Abstract
Composite 3D scaffolds combining natural polymers and bioceramics are promising candidates for bone tissue engineering (BTE). Zein, as a natural plant protein, offers several advantages, including biocompatibility, adequate strength properties, and low/no immunogenicity; however, it lacks bioactivity. Thus, composite zein: bioactive glass (BG) scaffolds are proposed as promising candidate for BTE applications, with silver-doping of bioactive glass providing an antibacterial effect against possible post-implantation infection. Therefore, the aim of this study was to investigate the in vitro antibacterial properties, biocompatibility, bioactivity and compressive strength of zein scaffolds containing silver-doped bioactive glass. BG nanoparticles, undoped and Ag-doped, were fabricated using the sol-gel method. 3D composite zein:BG scaffolds, containing 20 wt% BG, were prepared and their antibacterial activity against Escherichia coli (E. coli) and Staphylococcus aureus (S. aureus) was assessed using the disc diffusion assay. Human osteoblast-like MG-63 cells were used to evaluate the in vitro biocompatibility of the prepared scaffold groups. In addition, the compressive strength of the scaffolds was determined using uniaxial compression strength testing and the scaffold interconnected porosity was measured using helium pycnometer. Disc diffusion assay showed that only zein scaffolds containing Ag-doped sol-gel BG are antibacterially positive against E. coli and S. aureus. Pure zein scaffolds and zein scaffolds containing sol-gel-derived BG showed no negative influence on the growth of MG-63 cells, as evident by the cells' ability to survive, proliferate, and function on these scaffolds. Moreover, incorporating sol-gel-derived BG into zein scaffolds at zein:BG of 80:20 ratio showed bioactive properties with adequate porosity without affecting the scaffolds' compressive strengths, which was similar to that of trabecular bone, suggesting that the new composites have potential for BTE applications in non-loaded bearing areas.
Export citation and abstract BibTeX RIS
1. Introduction
Tissue engineering (TE) is an effective treatment modality for regeneration of bone defects larger than critical defect size. The simplest paradigm of TE utilizes a scaffold as temporary extracellular matrix to support cell attachment and proliferation. For the TE scaffold to perform this critical function, it should fulfill certain requirements: allow and facilitate cellular infiltration and in-growth of newly formed tissues by having proper pore structure, size, interconnectivity and total porosity [1]. Scaffolds should also be biodegradable with a degradation rate matching the new tissue formation rate [1–3]. They should further possess suitable mechanical properties to provide temporary mechanical support; however, increased mechanical properties require dense scaffolds, while enhanced cell in-growth and tissue regeneration require porous scaffolds [4].
The use of a single material to satisfy these numerous requirements is not possible; therefore, composite systems, which combine the advantages of different materials, are more promising [5]. One such class of composites comprises natural polymers and bioceramics to benefit from the flexibility and easy shaping capability of polymers with the higher strength, stiffness, and bioactivity of the bioactive inorganic fillers, thus achieving the best possible mechanical and biological performance [5–9].
Naturally derived biopolymers are mainly proteins (for example collagen, gelatin, zein and silk) and polysaccharides (e.g. starch, alginate, cellulose, chitosan, etc) [1]. Zein, one of the plant proteins currently of interest, is a major storage protein of corn and an alcohol-soluble protein, but insoluble in water. Zein shows a promising potential for tissue engineering and drug delivery applications owing to its availability, good mechanical properties and no immunogenicity compared to animal-derived proteins such as collagen [1, 2, 10–13].
Zein protein has been proven to be biocompatible with endothelial cells of human umbilical veins [14], human liver cells [15], mice fibroblast cells [15], rat bone mesenchymal stem cells [12], human bone marrow stromal cells [13], and human periodontal ligament cells [16]. Thus, zein shows promising potential as a scaffold for TE applications. However, zein lacks bioactive behavior in simulated body fluid (SBF) [11, 17, 18], e.g. the induction of the growth of a hydroxyapatite (HA) phase on the material surface in contact with biological fluids, which is often used to characterize in vitro the bone-bonding ability of a material [19].
A characteristic feature of bioactive materials, including bioactive glasses (BGs) and calcium phosphate (CaP) ceramics, is the time-dependent and dynamic interactions that occur at the surface upon contact with biological fluids. The formation of highly reactive carbonated HA layer (HCA) provides the bonding interface with bone as well as soft tissues for special compositions [20]. The bioactivity of BGs is achieved by releasing critical concentrations of biologically active soluble silicon (Si), calcium (Ca), phosphorus (P), and sodium (Na) ions, during BG controlled dissolution at the rate needed for cell proliferation and differentiation [21].
