Abstract
Gelatin methacryloyl (GelMA) is a versatile biomaterial that has been shown to possess many advantages such as good biocompatibility, support for cell growth, tunable mechanical properties, photocurable capability, and low material cost. Due to these superior properties, much research has been carried out to develop GelMA as a bioink for bioprinting. However, there are still many challenges, and one major challenge is the control of its rheological properties to yield good printability. Herein, this study presents a strategy to control the rheology of GelMA through partial enzymatic crosslinking. Unlike other enzymatic crosslinking strategies where the rheological properties could not be controlled once reaction takes place, we could, to a large extent, keep the rheological properties stable by introducing a deactivation step after obtaining the optimized rheological properties. Ca2+-independent microbial transglutaminase (MTGase) was introduced to partially catalyze covalent bond formation between chains of GelMA. The enzyme was then deactivated to prevent further uncontrolled crosslinking that would render the hydrogel not printable. After printing, a secondary post-printing crosslinking step (photo crosslinking) was then introduced to ensure long-term stability of the printed structure for subsequent cell studies. Biocompatibility studies carried out using cells encapsulated in the printed structure showed excellent cell viability for at least 7 d. This strategy for better control of rheological properties of GelMA could more significantly enhance the usability of this material as bioink for bioprinting of cell-laden structures for soft tissue engineering.
Export citation and abstract BibTeX RIS

Original content from this work may be used under the terms of the Creative Commons Attribution 3.0 licence. Any further distribution of this work must maintain attribution to the author(s) and the title of the work, journal citation and DOI.
1. Introduction
Gelatin-based hydrogels have received great interest in the field of tissue engineering [1–5]. Gelatin is a product of denatured and partially hydrolysed collagen containing bioactive sequences, such as arginine-glycine-aspartic acid (RGD) for cell attachment, and thus is highly desirable as a biomaterial [6, 7]. The tunability of the rheological properties of gelatin has also rendered it to be popular as a bioink [8]. The commonly used bioink among gelatin hydrogels is gelatin methacryloyl (GelMA) which is obtained by modifying gelatin with methacryloyl groups and thus enables formation of covalently crosslinked hydrogels for shape preservation via UV light curing [9, 10].
GelMA is a highly useful material for 3D bioprinting because of its cell compatibility photocurable properties, and tunable mechanical properties [11–17]. However, GelMA has low viscosity at high temperature, which elicits poor printability for bioprinting [18]. Several strategies have been developed to improve the printability of GelMA. For one example, GelMA has been blended with other materials such as gelatin, alginate, hyaluronic acid to improve the processability of GelMA [19]. Another strategy of enhancing the printability of GelMA was to increase the concentration of GelMA above 15%, because a higher concentration of GelMA has offered better printability at high temperature [18, 20, 21]. However, this strategy can compromise cell viability inside dense hydrogels. A dense hydrogel network is not desired for cell encapsulation where it can limit nutrient and waste transportation and inhibit network remodelling and formation of new extracellular matrix proteins [22, 23]. Another strategy is to utilize temperature-sensitivity of GelMA. GelMA like gelatin undergoes a sol–gel transition from high temperature to low temperature, which can vary according to the degree of methacryloylation. Normally, a higher degree of methacryloylation lowers further a sol–gel transition temperature and renders a GelMA solution more Newtonian fluid at room temperature because the methacryloylation of GelMA can cause GelMA to lose its triple-helix formation in a certain degree [10, 24]. Therefore, the printability of GelMA was successfully enhanced when a printer with a feature of precise control over the temperature of the nozzle and the platform was employed, which requires a costly complex system [20].
Recently, Liu et al reported that GelMA physical gels (GPG) could be used for direct bioprinting of porous and soft constructs at relatively low concentrations (3%–5%) [25]. GPG bioinks were prepared by cooling GelMA solutions to 4 °C for 20 min. Then, GPG bioprinting was conducted at 21 °C (slightly lower than room temperature). Consequently, the encapsulated cells were exposed to two very different temperatures (4 °C and 21 °C) sequentially, which might not be ideal for cell culture and furthermore, this requires a printing machine equipped with a temperature-controlled nozzle and platform. Prepolymerizing GelMA is another strategy for direct printing of GelMA. Bertassoni et al have directly printed GelMA with concentrations ranging from 7% to 15% by prepolymerizing GelMA through photo crosslinking inside a glass capillary [26]. The limitation associated with this method is that dispensing of continuous fibres is not possible. In this method, GelMA is aspirated by a piston into a glass capillary and then UV cured in the capillary. The crosslinked GelMA is then removed and assembled ('printed') according to the desired pattern. In addition, it is known that photo crosslinking is a rapid process and therefore to control partial photo crosslinking is a challenging process. Consequently, it is still a significant challenge to design versatile bioink formulations that have the delicate balance between printability and good cell support.
