed at avoiding electrical and thermal damage by plasma treatment.
Figure 1. Schematic diagram of experimental setup for the plasma-processed air system.
Download figure:
Standard image High-resolution image2.4. Plasma-processed air treatment
Inoculated button mushrooms were placed in the process chamber treated with PPA under different treatment conditions and collected in sterile boxes for further analyses. The treatment conditions included treatment times of 10, 12 and 14 min, flow rates of 0.9, 1.2 and 1.5 l min−1 and treatment power of 60, 80 and 100 W, respectively. Three replications at the center point was applied. Single-factor experiments were performed by a fixing flow rate of 1.2 l min−1 and changing treatment power of 60 W, 80 W and 100 W, each treatment time for 10 min up to 14 min. To evaluate the impact of flow rate on sterilization efficiency, the treatment power of 80 W was fixed, and different flow rates of 0.9, 1.2, 1.5 l min−1 treated for 10 min to 14 min were conducted. Each treatment was repeated three times.
2.5. Microbiological analysis
Determination of E. coli population was carried out as described by Guan et al and Xu et al [28, 29]. Four button mushrooms were blended with 0.08 l 1 g l−1 serially peptone water (PW) in stomacher bags and massaged at the inoculated cap surface by hand for 1 min. Treated samples were serially diluted in 9 ml 1 g l−1 PW and 0.1 ml of each dilution was placed on a count agar. These Petri dishes were incubated at 37 °C for 36 h and the colonies of E. coli counted for 0–300. The results reported as log CFU/g. Inactivation of E. coli attributable to each treatment was expressed as the following equation.

where N represents post-treatment cell cultivability and N0 was the initial inoculated mushrooms counted in the same way.
2.6. Experimental design
The different conditions (treatment power, treatment time, flow rate) for sterilization were optimized by response surface methodology (RSM) using Design-Expert software (Version 8.0.6.1 Stat-Ease Inc., Minneapolis, MN, USA). Box–Behnken experimental design with three variables, i.e. treatment time (X1), treatment power (X2) and flow rate (X3), each at three levels (10, 12, 14 min, 60, 80, 100 W, 0.9, 1.2, 1.5 l min−1, respectively) and three replications at the center point were applied. With as the response value, the levels and codes for the experiment were shown in table 1.
Table 1. Box–Behnken experimental design and results.
Variable codes | ||||
---|---|---|---|---|
Run number | Treatment time (min) X1 | Treatment power (W) X2 | Flow rate (l min−1) X3 | Reduction Loga (CFU/g) Y |
1 | 14 | 100 | 1.2 | 4.56 |
2 | 10 | 100 | 1.2 | 3.90 |
3 | 12 | 80 | 1.2 | 4.77 |
4 | 14 | 60 | 1.2 | 3.48 |
5 | 14 | 80 | 1.5 | 4.26 |
6 | 12 | 80 | 1.2 | 5.04 |
7 | 12 | 100 | 1.5 | 4.35 |
8 | 12 | 60 | 1.5 | 3.44 |
9 | 14 | 80 | 1.5 | 2.83 |
10 | 10 | 80 | 0.9 | 1.92 |
11 | 10 | 60 | 1.2 | 1.09 |
12 | 14 | 80 | 0.9 | 3.80 |
13 | 12 | 100 | 0.9 | 4.14 |
14 | 12 | 80 | 1.2 | 4.58 |
15 | 12 | 60 | 0.9 | 1.98 |
16 | 12 | 80 | 1.2 | 4.71 |
17 | 12 | 80 | 1.2 | 5.18 |
2.7. Scanning electron microscopy (SEM)
The effect of PPA treatment by different treatment time on E. coli 48 h bacterial biofilms formed on the cap of mushrooms was observed using SEM (FEI Sirion 200, FEI, USA). The method of SEM for E. coli on the surface of the button mushroom was adopted as described in [30].
2.8. PPA treatment of the button mushrooms (Agaricus bisporus)
The treatment condition was used based on the results of RSREG. The button mushrooms treated with PPA for 12 min acted as PAA-12. The button mushroom without any treatment represented the CK. The mushrooms were placed in the processed chamber under the optimized treatment conditions. After treatment, the button mushrooms were stored in the processed chamber at 4 °C ± 1 °C and 90% relative humidity (RH) for 12 d. The 30 mushrooms were randomly selected and analyzed for post-harvest quality parameters every 3 d. Weight loss was determined by weighing the button mushrooms before and after the storage period. Firmness was measured with a GY-1 penetrometer (Mudanjiang Machinery Research Institute, China). The cell membrane permeability was expressed by tissue electrolyte leakage. Electrolyte leakage was measured following a procedure from [31]. The surface color of the mushroom caps was determined according to the method in [32].