Silver ions (Ag+) have a well-documented broad antibacterial effect [22–28], which is considered a promising solution to post-implantation bacterial infections, which often complicates wound healing. The incorporation of Ag+ ions in BGs has been the subject of considerable research efforts [23–26, 29–33]. In this study, we hypothesize that the addition of Ag+ ions into BG and the incorporation of this BG into zein scaffolds will benefit from the favorable biocompatibility and mechanical properties of zein, the bioactivity of the glass, and the antibacterial action of silver. Therefore, the aim of the current study is to investigate the in vitro antibacterial activity, biocompatibility, bioactivity and compressive strength of a new family of zein scaffolds containing silver-doped bioactive glass.
2. Materials and methods
2.1. Bioactive glass powder preparation
Undoped (BG) and 5 wt% Ag-doped (Ag-BG) were prepared via the sol-gel process according to the method described by El-Kady et al [24] with some modifications. 5 wt% Ag-doped BG with the composition (wt%) of 58 SiO2, 28 CaO, 9 P2O5, and 5 Ag2O was prepared by sequential mixing of tetraethyl orthosilicate (TEOS) (Sigma-Aldrich, Germany), distilled water, and 2 M nitric acid (VWR Chemicals, Germany) in ethanol. The mixture was stirred for 60 min at room temperature under continuous magnetic stirring to allow the acid hydrolysis of TEOS. Followed by the sequential addition of triethyl phosphate (TEP) (Merck, Germany), calcium nitrate tetrahydrate Ca(NO3)24H2O (Sigma-Aldrich, Germany) and silver nitrate AgNO3 (VWR Chemicals, Germany), allowing 60 min for each chemical to react. After the final addition, the mixture was stirred for 60 min to allow the completion of hydrolysis. 2 M ammonia solution (a gelation catalyst) was added dropwise to the mixture while vigorously stirring. Finally, the resulting gel was dried at 60 °C for one day. The dried gel powders were calcined at 700 °C for 2 h at a heating rate of 2 °C min−1.
2.2. Fabrication of zein scaffolds
A particle leaching process was employed using sodium chloride (NaCl) as porogen. For fabrication of pure zein scaffolds, zein powder (Sigma-Aldrich, Germany) was mixed with NaCl particles (100–125 μm) at a ratio of 1:2 (zein:NaCl). For fabrication of the zein-BG scaffolds, 20 wt% of the prepared sol-gel-BGs was added to the zein and NaCl mixture. The mixture was compressed into pellets (10 mm diameter, 6.5 mm height for bioactivity and mechanical tests/10 mm diameter, 2.35 mm height for antibacterial and cell culture tests), using an electrohydraulic pressing device applying a load of 2 × 104 Pa. The pellets were salt-leached in a hot water bath at 80 °C for 2 h under magnetic stirring. Later, the scaffolds were washed in distilled water, frozen for 24 h at −20 °C and afterwards freeze-dried.
2.3. Scaffolds morphology, microstructure, and Fourier transform infrared (FTIR) analysis
Characterization of the scaffolds morphology and microstructure was performed using high resolution scanning electron microscopy (SEM, Auriga, Zeiss, Germany). The prepared scaffolds were fixed to SEM sample holders, using a conductive silver adhesive paste and Au-sputter coated at a current of 20 mA for 40 s. Chemical structure analysis was performed using Fourier transform infrared spectroscopy (FTIR, IRAffinity-1S Fourier Transform Infrared Spectrophotometer, SHIMADZU, Japan).
2.4. In vitro bioactivity of scaffolds
The in vitro HA forming ability of the prepared scaffolds was investigated. Each scaffold was placed in 50 ml Kokubo simulated body fluid (SBF) [19] in airtight polyethylene bottles and placed in a shaking incubator at 37 °C and 90 rpm. At 7 and 14 days, samples were removed from SBF, washed gently with distilled water to stop any further reactions, and dried at 60 °C for 24 h. The microstructure of formed HA was observed by SEM; chemical structure analysis was performed by FTIR.