In our work, we attempt to develop a new GelMA bioink with tunable rheological properties by introducing enzymatic crosslinking triggered by microbial transglutaminase (MTGase). As is known, transglutaminases (amine γ-glutaminyl transferase, EC 2.3.2.13) catalyze the formation of covalent bonds between the γ-carbonyl group of a glutamine residue and the ε-amino group of a lysine residue in proteins [27]. The crosslinking reaction can result in a change of the physical and chemical properties of substrates, such as viscosity and thermal stability [28]. In our previous study, we have reported that this enzymatic reaction could influence the rheological properties of GelMA [29]. Nevertheless, the rheology control, printability, and biocompatibility of this developed material for bioprinting have not been demonstrated.
Here, we will employ this strategy to improve the ability of printing 3D cell-laden GelMA constructs. Due to the ability of MTGase to modify rheological properties of GelMA derivatives, our enzyme-induced controllable gelling GelMA system circumvents the need to change the initial GelMA solutions concentration or the need for a more complex machine.
2. Materials and methods
2.1. Synthesis of GelMA
Synthesis of GelMA was conducted under the optimized condition according to the previous work [2]. Briefly, 7.95 g of Na2CO3 and 14.65 g of NaHCO3 were dissolved in 1 l distilled water to produce a 0.25 M carbonate-bicarbonate (CB) buffer solution. Following that, 50 g of Gelatin (Bloom Strength 175, Type A, Sigma-Aldrich) was dissolved into 500 ml of the as-prepared buffer. The pH value of the gelatin solution was gradually adjusted to 9 by adding a 5 N NaOH solution in a dropwise manner. Methacrylic anhydride (MAAnh, Sigma-Aldrich) was added to the solution to achieve the MAAnh:gelatin ratio of 0.05 ml g−1. The reaction proceeded at 50 °C for 3 h. After that, 1 N HCl was added to stop the reaction till the pH value of the solution reached 7.4. The crude product was filtered and dialyzed to remove any unreacted MAAnh and methacrylic acid by-product. Finally, GelMA was lyophilized to obtain a dried product and stored at −20 °C for future use. The yield of GelMA was around 76.0%. As shown in figure S1 is available online at stacks.iop.org/BF/11/025011/mmedia, the degree of substitution of GelMA was 64.8% ± 1.0%, and the amount of methacryloyl groups in GelMA was 0.238 ± 0.004 mmol g−1; thus, the remaining free lysine groups (35.2%) could be utilized for the enzymatic reaction via MTGase.
2.2. Bioink preparation
A GelMA solution was prepared by dissolving the lyophilized GelMA at the concentration of 10% (w/v) in phosphate buffered saline (PBS) solution. Different amounts of MTGase (TG-BW-MH, 100 U g−1, EC 2.3.2.13, Ajinomoto) were separately added to the as-prepared GelMA solutions to prepare GelMA samples with the final concentrations at 1, 3, and 5 U ml−1. The incubation temperature was 37 °C. The incubation durations were 0, 1, 2, 3, and 4 h. MTGase was inactivated by heat treatment (80 °C, 10 min) after the fixed incubation time.
2.3. Rheological properties
The rheological properties of the enzymatically crosslinked samples were tested by a rheometer (MCR 501, Anton Paar Physica, Ostfildern, Germany) with a cone-plate geometry of 25 mm. The time-dependent viscosity during the proceeding enzymatic crosslinking was tested at 37 °C under a shear rate of 100 s−1. To avoid evaporation of water, the solutions were sealed in the tube and incubated at 37 °C and then sequentially loaded onto the rheometer at an interval of 1 h and tested for 1 min. During testing, measurements were collected every second, and the average of 60 data points represented the average modulus of the sample at a different incubation time point.
