Abstract
In this work, we report a flexible nanofibrous cellulose nanocomposite with great potential for lithium-ion battery (LiB) polymer electrolyte. Flexible and fibrous material is synthesized using a simple and easy technique by synergistically combining carboxymethyl cellulose (CMC) and glycerol (Gly). Flexible porous cellulose forms a three-dimensional network for the mobility of Li ions in the polymer electrolyte of LIB systems. We investigated the effect ionic liquid of flexible fibrous cellulose (BC-CMC-Gly) on the electrochemical properties. The surface interaction between Li ions and the porous network is a key parameter demonstrated by the Li-ion emission line at 610.37 nm using laser inductance breakdown spectroscopy (LiBS). The ionic conductivity of BC-CMC-Gly characterized by EIS measurement is about 1.1 × 10−3 S cm−1. According to linear sweep voltammetry (LSV), BC-CMC-Gly, with a potential window of 4.3 V, shows a more expansive window voltage than pure BC (2.75 V) and BC-CMC (3.3 V). This indicates that the electrochemical stability is good, as wide as the range of voltages that the electrode reactions define. The specific capacity of BC-CMC-Gly containing IL is very high, about 27.6 mAh g−1 compared to BC (7.4 mAh g−1) and BC-CMC (11,5 mAh g−1). All these findings clearly show that forming plasticized structures synergistically with CMC trapped in the BC structure results in the largest Li-ion adsorption capacity and electrochemical performance improvement. Thermal stability up to 200 °C and electrolyte uptake of approx. 189% are the beneficial properties of BC-CMC-Gly fibrous cellulose for LiB electrolyte polymer.
Export citation and abstract BibTeX RIS

Original content from this work may be used under the terms of the Creative Commons Attribution 4.0 licence. Any further distribution of this work must maintain attribution to the author(s) and the title of the work, journal citation and DOI.
1. Introduction
According to Li-ion battery research, polymer electrolyte materials are developing quite rapidly. Electrolyte polymers are advantageous because the polymer matrix replaces the liquid solvent, eliminating the need for a separator, reducing the electrode volume expansion, and improving safety, corrosion resistance, and flexibility [1]. Electrolyte polymer batteries are very popular, but their conductivity is still low (<10−3 S cm−1). At the same time, the li-ion battery must have a conductance of ions in the range of 10−3 –10−2 S cm−1 at room temperature. A large amount of Li+ transference, a broad electrochemical stability range, mechanical stability, and exceptional thermal stability are prerequisites to guarantee safe battery operation under electrical short circuits, overcharging or thermal abuse [1, 2]. Many investigations have been conducted to produce effective polymer electrolytes, and it is necessary to experiment with various host polymers, metal salts, liquid solvents, and inorganic fillers. Polymer hosts must have mostly polar groups for binding metal salts and chemical, thermal and mechanical stability [1]. Today, several energy storage applications report the excellent performance potential of cellulose because the polar hydroxyl functional groups on the cellulose skeleton accelerate the dissociation of salts, increasing the ionic conductivity [3, 4]. Its hydrophilicity, unique network structure and high mechanical strength make cellulose an ideal natural material for polymer electrolytes [5].
The macromolecular structure of bacterial cellulose comprises b—>1,4-D-glucopyranose units coupled by intermolecular hydrogen bonds [6, 7]. Strains of the bacterium Acetobacter typically manufacture bacterial cellulose [8]. The three-dimensional structure of cellulose-containing novel porous BC sponges could easily be controlled through drying techniques, such as the hot drying technique. Bacterial cellulose would have a rigid structure under the hot, dry technique and mechanical properties with less porous but cavities and cracks configurations [9, 10]. The primary goal of incorporating BC into a biopolymer electrolyte system was to increase the electrolyte's resistance to temperature changes [11]. Due to its favorable mechanical qualities and compatibility with Li salts, bacterial cellulose (BC), an environmentally benign filler, can create a high-performance solid polymer electrolyte [12]. However, BC as a polymer matrix retaining more liquid components can improve the polymer electrolyte conduct ions (10−4 S cm−1 at the standard temperature) [4].
In the previous research, we investigated the effect of dry technique treatments, such as oven and freeze drying, to allow cross-linking reactions [13]. Freeze drying is a suitable treatment to generate a porous and soft structure. However, the molecular chain rigidity of bacterial cellulose for electrolyte polymers is still an obstacle to its mechanical stability [13]. Flexible molecular chains with more polar groups provided excellent thermal properties and a high rate of lithium-ion transfer [14].