2.9. Statistical analysis
Statistical data analysis of three independent replicates was performed and the data is expressed as the mean ± standard deviation values. The results were calculated and graphs were obtained using Origin software (version 8.5), followed by one-way analysis of variance (ANOVA) at a significance level of 0.05.
3. Results and discussion
3.1. Impact of treatment time and treatment power of PPA on E. coli inactivation
The reductions of population of E. coli on button mushrooms after PPA treatment at different power for treatment times up to 14 min with a flow rate of 1.2 l min−1 are shown in figure 2. Obviously, the population of E. coli decreased by increasing plasma exposure time. Compared to the untreated samples, the reduction of 4.11, 4.95 and 5.12 log CFU/g at treatment power of 60 W, 80 W and 100 W, respectively. The lowest inactivation of E. coli was obtained at the lowest treatment power (40 W), whereas there were no significant differences between 80 W and 100 W treatment samples. The reason could be that the reactive components increased with treatment time increasing, those reactive components were used to inactivate E. coli on the surfaces of button mushrooms, however, the content of air in the quartz tube was constant and enough reactive components could be generated for reaction with microorganisms at treatment power of 80 W.
Figure 2. Numbers of log reduction of E. coli under different power at a flow rate of 1.2 l min−1.
Download figure:
Standard image High-resolution image3.2. Impact of flow rate of PPA on E. coli inactivation
Air flow rate was also one of crucial process parameters affecting the reduction of E. coli in button mushrooms by PPA treatment. As shown in figure 3, after 14 min treatment, reductions of 4.52, 4.97 and 4.43 log CFU/g were observed at flow rates of 0.9 l min−1, 1.2 l min−1, and 1.5 l min−1, respectively. The strongest inactivation of E. coli was achieved at the flow rate of 1.2 l min−1. It indicated that the react time between plasma-processed air and the button mushroom surface was very short and it may influence the efficiency of inactivation of E. coli. Xu et al [29] found the similar phenomenon by using pulse light (PL) sterilization for E. coli in red grape juice at different liquid flow rates. They found that a high level of flow rate could shorten the contact time between PL and juice.
Figure 3. Numbers of log reduction of E. coli under different flow rates at treatment power of 80 W.
Download figure:
Standard image High-resolution image3.3. Model fitting and response surface analyzing
The Box–Behnken experimental design was carried out by Design-Expert 8.0.6 software and the response surface optimization test was carried out by taking the treatment time, treatment power and flow air as factors and the reduction of E. coli as response value (Y). Test results of 17 groups were obtained as shown in table 1. The second-order polynomial regression equation for the regression model was obtained as follows:
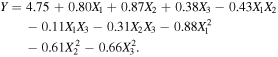
The calculated determination coefficient (R2) was 0.9220. It indicated that the model was effective under the range of the treatment parameters. Table 2 showed the experimental data analyzed by ANOVA. The model F-value of the quadratic regression was 22.01 and P-value (0.0002) < 0.01, which indicates that the model fit was significant. The lack of fit was 0.10, P = 0.88. It indicated that the loss-of-profiling was not significant. To sum up, the second-order polynomial regression equation for the regression model was adapted to the analysis of this response trait. The values of X1, X2, X3, X1X2, X1X3, X2X3,
and
showed that these independent variables were all significant and the influence factors' ranking from large to small is treatment time (X1) > treatment power (X2) > flow rate (X3).
Table 2. Analysis variance (ANOVA) for the fitted quadratic polynomial model for optimization of log reduction.
Variance | Sum of | Degree of | Mean | P-value | |
---|---|---|---|---|---|
sourcea | squares | freedom | square | F value | Prob > F |
Model | 20.90 | 9 | 2.32 | 22.01 | 0.0002 (significant) |
X1 | 5.06 | 1 | 5.06 | 47.93 | 0.0002 |
X2 | 6.06 | 1 | 6.06 | 57.40 | 0.0001 |
X3 | 1.16 | 1 | 1.16 | 10.95 | 0.0130 |
X1X2 | 0.75 | 1 | 0.75 | 7.09 | 0.0323 |
X1X3 | 0.051 | 1 | 0.051 | 0.48 | 0.5108 |
X2X3 | 0.39 | 1 | 0.39 | 3.70 | 0.0957 |
![]() |
3.28 | 1 | 3.28 | 31.10 | 0.0008 |
![]() |
1.56 | 1 | 1.56 | 14.74 | 0.0064 |
![]() |
1.85 | 1 | 1.85 | 17.53 | 0.0041 |
Residual | 0.74 | 7 | 0.11 | ||
Lack of fit | 0.10 | 3 | 0.035 | 0.22 | 0.8794 (not significant) |
Pure error | 0.63 | 4 | 0.16 | ||
Corr. total | 21.64 | 16 | |||
R2 | 0.8774 | ||||
R2Adj | 0.9220 |
The response 3D plots and contour maps (figure 4) showed the mutual effects of two factors. The response surface analysis results for reduction of the E. coli counts showed that the optimal condition was treatment time of 12.60 min, treatment power of 91.53 W and flow rate of 1.24 l min−1. The value of log reduction under this condition was 5.14 logCFU/g. Considering practical operations the optimal parameters were modified to treatment time of 12 min, power 90 W and flow rate of 1.2 l min−1. The value of log reduction with three times experiment was 5.27 logCFU/g. Therefore, the log reduction under optimized condition was closed to the predicted one.