In addition, rheological properties were also assessed for printability under a printing condition. The solutions were allowed to reach the equilibrium temperature (25 °C) for 2 min prior to performing the experiments. Their viscosity was measured via varying the shear rate from 1000 to 1 s−1 at room temperature (25 °C). Temperature sweep was performed from 37 °C to 5 °C at a ramp rate of 2 °C min−1 with a frequency of 1 Hz and a strain of 3%. To investigate the effect of MTGase treatment on photopolymerization of GelMA, time sweep was performed at a frequency of 1 Hz and a strain of 3% in the rheometer system connected with Omicure s1000 (Excelitas Technologies, Waltham, MA, USA). Samples containing 0.1% w/v Irgacure 2959 (Sigma-Aldrich) were placed between the plates and exposed to UV light (365 nm) at an intensity of about 25 mW cm−2. UV exposure time was 5 min for all samples.
2.4. 3D bioprinting
C2C12 myoblasts were cultured and expanded before bioprinting. The cells were cultured in Dulbecco's Modified Eagle's Medium (DMEM, Hyclone) supplemented with 10% fetal bovine serum (FBS, Hyclone) and 1% antibiotic-antimycotic (ABAM, Life Technologies), in a humidified atmosphere with 5% CO2 at 37 °C. MTGase-GelMA formulations prepared above were bioprinted by using the RegenHU bioprinter (BioFactoryTM). The syringes, nozzles, and pyrex bottles were autoclaved to avoid contamination. MTGase-GelMA solutions containing 0.1% Irgacure 2959 were sterile-filtered through a 0.2 μm syringe filter under sterile conditions. Cell suspension was added to MTGase-GelMA solution, resulting in a final cell concentration of 6 × 105 cells ml−1, which was used for 3D bioprinting of the 3D grid pattern with a dimension of 1.4 cm × 1.4 cm with line spacing of 2 mm. A 27 G straight nozzle (200 μm) was used, and printing pressure was around 1–2 bar. The bioprinted construct was further photo-crosslinked by using the printing system furnished with a UV pen (365 nm, 150 mW, 150 s) at a feed rate 100 mm min−1 to obtain the stabilized structure for further cell study.
The volumetric flow rate of the bioink through a 200 μm diameter needle was approximately 0.463 mm3 s−1. The shear rate exerted on the bioink was calculated according to the equation (1) [30]:
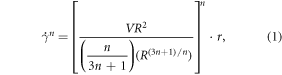
where is shear rate, R is the inner radius of the needle, V is the flow rate of the bioink extruded through the needle, n is the power law index, and r is the radial position (0 < r < R). At the position R (0.1 mm), the shear rate exerted on the bioink was 714.047 s−1. Following that, the shear stress was determined using the power law model (equation (2)):

where τ is shear stress, is shear rate, η is viscosity, K is the consistency index, and n is the power law index. Thus, the shear stress of the bioink experienced under shear rate 714.047 s−1 was 78.317 Pa.
2.5. Cell viability
Cell viability of C2C12 in the cell-laden printed scaffolds was measured using a live/dead assay (Molecular Probes) at day 0, day 3, and day 7. During cell culturing, the cell culture medium was changed every 2 d. Fluorescence microscopy (Zeiss Axio Vert. A1) was utilized to image the live/dead staining of cells. Cell viability was also semi-quantified by counting the live and dead cells in the images. Cell circularity was measured using Image J. The average cell circularity of 30 cells were used.
2.6. Statistic analysis
The experimental results are presented as means ± standard deviations (SDs). The statistical significance of the results was evaluated using Student's t-test for comparing two samples. A p-value < 0.05 was considered statistically significant. The value of n denotes the number of samples or the number of collected data.
3. Results and discussion
In developing the hydrogel bioink, the precursor solutions are aqueous solutions comprising a biocompatible polymer—GelMA, MTGase as a crosslinker, a photo-crosslinking initiator, and, optionally, cells. As illustrated in figure 1, the MTGase-induced controllable GelMA printing process begins with the addition of MTGase into the starting material—GelMA solution. Enzymatic crosslinking will start to take place, and the rheological properties will be monitored. Once the crosslinking has proceeded to a point where the rheological properties of MTGase-GelMA solutions match the required properties for printing, thermal inactivation would be conducted to stop the crosslinking. After optimization, the desired solution is transferred to the printhead and extruded in a series of strands. A secondary crosslinking procedure (photo crosslinking) can be performed post-printing to further stabilize and tailor the mechanical properties of the fabricated 3D multi-layer structures.