Therefore, in this current study, we further modified the porous structure of cellulose to be a more flexible nanofibrous cellulose nanocomposite by incorporating carboxymethyl cellulose (CMC) and glycerol for LiB polymer electrolytes (PE). Flexible and nanofibrous cellulose networks are promising to promote non-leaking, nonflammable characteristics and Li-ion mobility of polymer electrolytes (PE). This paper reports that cellulose nanocomposite significantly reduced structural rigidity and improved electrochemical performance. CMC is a highly promising alternative polymer that might minimize the aggregation of microfibrillar/fiber bundles of BC, and glycerol is selected as a plasticizer containing hydroxyl groups to dissolve and separate excess salt [15–17].
2. Experimental procedures
2.1. Materials
Bacterial cellulose (BC) gel was commercially purchased from the local market in Tangsel, Indonesia. Carboxymethyl cellulose (CMC) and glycerol were purchased from Merck. Sigma-Aldrich was the supplier for the ionic liquids lithium hexafluorophosphate (LiPF6), ethylene carbonate/diethyl carbonate (EC/DEC), and 1-hexyl-1 methylimidazolium iodide (HMII).
2.2. Fabrication of BC composite-based PE
The pH of the BC gel was adjusted to a neutral state by rinsing it with deionized water after it had been filtered using 1% NaOH at room temperature for an hour. BC suspension was prepared by crushing with an electric crusher for 10 min The sample is denoted as BC. CMC was dissolved in distilled water and glycerol in liquid form in nanocomposite preparation. To 200 g of suspension BC, we added 30% w/w CMC and 30% v/v glycerol relative to the dry mass of suspended BC and then heated at 60 °C while stirring for 3 h at 300 rpm. The BC composites were degassed in an ultrasonic bath to remove bubbles, poured onto glass plates using a solution casting technique, freeze-dried, and then stored in a desiccator with a moisture content below 2%. The BC composites were labeled BC-CMC-Gly. Fabricated in a glovebox with argon and a humidity level of 0.1 ppm, the PE had a membrane thickness of 100 mm, a diameter of 19 mm and was made from BC and BC composite materials. After that, the samples were submerged in a lithium hexafluorophosphate (LiFP6) solution in EC/DEC with a 1:1 molar ratio and ionic liquid at room temperature. The solution had a volume of 0.5 ml. Last but not least, the BC and PE-based BC composites were allowed to dry at ambient temperature. We have six samples (BC, BC CMC, BC CMC Glycerol, BC IL, BC CMC IL, BC CMC Glycerol IL) for each test condition.
2.3. Characterization method of BC Composite-based PE
Utilizing a Thermo Fisher Scientific Nicolet iS-10 from Japan, we carried out a Fourier transform analysis to investigate the material's structural components. During the spectrum collection process, the spectra were taken at room temperature utilizing surrounding air as a background spanning the wavelength range of 4000 to 700 cm-1. Differential scanning calorimetry (DSC) (EXSTAR 6000 DSC6220, SEIKO, Tokyo, Japan) was used to investigate crystallization temperature (Tc) and melting temperature (Tm) regions, and a D2 Phase BRUKER x-ray powder diffractometer with Cu Kα1 (1.5406 Å) radiation was used to assess crystallinity. In order to characterize the morphology of the BC composite, field emission scanning electron microscopes of the type JEOL JIB 4160F were used. The BC composites' thermal decomposition was studied using a thermogravimetry analysis (TGA) machine (EXSTAR 6000 TG/DTA7200 standard type, SEIKO, Tokyo, Japan) at a rate of 10 °C min−1 from 30 °C to 450 °C. Before undergoing thermal examination, all of the films were freeze-dried. Lithium detection materials from BC composite membranes were analyzed using laser-induced breakdown spectroscopy (LIBS) techniques. The Nd:YAG laser (1064 nm wavelength, 30 mJ laser energy) used in this experiment was run in Q-switched. In order to determine the amount of electrolyte that the dried BC absorbed, it was placed inside an argon-filled glove box for one hour. The weight of BC both before (W0) and after (Wt) absorption was measured, which allowed for the determination of the liquid electrolyte uptake(η). This was done by using the following formula:
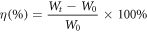
After sandwiching the BC composite membrane between two stainless steel (SS) disks, the ionic conductivity of PE was measured using an AC impedance spectroscopy equipment (Metrohm AutoLab) over a frequency range of 0.1 and 106 Hz. The measurement was conducted in a controlled chamber for temperature adjustment between 25 and 80 °C for the electrochemical impedance spectroscopy (EIS) tests. Direct current (DC) polarization measurements (TNMs) were taken by following the polarization current. An asymmetric cell was used to check the electrolyte membrane's ion transference number. The (SS/PE/SS) symmetric cell battery was used to measure the lithium-ion transference number Of the polymer electrolyte. The Wonatech Battery Cycler System maintained a cell's consistent 10 mV polarization voltage. Sandwich configurations of stainless steel (SS), polymer electrolyte (PE), and lithium metal (Li metal) have been used for Linear Sweep Voltammetry (LSV) measurements with a scan rate of 5 mV s−1. The working electrode is stainless steel, while the counter and reference electrodes are Li metal. In order to carry out cyclic voltammetry and charge-discharge experiments, CR2032 coin cells were built with Li metal serving as the reference and counter electrode; and LiMnPO4 serving as the positive electrode. We repeated at least twice the measurements for each variation to determine the pattern.
3. Results and discussion
3.1. Characterizations of structure and morphology
FTIR analysis was conducted to investigate the intermolecular complexation and interactions between the BC, CMC, and glycerol based on molecular vibrations of a particular functional group. Figure 1(a) depicts FT-IR spectra of the samples containing liquid electrolyte (LiPF6) (black line) and containing LiPF6 and ionic liquid (red line). FTIR region of 4000–700 cm−1 indicates the functional group of cellulose and its nanocomposite with CMC and CMC-glycerol. Peaks of 3440 cm−1, 2927 cm−1, 1650 cm−1, and 1440 cm−1 in FTIR spectra (figure 1) of BC represent O-H stretching, C-H stretching of alkane, and asymmetric CH2 stretching, O-H deformation, and CH2 deformation, respectively. Cellulose contains hydroxyl groups, the sites of numerous types of hydrogen bonding inside and between molecules. Hydrogen bonding, both within and between cellulose molecules, contributes significantly to cellulose's material characteristics. BC-CMC (figure 1(b)) did not show a significant change in the absorption of the BC functional group, while BC-CMC -Gly (figure 1(c)) shows a broader OH stretching absorption (3440 cm−1) caused by the glycerol hydroxyl group and sharper C-H stretching (2927 cm−1). Glycerol acts as a plasticizer by changing cellulose's intermolecular and intramolecular hydrogen bonds, while CMC acts as a crosslinker of intermolecular chains of cellulose. Carboxymethyl substitution result in higher ionic conductivity; it may act as additional attachment sites for mobile ion transport because it has enough electron-donor capability to establish coordination bonds with the cations and compensate for the salt's lattice energy. The simultaneous interaction of cellulose with both changes the physical properties significantly. It is more flexible and can withstand mechanical deformation such as crack propagation. Glycerol rich in hydroxyl groups interacts with the functional group of cellulose and plasticized polymer chains' segmental motion [18]. The broader O-H band is a replacement hydrogen bond between cellulose and cellulose that transitions to cellulose and glycerol [19].
Figure 1. (a) BC FT-IR spectrum, (b) BC- CMC, and (c) BC-CMC-Gly composite.
Download figure:
Standard image High-resolution imageWe analyze the absorption spectrum of the nanocomposite containing LiPF6 and IL. We identified a new infrared absorption peak at 741 cm−1, 1169 cm−1 and 1573 cm−1 corresponding to LiPF6, C-N stretching vibration and the imidazolium framework vibration, respectively. Using ionic liquids (ILs) as electrolyte components (solvent for the lithium salt or electrolyte additive) can improve battery safety [20].