2.5. Antibacterial tests
The antibacterial activity of the Ag-doped BG-containing scaffolds, using undoped BG-containing scaffolds and pure zein scaffolds as control, against Escherichia coli (E. coli) and Staphylococcus aureus (S. aureus) was investigated by the disc diffusion method (BSAC Disc Diffusion Method for Antimicrobial Susceptibility Testing, Version 4, 2005) [34]. Luria broth (LB) (Luria/Miller) agar plates were inoculated with a standardized culture of S. aureus (NCTC 6571) and E. coli (NCTC 10418), 20 μl inoculum was spread evenly over the entire surface of the plate and left to dry for a few minutes before placing the scaffolds. Scaffold discs of 10 mm diameter and 3–4 mm thickness (n = 8) were then applied to the surface of agar plates; these plates were incubated for 24 h at 37 °C. After 24 h, the formation of clear zones of inhibition around the scaffolds, if any, was observed.
2.6. In vitro biocompatibility tests
The human osteosarcoma cell line MG-63 (Sigma-Aldrich) was used for in vitro cell culturing. Cells were cultured in Dulbecco's modified eagle medium (DMEM) (Thermo Fisher Scientific, Germany), memented with 10 vol% of fetal bovine serum (Sigma-Aldrich, Germany) and 1 vol% of penicillin-streptomycin (Gibco, Thermo Scientific™, Germany) and incubated at 37 °C, 5% CO2, and 95% humidity until confluence.
Each of the three scaffold groups (pure zein, zein:BG and zein:Ag-BG,) was placed in a 24-well plate; disinfection was done for one hour by ultraviolet (UV) light, then washed with sterile Dulbecco's phosphate-buffered saline (Thermo Fisher Scientific, Germany) and preconditioned in culture medium for two days. Scaffolds were seeded with 1 ml of cell suspension containing 100 000 cells and culture medium was changed twice a week.
2.6.1. Cell viability
At predetermined time points (1, 3, 7, and 14 days), MG-63 cell viability was measured using WST-8 cell counting kit-8 (CCK-8, Sigma-Aldrich, Germany). To avoid color changes due to pH fluctuations, which can affect result accuracy, DMEM without phenol red was used (Sigma-Aldrich, Germany). At each time point, the medium was removed, and the scaffolds with cells were washed with phosphate buffered saline (PBS). After PBS removal, culture medium containing 1 vol% WST-8 was added to each well and incubated at 37 °C for 4 h. Then, the supernatant from each sample was collected and transferred to a 96-well plate (100 μl in each well). The absorbance was measured at 450 nm using a microplate reader (PHOmo, Autobio Labtec Instruments). The WST-8 assay was conducted in triplicate.
2.6.2. Alkaline phosphatase (ALP) activity
The specific ALP enzyme activity assay can be used as a simple marker for osteogenic differentiation. MG-63 cells were seeded on the three scaffold groups (pure zein, zein:BG, zein:Ag-BG) under the same culture condition as described before. ALP enzyme activity of the cells on the scaffolds was measured at different time points (1, 3, 7, and 14 days).
At each time point, cells on the scaffolds were lysed with 1 ml cell lysis buffer containing 20 mM TRIS buffered solution (VWR Chemicals, Germany), 0.1 wt% Triton X-100 (AMRESCO, USA), 1 mM MgCl2, and 0.1 mM ZnCl2 (Sigma-Aldrich, Germany). The lysates were collected and centrifuged, 250 μl of the supernatant was added to 100 μl of ALP buffer solution (0.1 M Tris, 2 mM MgCl2 and 9 mM p-NPP (Sigma-Aldrich, Germany), pH = 9.8) and incubated at 37 °C for 4.5 h. The reaction was stopped by adding 650 μl of 1 M NaOH, and absorbance was measured spectrometrically at 405 and 690 nm (background absorbance). The specific ALP activity was calculated with respect to the incubation time and the total protein content of the cell lysates. The total protein content was measured by Bradford reagent (Sigma-Aldrich, Germany). The specific ALP activity assay was conducted in triplicate.
2.6.3. Mineralization assay
Alizarin red S staining was used for qualitative evaluation of the extracellular mineralization, CaP mineral nodule formation. Calcium forms an Alizarin Red S-calcium complex in a chelation process, and the formed calcium deposits have an orange–red color. Calcium deposits are an indication of successful in vitro bone formation [35]. At day 14, the medium was removed and the samples were washed with PBS, fixed in 70% ethanol, and stained with 40 mM Alizarin Red S (Sigma-Aldrich, Germany) at pH 4.2 for 10 min. Stained samples were rinsed with Nanopure water followed by a 15 min wash with PBS (to remove nonspecific stain, not associated with calcium mineral deposits). Stained samples were imaged for qualitative analysis using a stereomicroscope (Stemi 508 Stereo Microscope, Zeiss, Germany) checking for any orange–red colored deposits. The mineralization assay was conducted in duplicate.