Figure 1. Scheme of the MTGase-induced controllable GelMA bioink system. The printing process begins with the addition of MTGase into the GelMA solution. The MTGase-induced crosslinking is stopped by conducting a thermal treatment to inactivate the MTGase after customising the desired rheological properties. Following that, the desired solution is transferred to the printhead and extruded in a series of strands. The secondary crosslinking (light curing) can be performed post-printing to stabilize the fabricated structure.
Download figure:
Standard image High-resolution imageIn our studies, a 10% w/v GelMA solution was used as it has been found that such a low concentration is conducive to cell viability though the printability is significantly compromised [18, 20]. Therefore, MTGase was harnessed to modify the rheological properties without compromising on cell viability. Figure 2 demonstrates the influence of various concentrations (0, 1, 3, and 5 U ml−1) and incubation durations (0, 1, 2, 3, and 4 h) of MTGase on viscosity of GelMA solutions under a shear rate of 100 s−1.
Figure 2. The time-dependent apparent viscosity of 10% w/v GelMA solutions incubated with different concentrations of MTGase at 37 °C under the shear rate of 100 s−1. Arrow-marked points were selected and thermally inactivated for further rheological assessment. Each data point was the average of 60 data points (n = 3).
Download figure:
Standard image High-resolution imageThe GelMA solution alone (that is—before the addition of MTGase) exhibited low viscosities, but upon addition of MTGase and subsequent crosslinking reaction at 37 °C, the viscosity of GelMA increased due to the enzyme-triggered coupling reaction (as shown in figure 2). The viscosity increased at a faster rate when the concentration of MTGase increased. At a MTGase concentration of 1 U ml−1, no distinct viscosity increase was observed over 4 h while there was a large increase in viscosity for solutions containing 3 U ml−1 and 5 U ml−1 MTGase between 3–4 h and 1–2 h, respectively, which could be explained by the formation of a chemical gel.
For bioprinting applications, samples with their viscosities located in the grey shades (as displayed in figure 2) would not be considered for the next experiments, because those irreversibly fixed chemical gels were not suitable for cell encapsulation and printing. The representative arrow-marked points outside the shaded region were selected, and the samples were thermally treated (80 °C, 10 min) to inactivate MTGase for further assessment of their printability. The bioinks obtained from different processing conditions were labelled generically as ATBh, in which A refers to the concentration of MTGase and B refers to the incubation time; as shown in table 1, 0T, 1T4h, 3T3h, and 5T1h are 10% GelMA samples treated with different concentrations of MTGase and different incubation durations. 3T0h, 3T1h, 3T2h, and 3T3h are 10% GelMA samples treated with 3 U ml−1 MTGase over different incubation durations.
Table 1. Labels of MTGase-GelMA.
Group 1 | Group 2 | ||||
---|---|---|---|---|---|
Label | Concentration of MTGase (U ml−1) | Incubation time (h) | Label | Concentration of MTGase (U ml−1) | Incubation time (h) |
0 T | 0 | — | 3T0h | 3 | 0 |
1T4h | 1 | 4 | 3T1h | 3 | 1 |
3T3h | 3 | 3 | 3T2h | 3 | 2 |
5T1h | 5 | 1 | 3T3h | 3 | 3 |
To examine the effect of different shear rates on viscosity of the samples during the printing process, rheological properties of the representative points aforementioned were studied at 25 °C under shear rates ranging from 1000 to 1 s−1. The samples were allowed to reach the equilibrium temperature for 2 min prior to performing the experiments. The resulting rheological data as shown in figure 3, demonstrate that the viscosity decreased with increasing the shear rate, indicating shear thinning behaviour. Using the power law model (equation (2) in section 2.4), the power law index, n and consistency index, K were determined. Three range values can be defined for n: n < 1: shear thinning behaviour; n = 1: Newtonian behaviour; n > 1: shear thickening behaviour.
Figure 3. Flow behaviour of 10% GelMA without/with MTGase treatment at room temperature (25 °C) (a) Group 1; (b) Group 2. Their viscosity was measured via varying the shear rate from 1000 to 1 s−1 at room temperature (25 °C) (n = 3).