Figure 2 shows the comparison of BC nanocomposite XRD versus bulk XRD. Diffraction peaks of those samples appear dominant at 2θ = 14.5°, 16.5°, and 22.5°, which correspond to (−110), (110) and (200) diffraction planes, respectively. It was reported that cellulose crystals became more plane oriented (110). Crystallinity obtained from XRD data was also calculated according to the Segal method [21]. The estimated crystallinity index of BC, the BC CMC, and the BC CMC Gly composites were approximately 84.95%, 74.85% and 64.5%, respectively. CMC and glycerol decrease the crystallinity index of the nanocomposite. This may be due to the strong molecular interaction between BC with CMC and glycerol, which disrupts inter-intra BC molecular hydrogen bonding. As well known, the high crystallinity of BC hinders the migration of Li+ ions and leads to low ionic conductivity of polymer electrolytes [22]. Polymer electrolyte materials that support good ionic mobility and transfer are required. Studies have shown that high crystallinity can suppress ionic conductivity and lithium-ion migration [13, 16]. Therefore, the amorphous domain of BC-CMC-Gly nanocomposite due to both interaction of CMC and glycerol in BC becomes one of the important characteristics of LIB electrolyte polymer. Flexible chain molecular dynamics in the amorphous domain support Li-ion transfer.
Figure 2. BC composite XRD diagram.
Download figure:
Standard image High-resolution imageFigure 3 presents a surface morphology of freeze-dried BC compared with BC CMC and BC CMC Gly composites. The Nanofibril structure of BC (figures 3(a) and (b)) forms a strong fiber network of macromolecular structure. Compared with nanocomposites, the BC CMC fiber network (figures 3(c) and (d)) is thicker and coarser due to CMC's adsorption on the fiber network's surfaces. In figures 3(e) and (f), glycerol flexes the entire fiber network to become more flexible and smoother, forming a porous structure with homogeneous surface morphology. The fibril network provides a primary route for transferring electrons and lithium ions. Glycerol is a plasticizer that supports the connected nanofibrous network to retain stresses caused by volume changes during long-term cycles and prevent fracture. Mechanically stable electrolyte polymers will provide proper electron and ion pathways.
Figure 3. FESEM morphology showing (a) BC, (b) BC IL, (c) BC CMC, (d) BC CMC IL, (e) BC CMC Glycerol, and (f) BC CMC Glycerol IL.
Download figure:
Standard image High-resolution imageThe heat absorbed by BC and its nanocomposite material is plotted against temperature using differential scanning calorimetry (DSC) in figures 4(a)–(c). In general, the glass transition temperatures (Tg) depend on the interactions between molecules, the molecular weight, the flexibility of the chain, and the number of branches and cross-links [23]. In order to successfully manufacture an electrolyte, it is necessary to consider not only the polymer's glass transition temperature (Tg) but also its crystalline structure and how well it can dissolve ions. Solvation is facilitated by the ability of polymers with several high-polar sites to establish electrostatic connections with cations. Without IL, the engineered bacterial cellulose composite's glass transition temperature (Tg) is significantly greater than that of the BC Composite with IL. The generated bacterial cellulose composite may have a larger crystalline proportion, explaining why its Tg is much greater. From the curve, a lower glass transition temperature is inversely related to ionic conductivity. Therefore it seems sensible that adding CMC and glycerol would have this effect. Increased chain mobility and ionic conductivity coincide with a lower glass transition temperature. The plasticizing effect tends to weaken dipole-to-dipole interactions, which breaks the temporary cross-link bonds between the polymer matrix and backbone and throws off their orderly arrangement. So, it loosened up the polymer lattice and made a very flexible polymer chain. It also improved the segmental quality, which led to higher ionic conductivity [24, 25]. It is possible that the dissolution of the ionic dopant, which generates free Li+ ions that can pass easily through the plasticizer-rich phase, is responsible for the increase in conductivity brought about by the plasticizer [3].
Figure 4. DSC curve of (a) BC, (b) BC CMC, (c) BC CMC glycerol.