2.7. Compressive strength
To evaluate the potential of zein and zein-BG scaffolds for bone tissue engineering applications, the compressive strength of the three scaffold groups (five replicates per group) was measured. Cylindrical scaffolds, 10 mm in diameter and 10 mm height [36–38], were subjected to uniaxial compression testing (Z050, Zwick Roell, Germany) at a crosshead speed of 1 mm min−1; a preload of 0.1 N and maximum applied force of 1 kN until densification of the specimens occurred.
2.8. Porosity measurement
Interconnected porosity (Øc) of the prepared scaffolds was measured using helium injection at 1.2 bar pressure using a gas pycnometer (Ultrapyc 1200e Helium Pycnometer; Quantachrome Instruments, USA). The bulk volume and dry weight of six replicates from each of the scaffolds groups were measured using a precision caliper (0.01 mm precision) and an electronic balance (0.1 mg precision) to calculate the bulk density of the samples (ρb). The true volume (vt) of each sample was measured using the helium pycnometer to calculate the true (skeletal) density of the samples (ρt)
Three measurements were taken for each sample and the final arithmetic mean was then calculated and used in further calculations. The interconnected porosity (ØHe) was calculated using the following equation [39, 40]:
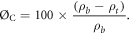
2.9. Statistical analysis
All data were expressed as mean ± standard deviation. The data were checked using the Shapiro–Wilk test for normal distribution. For normally distributed variables, comparison between the mean of the different materials was carried out using parametric one-way analysis of variance (ANOVA) with a Tukey's post hoc multiple comparison of the mean. Non-normally distributed data were tested using non-parametric Kruskal–Wallis test with Mann–Whitney U test post hoc for pairwise comparisons. A p-value ≤ 0.05 was considered statistically significant. All tests were two-nano-sized in diametertailed.
3. Results
3.1. Morphology and microstructure of scaffolds
SEM micrographs of pure zein, zein:BG, and zein:Ag-BG (figure 1) show that all the different scaffold groups are porous. SEM as 2D pore structure analysis method shows that the scaffolds had a wide range of pore diameter with large-sized pores in the range of 100 μm, medium-sized pores of 50 μm, and small-sized pores, which are nano-sized in diameter found in the scaffold struts. In zein scaffolds containing the sol-gel-derived BGs (BG and Ag-BG), the added BG powder is not homogenously distributed within the zein scaffolds; instead, they are aggregated as clusters with a distinct interface between the zein and the added BGs (figures 1(b.2) and (c.2)).
Figure 1. SEM micrographs of the outer surface of a pure zein scaffold (a), zein:BG (b), and zein:Ag-BG (c) at low and high magnifications. White arrows show zein, black arrows show BG.
Download figure:
Standard image High-resolution image3.2. FTIR spectra analysis
FTIR spectra of pure zein scaffolds (figure 2) show the three characteristic amide peaks for zein protein at around 1630, 1520, and 1230 cm−1, which correspond to amide I, amide II, and amide III, respectively [1, 11]. Zein:BG scaffolds spectra (the upper two spectra in figure 2) show additional peaks characteristic of SiO2-based glasses, which are presented as three main vibrational modes of the Si–O–Si groups in the region between 400 and 1300 cm−1 [41–43]. Two additional peaks of the phosphate group (PO43−) are observed at around 565 and 601 cm−1 [41, 42].
Figure 2. FTIR spectra of the as-prepared zein:BG scaffolds versus pure zein scaffold. (Peaks are discussed in the text.)
Download figure:
Standard image High-resolution image3.3. In vitro bioactivity of scaffolds
SEM micrographs of pure zein scaffolds incubated in SBF (figure 3) show no HA formation on the surface of zein, neither after 7 nor 14 days. This result was also confirmed by the FTIR spectral analysis of incubated zein scaffolds, which show no change in peaks compared to the as-prepared scaffolds (figure 4(a)).
Figure 3. SEM micrographs of pure zein, zein:BG, and zein:Ag-BG scaffolds incubated in SBF for 7 and 14 days. White arrows show areas of zein not covered with HA; black arrow show areas of HA crystals.