Download figure:
Standard image High-resolution imageFrom the data listed in table 2, all samples exhibited shear thinning behaviour (n < 1). The shear thinning behaviour of the materials facilitates the extrusion-based printing process. From Group 1 (table 2), it is obvious that sample 3T3h has the lowest n and the highest K, as compared to 10% w/v GelMA (n = 0.999), indicating that shear thinning is greatly enhanced for 3T3h (n = 0.542). Table 2 (Group 2) shows that as the incubation time lengthens, n is decreased, and K is increased, which is most likely attributable to the increased molecular weight and viscosity of samples when the enzymatic reaction between GelMA and MTGase was allowed to proceed for a longer period of time.
Table 2. Power law index n and consistency index K of MTGase-GelMA.
Group 1 | Group 2 | ||||
---|---|---|---|---|---|
Label | Power law index n | Consistency index K | Label | Power law index n | Consistency index K |
0 T | 0.999 ± 0.016 | 0.007 ± 0.001 | 3T0h | 0.968 ± 0.019 | 0.016 ± 0.030 |
1T4h | 0.894 ± 0.041 | 0.050 ± 0.016 | 3T1h | 0.929 ± 0.017 | 0.243 ± 0.011 |
3T3h | 0.542 ± 0.011 | 2.224 ± 0.530 | 3T2h | 0.646 ± 0.044 | 0.439 ± 0.170 |
5T1h | 0.683 ± 0.025 | 0.386 ± 0.079 | 3T3h | 0.542 ± 0.011 | 2.224 ± 0.530 |
As GelMA is a thermo-sensitive material, it is important to understand the rheological behaviour changes with temperature as well as to investigate the effect of MTGase treatment on these thermal behaviours. To examine the enzymatic influence, temperature sweep was performed by cooling samples from 37 °C to 5 °C at 2 °C min−1. At 37 °C, the materials showed liquid behaviour for G'' (loss modulus) was higher than storage modulus G' (storage modulus). Upon cooling, G' and G'' increased and a crossover occurred (figure 4). The crossover temperature is assigned as the sol–gel transition temperature (gel point), and it suggests the transition from a solution state to a gel state as the temperature is decreased.
Figure 4. Temperature sweep measurements of 10% GelMA with MTGase treatment (a) Group 1; (b) Group 2. Temperature sweep was performed from 37 °C to 5 °C at a ramp rate of 2 °C min−1: G' (solid lines) and G'' (dotted lines) (n = 3).
Download figure:
Standard image High-resolution imageThe sol–gel temperatures of the different conditions are summarized in table 3 below.
Table 3. Sol–gel transition temperature of GelMA with different treatment conditions.
Group 1 | Group 2 | ||
---|---|---|---|
Labels | Sol–gel transition temperature (°C) | Labels | Sol–gel transition temperature (°C) |
0T | 16.4 ± 0.4 | 3T0h | 17.1 ± 0.2 |
1T4h | 20.2 ± 0.4 | 3T1h | 19.2 ± 0.5 |
3T3h | 26.0 ± 0.3 | 3T2h | 21.7 ± 0.5 |
5T1h | 21.9 ± 0.3 | 3T3h | 26.0 ± 0.3 |
In Group 1 (table 3), 3T3h has the highest sol–gel transition temperature at about 26 °C. From data shown in Group 2 (table 3), the sol–gel transition temperature increases from 17.1 °C ± 0.2 °C (3T0h) to 26.0 °C ± 0.3 °C (3T3h) as the incubation time increases. This implies that extending the incubation time could increase the sol–gel transition temperature (table 3), which can be attributed to the more covalent crosslinking action triggered by MTGase, thus leading to a tighter network facilitating chain entanglement. It was reported that when the printing temperature was above gelation temperature, the solution would exhibit liquid behaviour, and the viscosity would be too low for extrusion-based 3D printing studies [31–33]. The samples with a sol–gel transition temperature below room temperature (25 °C) were in a sol state with low viscosity at 25 °C; therefore, lowering the temperature of the printer's platform below the sol–gel temperature may be required to achieve a good shape fidelity. From figures 4, 3T3h shows promising rheological properties for printing at room temperature (25 °C). Interestingly, G' values of GelMA with MTGase treatment tended to level off or slightly increase at high temperature. It is potentially because the elastic portion imparted by enzyme-induced chemical gelation in MTGase-catalyzed GelMA samples could be maintained even at high temperature even though the physical gelation portion could be destroyed at high temperature. This is in contrary to GelMA (0T) sample where it is clearly shown that at higher temperature (above 16 °C) when significant physical crosslinking could not take place, the G' decreased steadily.