Download figure:
Standard image High-resolution imageFigure 5 shows the thermal degradation of BC and its nanocomposites. At first glance, the thermogravimetric BC and BC CMCs had similar profiles, but the weight loss of BC CMC IL nanocomposites was reduced more than that of BC-IL above, most likely due to IL loss. This shows that the absorption of IL by BC CMC is greater than BC 250 °C. While without IL, the temperature degradation curves of BC and BC CMC composites showed a gradual weight loss up to 300 °C and a drastic weight loss above 300 °C. This indicates the thermal properties of cellulose. The decomposition of the CMC backbone's carboxylate group (COO-) has also dramatically reduced weight loss. The adsorbed IL prevents excessive weight loss of the nanocomposite up to 150 °C (figure 5(b)). In figures 5(a)–(c), all samples meet the thermal stability up to 150 °C. Meanwhile, BC CMC gly nanocomposite showed a faster weight loss above 100 °C. The content of glycerol and IL tends to be the cause of the weight loss of nanocomposites [26]. This shows that the adsorption power of BC CMC Gly is large compared to the other two samples. A lessening in the crystallinity of the BC CMC Gly is responsible for the increased high chain flexibility of the polymeric matrix, which, in turn, leads to an increase in the mobility of lithium ions [24]. The higher crystallinity of cellulose caused a rise in the thermal degradation temperature [15]. In this research study, The inclusion of glycerol into BC chain molecular increases the chain flexibility of nanocomposite.
Figure 5. TGA curve of (a) BC, (b) BC CMC, (c) BC CMC glycerol.
Download figure:
Standard image High-resolution imageIn the case of Li, one of the light elements that x-ray diffraction spectroscopy cannot detect, it is extremely challenging to determine the amount of Li present in a material or sample. Neutron scattering spectroscopy has shown to be an effective technique for determining the presence of Li elements in samples so far. This results in a significant financial burden due to the production of neutron sources. Laser Inductance Breakdown Spectroscopy (LIBS) is a novel and alternative technique for detecting Li [27, 28]. The data comes from the average of 10 measurement data. Figure 6 spectra revealed the presence of strong lithium emission lines at 610.37 nm. The occurrence of lithium in the BC composite membrane indicated that the lithium salt doping process was successful through the LiPF6 electrolyte immersion process. The type transitions describing the distribution of lithium electrolytes corresponded to the lithium emission lines [29, 30]. The number of Li elements illustrates the porosity of the sample to which they contribute to the electrolyte salt adsorption process. The porous structure of the BC CMC Gly membrane showed the highest lithium detection among BC and BC CMC.
Figure 6. Laser inductance breakdown spectroscopy for lithium detection.
Download figure:
Standard image High-resolution image3.2. Electrochemical performance characteristics
We investigated the electrochemical performance of the nanocomposites in a temperature-controlled chamber from 25 to 80 °C. Figure 7 depicts a Nyquist plot of the ionic conductivity of BC nanocomposite (σ) at various temperatures to know the role of CMC-glycerol on the electrochemical properties of the battery electrolyte. Due to flexible molecular segments, the ionic conductivity of the nanocomposite improved when the temperature was increased. Figures 7(a) and (d) plot shows that only BCs have a minor incomplete or compressed semicircle curve. The semi-circular arcs appeared at a higher frequency (left towards the origin), whereas an inclined straight line to the semi-circular curve is in the lower-frequency region. Our cellulose-based polymer electrolyte shows parallel bulk resistance and capacitance in the previous results, resulting in a semicircle on the Nyquist plot [5]. However, we currently note that the semicircle was reduced, which could be attributed to the complexation of the altered structural properties of the PE, as proven by the FTIR and XRD analyses. With the addition of CMC and glycerol (figures 7(b), (c), (e), and (f)), the system's ionic mobility and the quantity of ion carriers are both enhanced. The impedance spectrum first emerges in the high-frequency region, and then the low-frequency region follows. Double-layer capacitance near the electrode-electrolyte interface is responsible for developing the impedance spectrum in the low-frequency region, formed by the abundance of ion transfer and arcing at high frequencies, as seen by the bulk characteristic of the impedance diagram.
Figure 7. Nyquist plot of BC nanocomposite at temperature variation: (a) BC, (b) BC CMC, (c) BC CMC Glycerol, (d) BC IL, (e) BC CMC IL and (f) BC CMC Glycerol IL.
Download figure:
Standard image High-resolution imageGenerally, during operation, the battery easily reaches a temperature of over 50 °C. For this instant, BC nanocomposite still has a regular size and does not shrink at high temperatures resulting in a cathode-anode short circuit. Increasing temperatures facilitate translational ion transport because of increased biopolymer chain mobility. Ionic liquids (ILs) are electrolyte additives that can increase the electrolyte conductivity of biopolymers by transporting cations by hopping ions to neighboring vacant sites. In addition, the high thermal stability of ILs and the mechanical and chemical durability of polymer components lead to better ionic conductivity.