Download figure:
Standard image High-resolution imageFigure 4. FTIR spectra of (a) pure zein, (b) zein:BG, and (c) zein:Ag-BG scaffolds after incubation in SBF for 7 and 14 days.
Download figure:
Standard image High-resolution imageOn the other hand, SEM micrographs of zein scaffolds containing the sol-gel-derived BGs (zein:BG and zein:Ag-BG scaffolds) show the formation of rounded shaped HA crystals (figure 3). It is observed that the formed HA crystals do not homogenously cover the entire scaffold surface; however, they grow in the form of clusters only on the surface of BG leading to a distinct interface between the formed HA crystals and the adjacent zein surface. FTIR spectral analysis (figures 4(b) and (c)) shows the presence of new peaks characteristic of HA formation demonstrated by the increasing intensity of the P–O vibrational band, the appearance of a new carbonate (CO32−) peak at around 875 cm−1 and a weak silanol peak near 960 cm−1.
3.4. In vitro antibacterial activity
The results of the disc diffusion assay indicated that only zein scaffolds containing Ag-BG had positive antibacterial activity against E. coli and S. aureus as indicated by the formation of clear zones of inhibition around them (figure 5). However, other scaffold groups showed no antibacterial effect with absence of inhibition zone formation as evident by the ability of bacteria to grow around them.
Figure 5. Antibacterial activity of different scaffold groups: (1) pure zein, (2) zein:BG, and (3) zein:Ag-BG against E. coli (a) and S. aureus (b). Arrows show clear inhibition zones formed.
Download figure:
Standard image High-resolution image3.5. In vitro biocompatibility tests
3.5.1. Cell viability
Data from the WST-8 assay is shown in figure 6. At all-time points, zein:BG showed the highest mean cell viability. At day 1, 3, and 7, zein:BG scaffold groups showed the highest mean cell viability, which was significantly higher than the value for zein:Ag-BG (p ≤ 0.05), while it showed no significant difference compared to the pure zein scaffolds. At day 14, the zein:BG scaffold exhibited the highest mean value among all groups.
Figure 6. Viability of MG-63 cells (absorbance at 450 nm) at different time points cultured for the groups zein, zein:BG, and zein:Ag-BG. Error bars show the standard deviations (n = 3). Data were compared using Tukey's test and different letters in the same day represent statistically significant difference at p-value ≤ 0.05.
Download figure:
Standard image High-resolution imageResults showed significant statistical changes in the MG-63 cell count within each of the zein, zein:BG, and zein:Ag-BG groups at the different time points.
3.5.2. Specific ALP activity
Specific ALP enzyme activity assay revealed that zein:BG scaffolds show no significant difference in the specific ALP activity compared to other groups at most of the time points (day 1, 3, and 14) (figure 7). At day 7, the zein group shows the highest specific ALP activity among all groups (p ≤ 0.05). The results indicate significant changes in specific ALP activity of MG-63 cells cultured on the zein and zein:BG groups at different time points.
Figure 7. Specific ALP activity of MG-63 cells at different time points cultured on zein, zein:BG, and zein:Ag-BG scaffolds. Error bars show the standard deviations (n = 3). Data were compared using Tukey's test and different letters in the same day represent statistically significant difference at p-value ≤ 0.05.
Download figure:
Standard image High-resolution imageThe total protein content of the cell lysates (supplementary information S1 is available online at stacks.iop.org/BMM/13/065006/mmedia) showed no significant difference at day 1 of culture. At days 3, 7 and 14, the zein:BG scaffold group showed the significantly highest values, while the pure zein group showed statistically lower protein content than the two composite scaffold groups.
3.5.3. Mineralization assay
After 14 days of culture, more intense orange–red color staining was observed on zein:BG scaffolds (figure 8(b)) compared to pure zein (figure 8(a)) and zein:Ag-BG (figure 8(c)) scaffolds.
Figure 8. Alizarin red S staining of MG-63 cells cultured on pure zein (a), zein:BG (b), and zein:Ag-BG (c) scaffolds after 14 days.
Download figure:
Standard image High-resolution image3.6. Compressive strength
The mean compressive strength values showed no significant difference between the pure zein scaffolds and the composite scaffolds containing 20 wt% undoped and Ag-doped BG (table 1).
Table 1. Compressive strength values of the three scaffold groups. Data are represented as means (±SD) and compared using one-way ANOVA test (n = 5).