While sufficient rheological properties such as shear thinning behaviour are required for printability, further gelation is necessary for handling and maintaining the final shape and thus ensure a prolonged cell culture as well as structural stability at body temperature (37 °C). Thus, photo crosslinking via UV light was conducted to study the effect of MTGase treatment on photopolymerization of GelMA at 37 °C using three different materials: 0T, 3T0h, and 3T3h. Figure 5 depicts the gelation kinetics of 10% GelMA without/with MTGase treatment, showing that a discernible increase can be observed in G', indicating the formation of a robust gel network. At t (time) = 360 s, the storage modulus of 3T3h after photo crosslinking was higher than those of the other two samples, which indicates that MTGase treatment increased the storage modulus of GelMA hydrogels. Besides, from the gelation curve of 3T3h (blue curve), the storage modulus increased tremendously after photo-crosslinking, showing that photo-crosslinking is the dominant mechanism to enhance the mechanical properties of GelMA samples. In addition, figure S2 shows the compressive moduli of MTGase-GelMA samples (9.2 ± 1.1, 11.3 ± 0.4, and 17.9 ± 2.7 kPa for 0 T, 3T0h, and 3T3h, respectively), which was consistent with the rheological results. As shown in figure S3, the swelling ratio of 3T3h was lower than those of other two samples (i.e. 24.7 ± 1.3, 34.1 ± 3.1, and 37.9 ± 2.2 at 24 h for 3T3h, 3T0h, and 0T, respectively), indicating that MTGase treatment increased the crosslinking density.
Figure 5. The gelation kinetics of 10% GelMA without/with MTGase treatment at 37 °C. Samples containing 0.1% w/v Irgacure 2959 were exposed to UV light (365 nm) at an intensity of about 25 mW cm−2. UV exposure time was 5 min for all samples (n = 3).
Download figure:
Standard image High-resolution imageAfter optimizing the materials by screening the concentration and incubation time of MTGase in their rheological properties, their printability was investigated as a proof of concept. The printing was conducted at room temperature (25 °C–26 °C). From the pictures shown in figure 6(a), a fine structure could not be achieved with 0T and 3T0h. With increasing the feed rate, the grid structure of 3T0h gradually became more defined (figure 6(a)) but 3T0h was still not able to support layer-by-layer stacking due to its low viscosity. In contrast, a fine grid structure was achieved using 3T3h bioink as shown in figure 6(b). The strut size decreased with the increased feed rate (525.6 ± 23.1, 220.1 ± 16.1, and 160.3 ± 6.4 μm for 500, 1000, and 2000 mm min−1, respectively). However, the filaments became more discontinuous at the higher feed rates of 1000 and 2000 mm min−1 (figure 6(b)). With a low feed rate (500 mm min−1), 3T3h could form a stacking structure with 5 layers (figure 6(c)). This enhancement could be due to the increase of solution viscosity after MTGase treatment as well as the increased sol–gel transition temperature of 3T3h which is about or above room temperature (25 °C). This in turn enables 3T3h to exhibit gel-like characteristics at the printing temperatures (25 °C–26 °C), thus avoiding the ink from spreading.
Figure 6. 3D printed grid structures at room temperature (25 °C–26 °C). (a) Printed structures with 0T and 3T0h under different feed rates (500, 1000, and 2000 mm min−1) (Scale bar = 0.5 cm); (b) Printed structures with 3T3h under different feed rates (500, 1000, and 2000 mm min−1) (Scale bar = 500 μm); (c) 3T3h printed structures with 1 layer and 5 layers at a feed rate of 500 mm min−1 (Scale bar = 0.5 cm).