According to table 1, the ionic conductivity of a mixture of BC CMC glycerol and ionic liquid (IL) is 1.1 × 10−3 S cm−1, superior to the ionic conductivity of real BC, BC CMC, and BC CMC glycerol. ILs absorbed by porous structures create new ion transport routes, increasing ionic conductivity. Glycerol, which has three hydroxyls (OH), groups, facilitates more salt separation and disruption of hydrogen bonds between cellulose polymer chains. Cation-anion columbic interaction has been reduced by the addition of glycerol. Nevertheless, the inclusion of glycerol is more than 30% resulting in a reduction in conductivity by displacing the cellulose polymer that serves as the host. For this instant, the molecular displacement causes salt crystallization resulting in a decreased conductivity. The highest electrolyte absorption of about 189% is a favorable property of the BC-CMC-glycerol fibrous structure for LiB polymer electrolytes. Swelling properties of all nanocomposites against salt electrolytes are shown in figure 8, BC membrane fortified with cmc glycerol is more flexible than a bc membrane on its own. It can better withstand the mechanical stresses imposed by the battery's charging and discharging cycles without being punctured by lithium dendrites.
Table 1. Ionic conductivity and electrolyte uptake measurement.
PE | Ionic conductivity σ (S cm−1) | Electrolyte uptake (η) | ||||
---|---|---|---|---|---|---|
25 °C | 40 °C | 50 °C | 60 °C | 80 °C | ||
BC LiPF6 | 5.7 × 10−5 | 6.5 × 10−5 | 8.0 × 10−5 | 1.1 × 10−4 | 2.2 × 10−4 | 170% |
BC LiPF6 IL | 1.1 × 10−4 | 1.2 × 10−4 | 1.4 × 10−4 | 1.6 × 10−4 | 2.7 × 10−4 | 172% |
BC CMC LiPF6 | 5.6 × 10−4 | 6.1 × 10−4 | 6.6 × 10−4 | 6.6 × 10−4 | 6.9 × 10−4 | 178% |
BC CMC LiPF6 IL | 7.0 × 10−4 | 7.3 × 10−4 | 7.6 × 10−4 | 7.9 × 10−4 | 9.4 × 10−4 | 183% |
BC CMC Gly LiPF6 | 7.2 × 10−4 | 8.3 × 10−4 | 8.7 × 10−4 | 9.1 × 10−4 | 9.5 × 10−4 | 184% |
BC CMC Gly LiPF6 IL | 1.1 × 10−3 | 2.9 × 10−3 | 3.9 × 10−3 | 5.3 × 10−3 | 8.4 × 10−3 | 189% |
Figure 8. BC blend's physical look after immersion.
Download figure:
Standard image High-resolution imageThe ion conductivity of a polymer electrolyte is determined by its lithium-ion transference number () [31]. Transference number (TNM) measurements were carried out to determine the mechanism of the conductor species of the PE system. The equation can be used to calculate the ion transference number (
).

Where is the initial current and
Refers to the current measured at the steady state.
A transference number can be known from the ratio of constant current versus the current at the beginning. The current was observed to drop rapidly initially before being saturated to a lower current value. The cell was given a continuous polarized voltage of 10 mV a, and the current was measured as it changed from its starting value to its steady-state value. Polarization current, Versus time, t is plotted in figures 9 (a)–(e). BC CMC Gly IL with optimal ionic conductivity performance, it has found that the battery's total current gradually drops till it has fully discharged. This happens when the temperature changes. When conditions are stable, electron mobility is at its maximum. Since the electrolyte is predominantly ionic, the ionic currents traveling through an ion-blocking electrode may decrease dramatically with time, explaining the phenomenon. The Multi OH moiety structure of sample BC CMC Gly IL is an alternate mechanism for free ions from salt to move in PE. Glycerol's OH groups allow it to dissociate more salt while also scarifying the intra and inter-hydrogen bonding within the polymer matrix, which leads to a rise in the number of amorphous structures required for the ion transport process. High-dielectric-constant glycerol in salt solutions decreases anion-cation repulsion, resulting in more mobile ions being available, especially when the measurement temperature increases.
Figure 9. Lithium transference numbers ()) by symmetric Li/BC CMC Glycerol IL PE/Li cells undergo potentiation polarization at 10 mV with variations in temperature measurement (a) 25 °C, (b) 40 °C, (c) 50 °C, (d) 60 °C and (e) 80 °C.