Figure 4. Double-factors mutual effect on the log reduction. (a) Treatment power (X2) and treatment time (X1), (b) flow rate (X3) and treatment time (X1) and (c) flow rate (X3) and treatment power (X2).
Download figure:
Standard image High-resolution image3.4. SEM
To further our understanding of the inactivation mechanism, especially the effect of treatment time on ultrastructural changes of cell morphology, the antimicrobial activities of E. coli were evaluated by SEM in the present study. SEM analysis was obtained on E. coli cells, including PPA treatment for 0, 4, 8 and 12 min, respectively on button mushrooms (treatment power: 90 W, flow rate: 1.2 l min−1) and immediately stored for 24 h prior to SEM preparations. The observation of SEM images in figure 5(a) showed that E. coli in the control sample were a healthy rod shape. The wall of E. coli was continuous and there were no cracks, pores, crevice, or interruption on the structures. The PPA-treated E. coli for 4 min in figure 5(b) indicated that the surface of the cell was distinctly wrinkled and, after 8 min of PPA treatment (figure 5(c)), part of bacterial cells were visibly disintegrated. Figure 5(d) showed the significant changes in cell morphology compared with the other samples. Most bacterial cells were completely disintegrated. As we mentioned above, under the optimal condition (treatment time: 12 min, treatment power: 90 W, flow rate: 1.2 l min−1), the reduction of E. coli on the button mushroom was 5.27 CFU/g. Figure 5 showed the process of the cell morphology changes of E. coli under PPA treatment, it should be pointed out that the treatment time is proportional to the extent of the disruptive membrane structure. Similarly, van Bokhorst–van De Veen et al [33] reported that cold plasma treatment induced morphological changes and SEM images showed that the surface of the spore was significantly changed by cold plasma treatment. In addition, severe physical damage, such as irregular surfaces and etching on the spores, were also observed. Huang et al [34] used the atmospheric plasma to treat E. coli. The cell morphology was significantly changed at 75 W after 2 min. Their results clearly revealed that the E. coli cell had severe cytoplasmic deformations and leakage of the bacterial chromosome. Additionally, the loss of viability of bacterial cells was also observed after cold plasma treatment. In this study, the reactive species in plasma-processed air, such as O2, O3, and NO2, were potentially effectively sterilizing agents, which have been widely associated with the oxidative effects on the surface of microbial cells. The E. coli cells on the surface of the button mushroom were exposed to an intense bombardment by reactive species and radicals, which can lead to E. coli cell wall ruptured. The structural bonds like C–O, C–N bonds in cell wall component peptidoglycans may be destructed by reactive species such as O2, O3, and NO2. The main effect of the PPA treatment is the action of ROS and RNS on the microbial cells, mainly ozone [35]. Moreover, gram-negative cells such as E. coli have outer membranes composed of lipopolysaccharides and a very thin layer of peptidoglycans (only 2 nm), which was easily disrupted [36]. Feng et al pointed out charge accumulation on the outer surface of the membranes was the main reason of the inactivation of gram-positive bacteria [37]. This may partially explain the reduction of E. coli and SEM images under PPA treatment.
Figure 5. SEM images of control and treated E. coli after PPA treatment (treatment time: 0, 4, 8, 12 min, treatment power: 90 W and flow rate: 1.2 l min−1) at 50 KVRMS for 1 min following 24 h of post-treatment storage. (a) Untreated E. coli, (b) treated E. coli for 4 min, (c) treated E. coli for 8 min, and (d) treated E. coli for 12 min.
Download figure:
Standard image High-resolution image3.5. PPA treatment of the button mushrooms
Figure 6(a) showed the weight loss of the button mushroom during 12 d of storage period. Untreated button mushrooms showed a weight loss of 3.21% at the end of 12 d of storage period. It is suggested that dehydration is one of important processes during post-harvest storag,e which could cause quality loss with button mushrooms. Because the epidermal structure of the button mushroom was very thin, it could not prevent a quick superficial water loss. Compared with control samples, PPA-treated mushrooms for 12 min showed a weight loss of 1.92% at the end of the 12 d of storage. Substance consumption and water transpiration by respiration were major reasons for the weight loss of the button mushroom. Xu et al [38] reported cold plasma treatment (plasma active water) could reduce the weight loss for button mushrooms and they found that it is consistent with strong suppression of respiration rate. In this study, PPA treatment recorded the lower weight loss during the entire storage period, which suggested that PPA treatment could also inhibit the respiration of button mushrooms.