Download figure:
Standard image High-resolution imageTo validate the possible use of 3T3h for bioprinting, the printed structure was subsequently photo-crosslinked by using a printer equipped with a UV curing pen (365 nm, 150 mW, 150 s) at a feed rate of 100 mm min−1, and the encapsulated cell viability for (C2C12 cells) was investigated at Day 0, Day 3, and Day 7 (figure 7(a)). Stress associated with the printing process has been cited as a common problem that may impact cell viability [26]. However, in our study, cell viability (98.3% ± 1.3%) at Day 0 that was examined right after printing (figure 7(b)) showed that our printing process was not harmful to cells. During the culture of 7 d, cells inside the enzyme-induced GelMA printed constructs maintained a good cell viability of over 80%. Cell viability at Day 3 and Day 7 was 80.6% ± 1.5% and 89.7% ± 1.6%, respectively. More importantly, as shown in figure 7(c), cells exhibited a more elongated morphology with prolonged cell culture (cell circularity of 0.25 ± 0.05 and 0.24 ± 0.03 at Day 3 and Day 7, respectively) compared with those at Day 0 (cell circularity of 0.84 ± 0.06), which indicates that 3T3h supports the viability as well as differentiation of C2C12 cells.
Figure 7. Biocompatibility of 3T3h printed constructs. (a) Live (green)/dead (red) staining of C2C12 cells encapsulated in 3T3h at Day 0, Day 3, and Day 7 (Scale bar = 200 μm); (b) Cell viability at Day 0, Day 3, and Day 7 (n = 3); (c) Cell circularity at Day 0, Day 3, and Day 7. The values were taken as the average of cell circularity of 30 cells using image J. * (P < 0.05) and ** (P < 0.01) indicate a significant difference.
Download figure:
Standard image High-resolution imageThese results demonstrate that manipulating MTGase catalysis to induce partial crosslinking is a viable strategy to develop a GelMA bioink that fulfils both high cell compatibility as well as printability via extrusion-based printing. MTGase's function in the modification of protein properties is widely reported in food processing industries [34]; however, little attention has been paid in other sectors such as 3D bioprinting. MTGase is an efficient biocatalyst with attractive characteristics including site specificity, favourable kinetics, ease of use, and cost-effectiveness [35]. Any natural or synthetic protein, containing glutamine and lysine residues, can potentially serve as a substrate for MTGase. This enzyme-catalyzed site-specific crosslinking expands GelMA chains and converts them to high molecular weight polymers through the formation of covalent crosslinks without any unwanted side reactions and compromised cell viability of encapsulated cells. Besides, the enzymatic crosslinking is easily controlled by conducting a thermal treatment to inactivate the MTGase after customising the desired rheological properties. This allows the bioprinting process to be operated at room temperature; therefore, cells are exposed to one moderate temperature during the printing process. Furthermore, transglutaminase-induced GelMA bioinks displayed outstanding shear thinning properties and good printability in extrusion-based printing at ambient temperature and provided good cell viability as well. In these respects, the transglutaminase-induced controllable crosslinking system of GelMA holds promising potential for bioprinting.
4. Conclusion
In summary, we have developed a strategy to print photo-crosslinkable GelMA with highly tunable rheological properties, shear thinning capability, and sequential crosslinking ability towards long-term stable constructs without compromising cell viability and biocompatibility. Rheological properties of the GelMA solution could be tailored by manipulating the MTGase concentration and incubation time. Additionally, to maintain a stable rheological property, the activity of MTGase treatment was inhibited after appropriate rheological properties were obtained. The MTGase-induced GelMA bioink was then used to fabricate a 3D cell-laden structure at room temperature via extrusion-based bioprinting. High cell viability right after printing validated that the printing process using the 3T3h bioink did not adversely affect the cells. The C2C12 cells encapsulated in the structure became elongated after 3 d of culture, which indicated the biocompatibility and support of C2C12 differentiation within the new bioink. Based on these results, the controlled treatment of MTGase on GelMA could be a practical method to improve GelMA's printability at ambient temperature in 3D bioprinting. This strategy could significantly widen the usability of GelMA for different types of soft tissue bioprinting.
Acknowledgments
The authors gratefully acknowledge the National Research Foundation (NRF) Competitive Research Programme (NRF-CRP10-2012-07) and Ministry of Education (MOE) Singapore, Tier 1 (RG46/18) for the financial support. BHL is grateful for the support from the Wenzhou Institute of Biomaterial and Engineering (WIBEZD2017010-03) and Zhejiang Provincial Natural Science Foundation of China (LGF19E030001).