Download figure:
Standard image High-resolution imageLi-ion batteries operate at <60 °C due to thermal instability [32]. Using ionic liquids (ILs) as solvents of lithium salts increases the operating range for Li-ion batteries up to 80 °C. Figures 9(a)–(e) illustrate the increase in the lithium-ion transference number as the temperature increases. At the temperature of 80 °C (), sample BC CMC glycerol IL demonstrates the highest value of 0.59. This shows that temperature significantly affects the transport characteristics of electrolytes in concentration. The electrolyte's diffusivity and conductivity increase exponentially with temperature. Analysis of ionic and salt-solvent complexes' residence times reveals that temperature has a major impact on the complexes' dynamic behavior. Specifically, the rate at which lithium ions exchange counterions and solvent molecules in their initial coordination shell are accelerated by increased thermal energy related to the electrolyte molecules at high temperatures. As a result, temperature plays a significant influence in determining the behavior of the electrolyte, which might influence the battery's overall performance if different temperature versions are used.
The polymer electrolyte's potential operating range and electrochemical stability are crucial. One of the criteria that needs to be chosen for it to be used in electrochemical devices. The linear sweep voltammetry (LSV) technique looks at PE's electrochemical window. From the plot of current versus voltage (figure 10), the current flow of the BC CMC Glycerol PE- figure 10(c) is practically steady until the voltage being applied is changed to a higher value, at which point it achieves a decomposition voltage for CMC glycerol of 4.0 V. When the electrode's potential exceeds the voltage required for decomposition, the current gradually increases. It has been found that the breakdown voltage of PE with BC, CMC, and plasticized glycerol has a higher breakdown voltage than BC CMC PE (3.05 V) figure 10(a) and BC PE (2.25 V) figure 10(b). Thanks to the incorporation of the IL as a salt solvent for the electrolyte, the potential electrochemical range of the composite polymer electrolyte is greatly increased, BC CMC Gly IL (4.3 V), BC CMC PE (3.3 V), and BC PE (2.75 V). Due to the large electrochemical stability window, IL is a potential compound to increase the operating voltages. The unique ionic structure of ILs also increases the electrochemical decomposition voltage. This finding suggests that a high-voltage Li-ion battery can use the BC CMC Glycerol IL composite electrolyte with the largest electrochemical window. The electrochemical window represents the potential range in which oxidation and reduction reactions can occur. For an electrolyte to be inert to both electrodes, its oxidation potential must be greater than the embedding potential of Li+ in the cathode, and its reduction potential must be lower than that of lithium metal in the anode. Therefore, polymer electrolytes must be suitable for both electrode materials within an electrochemical window of up to 4-5 V versus Li/Li+ [33].
Figure 10. LSV was performed on PEs as the applied voltage was swept from 0 V to 5 V at a constant rate (a) BC composite, (b) BC CMC composite and (c) BC CMC Glycerol composite.
Download figure:
Standard image High-resolution imageIn electrochemical analytical methods, cyclic voltammetry (CV) records the current that occurs in response to a change in voltage, which can be related to the electrode's porosity and the electrolyte concentration used to study the redox electrochemical reactions of active materials [34, 35]. figure 11(a) shows the CV curves of pure BC PE membrane. The reduction peak is around 2,75 V perhaps a phase change occurred during the intercalation process, causing this. Li intercalation voltage is related to electrolyte ion kinetics. The electrolyte carries the Li+ ions and doesn't affect the Li+ ion diffusion process inside the electrode system due to the electrochemical processes of charge transfer in the electrode being much slower than those in the electrolyte [34, 36]. The anodic peaks around 4 V and cathodic peaks around 3 V in figures 11(b)–(c) result from the chemical interaction between lithium metal and lithium manganese dioxide.
Figure 11. Cyclic voltammetry of (a) BC, (b) BC CMC composite and (c) BC CMC Glycerol composite.