Figure 6. (a) Weight loss, (b) firmness, (c) membrane permeability, (d) whiteness index of the surface of CK and PPA-12 (treatment time: 12 min, treatment power: 90 W and flow rate: 1.2 l min−1) treated button mushroom during 12 d storage. Each data point is mean ± standard deviation. Vertical bars represent the standard deviation of means of three replicates.
Download figure:
Standard image High-resolution imageThe aging of button mushrooms lead to a soft and spongy texture characterized by the softening of the button mushroom. Generally, firmness tended to decrease during the whole storage time (figure 6(b)). Protein and polysaccharide degradation, central vacuole disruption and hyphae shrinkage were main reasons for the softening of the button mushroom. It was reported that the bacterial populations play a crucial role in the post-harvest quality of mushrooms. Therefore, it was extremely important to suppress microbial growth for delaying the softening of button mushrooms. Figure 6(b) showed PPA-12 samples were significantly firmer than control samples and the firmness was 5.29 N and 7.37 N in control and PPA-12 groups, respectively at 12 d of the storage time. Similar results were obtained by Tappi et al [39] who reported that the maximum firmness of mushrooms was observed by gas plasma treatment for fresh-cut apples. Generally, in this study, plasma-processed air treatment seemed to delay the softening of button mushrooms by inactivating microbes.
The cell membrane relative leakage rate of plant tissues is an index that was connected to tissues and membrane integrity. It was also reported that cell membrane relative leakage rate indicated the aging degree of the plant. The changes in cell membrane relative leakage rate from button mushrooms were presented in figure 6(c). Generally, the relative leakage rate was enhanced as the storage time increased. Similarly, Jing et al [40] pointed out that the membrane system of button mushrooms was more vulnerable to leakage. Particularly, the button mushroom in control groups showed the higher level of the cell membrane relative leakage rate during the whole storage time. After 12 d of storage, the relative leakage of the untreated group was 55.59%, while only 51.19% of that was observed in the PPA-12 treated button mushrooms. From the results, we found that PPA treatment may lengthen the aging of the button mushroom.
Button mushrooms have very limited shelf life. The browning of the surface color on button mushrooms has been a major post-harvest problem that affects the consumer's acceptance as an obvious indicator. As shown in figure 6(d), the whiteness index for cap button mushrooms decreased rapidly as storage time increases. It is suggested that mushrooms turn brown easily and lose their quality just in a few days after harvest. Compared with control samples, the mushrooms in the PPA-12 group had a higher whiteness index. At the 12 d of storage, the WI value of control and PPA-12 were 75.92 and 78.47. The results showed that PPA treatment could effectively inhibit the browning of mushrooms (p < 0.05). The physical appearance of button mushrooms was observed in figure 7. The button mushrooms experienced a change from white to brown. During the 12 d of storage, PPA-12 treatment showed the better sensory quality.
Figure 7. The physical appearance of button mushrooms of CK and PPA-12 (treatment time: 12 min, treatment power: 90 W and flow rate: 1.2 l min−1) treated button mushrooms during 12 d storage time.
Download figure:
Standard image High-resolution imagePPA treatment effectively delayed the browning in the surface cap of the button mushrooms. The main reason for this might be that PPA treatment for 12 min could generate a number of reactive oxygen species (ROS) and reactive nitrogen species (RNS), which may suppress the enzymes associated with browning. Moreover, PPA treatment has the effective ability for the inhibition of microbial growth, which also resulted in a better quality of button mushroom.
4. Conclusions
PPA treatment was an effective alternative for inactivation and the quality of the preservation of fruits and vegetables. In this study, the optimal parameters of PPA treatment for microbial inactivation of E. coli in button mushrooms were the treatment time of 12 min, treatment power of 90 W and flow rate of 1.2 l min−1. The results demonstrated that PPA treatment under optimized conditions could significantly improve the inactivation, the log reduction improved by at least 5 logs compared with un-treatment samples. The SEM images showed the morphologies of E. coli were strongly dependent on the condition of treatment time. For application to the button mushroom, PPA treatment exhibited the lower weight loss, membrane permeability and the higher firmness and whiteness index (WI) compared with the CK group. This study showed the application of PPA, as an indirect treatment, may offer an alternative technology for the preservation of fruits and vegetables.
Acknowledgments
This work was supported by National Natural Science Foundation of China (No. 31972144). This work was also supported by Beijing University of Agriculture (BAU).