Download figure:
Standard image High-resolution imageThe CD was measured to determine the maximal capacity during voltage exposure. Initial voltage profiles exhibit galvanostatic charge/discharge curves between Li metal and LiMnPO4 electrodes with LiPF6 immersion electrolyte in BC composite membrane under charge-discharge current density of 0.2 mA cm−2 and a potential range of 2.5–4.5 V. The specific capacity of the BC, BC CMC, and BC CMC glycerol composite with IL were 7,4 mAh g−1, 11,5 mAh g−1 and 27,6 mAh g−1, respectively. The charge–discharge capacity increases with Ionic liquid (IL), as described in figure 12(a)–(c). IL may be effective reduces electrode polarization and giving high-specific-capacity batteries power [37]. Because of polarization effects from side reactions at the electrolyte-electrode interface, Li+ would be consumed, leading to a permanent loss of capacity throughout a battery cycle [38]. Throughout the cycling procedure, the good columbic efficiency BC-CMC-Gly-IL (71%) was higher than other samples, indicating significant charge-transfer reversibility via the electrolyte/electrode interface (keeps the efficiency stable by reducing the interfacial resistance). The ionic liquid carries charges, and these charges have the potential to form Columbic and ion-dipole interactions with the surface of the matrix. IL has a wide range of electrochemical stability and the best ionic conductivity; both the cation and anion of imidazolium ionic liquid can interact with bc mc glycerol. IL can be made in many ways because there are many ways to combine anions and cations. So, ionic liquid electrolytes are used as conductive fillers to increase the value of ionic conductivity. Compared to pure BC membrane PE, additive CMC and glycerol can increase battery capacity. The improvement was attributed to the functional hydroxyl (–OH) groups of CMC and glycerol, which may trap undesired dissolved Mn+ ions from the LiMnPO4 cathode [39].
Figure 12. Charge discharge performance of PEs (a) BC composite, (b) BC CMC composite and (c) BC CMC Glycerol composite.
Download figure:
Standard image High-resolution imageWe compared our results with other reported results (figure 13). The figures presented some electrolyte materials such as cellulose [Du et al (2019) [40], Liu et al (2016) [41], lignin [Song et al (2017) [42]], cellulose triacetate (Nirmale et al (2017),[43] Kale et al (2021) [44], MC/Allyl-modified cellulose Yu et al (2020) [45]. The higher ionic conductivity (6.34 mS cm−1) is found in the cellulose material. Our previous results [Sabrina et al (2021)] [13] indicate that the cellulose-based electrolyte that was prepared has higher conductivity of about 6 mS cm−1 with a lower 0,2 Li transfer number. In this current research, our BC nanocomposite demonstrates a lower ionic conductivity due to the ionic conductivity formula's dependence on the sample's surface area and thickness and the composite membrane's larger size. Types of polymer composite blends can facilitate the ionization of lithium salts and enhance their diffusion because blending multiple polymers can increase chain flexibility and promote segmental chain motion for ion dissociation. Polar groups of CMC are efficient building blocks for lithium salt decomposition. This study aims to develop host blend polymers with low crystallinity and glass transition temperature. The polymer chains' segmental motion generates free volume and facilitates ion migration, increasing the lithium transfer number. The transfer number Li is the amount of the total electric current made up of the cation produced by the decomposition of lithium salts.
Figure 13. Ionic conductivity and Li transfer number compared with existing studies.
Download figure:
Standard image High-resolution image4. Conclusion
We have successfully developed BC composites with CMC and glycerol as PE lithium-ion batteries made by the solution casting technique. Introducing CMC and glycerol into BC-based PE effectively decreases the crystallinity with high chain flexibility. The simultaneous role of CMC and glycerol in BC-CMC-Gly contributes greatly, resulting in the development of porous networks and malleable structures in three dimensions to facilitate the mobility of Li ions and thermal-stable electrochemical properties. BC-CMC-Glycerol nanocomposite also forms a thermally stable nanocomposite with an increased amount of transferred lithium ions, high ionic conductivity, higher charging voltage and wide voltage window for lithium battery applications.
Acknowledgments
The authors would like to express their gratitude to the Japan Society for the Promotion of Science in the form of the RONPAKU Program, the Japan Society for the Promotion of Science (JSPS) KAKENHI 399 Grants (No. 23H02024), Department of Applied Chemistry, Graduate School of Engineering at Osaka University, and Research Center for Advanced Materials for their various forms of support. National Research and Innovation Agency and Advanced Characterization Laboratories Serpong through E-Layanan Sains, Badan Riset Inovasi Nasional for the facilities, scientific, and technical support.
Data availability statement
All data that support the findings of this study are included within the article (and any supplementary files).