Compressive strength (MPa) | |
---|---|
Pure zein | 5 ± 2 |
Zein:BG | 5 ± 1 |
Zein:Ag-BG | 5 ± 2 |
p-value | 0.840 |
On the other hand, composite scaffolds containing 10 wt% Ag-doped BG showed significantly higher compressive strength than the pure zein group (supplementary information S2).
3.7. Porosity measurement
Statistically significant differences in mean interconnected porosity were found among the different groups (p ≤ 0.05). Zein:BG scaffolds exhibited the highest mean porosity while the total porosity of pure zein was statistically lower than that in the other two groups (table 2).
Table 2. Interconnected porosity (Øc) (in %) of different scaffolds. Data are represented as means (±SD) and compared using Tukey's test (n = 6).
Interconnected porosity (%) | |
---|---|
Pure zein | 67 ± 2c |
Zein:BG | 85 ± 3a |
Zein:Ag-BG | 80 ± 2b |
p-value | <0.0001* |
Note. Different letters represent statistically significant differences at p-value ≤ 0.05.
*Highly statistical significance at p-value ≤ 0.001.4. Discussion
Composite scaffolds combining natural polymers and bioceramics are promising candidates for hard tissue engineering. This is achieved by providing the best possible mechanical and biological performance and by combining the advantages of polymers, such as flexibility and easy shaping, with the higher strength, stiffness, and bioactivity of the bioactive inorganic fillers. Zein is one of the naturally-derived polymers currently of interest, based on its biocompatibility, suitable mechanical properties and availability, while bioactive glasses are well-known for their good bone-forming ability. The current study aimed at investigating the in vitro antibacterial properties, biocompatibility, bioactivity and compressive strength of novel 3D nanocomposite zein scaffolds containing undoped and silver-doped bioactive glass nanoparticles in comparison to pure zein scaffolds.
SEM micrographs showed the formation of zein and composite zein:BG scaffolds with highly interconnected porous networks (figure 1). The existence of macro and micro-sized pores was evident with the characteristic presence of micropores in the walls of the macropores, which was previously reported for zein scaffolds fabricated by the salt-leaching method [11, 12, 16, 17]. The SEM micrographs showed that the sol-gel-derived BG particles are not fully embedded in zein (figures 1(b.2)). This may be attributed to the hydrophilicity of the sol-gel-derived glasses and the hydrophobicity of zein [17].
FTIR spectral analysis of the as-prepared scaffolds (figure 2) showed the characteristic bands of zein in the pure zein scaffold. The amide I peak is associated with stretching of carbonyl (C=O) of the amide groups. The amide II peak corresponds to the angular deformation vibrations of the N–H bond. Finally, the amide III band reflects the axial deformation vibrations of the C–N bond [1, 11]. In zein:BG scaffolds, both bands characteristic of zein and silicate BG were observed. Silicate-based glasses are characterized by the three main vibrational modes of the Si–O–Si groups (between 400 and 1300 cm−1) [41–43]. The broad band located in the range of 1000–1200 cm−1 is associated with Si–O–Si asymmetric stretching vibration, whereas the band observed around 800 cm−1 corresponds to the Si–O–S symmetric stretching vibration. In addition, the band located around 450 cm−1 is identified as the Si–O–Si bending mode [41–43]. Vibrational peaks of the phosphate group observed at 565 and 601 cm−1 are associated with the P–O asymmetric bending mode in bioactive glasses containing phosphate groups [41, 42]. However, no new peaks were observed, which indicate the absence of chemical interaction between zein and the incorporated BG particles.
It is well-known that in vitro bioactivity (or bioreactivity) may be related to bone-bonding ability in vivo, considering that bioactive materials should provide a favorable environment for osteoblasts to proliferate, differentiate and produce apatite and collagen [19, 44]. The in vitro apatite-forming ability of biomaterials is commonly evaluated by incubating the samples in SBF to detect HCA layer formation [19]. The formation of HCA involves several stages: including an initial rapid exchange of alkali ions with hydrogen ions in the body fluids (or SBF), followed by silica network dissolution and formation of Si–OH (silanols) bonds, which is followed by silica gel polymerization, which enhances HA crystals nucleation, and final crystallization of HCA layer through the incorporation of hydroxyls (OH−) and carbonate () from the solution [45].
After incubation of pure zein scaffolds in SBF, SEM micrographs showed lack of HA crystal formation, even after 14 days of incubation in SBF (figure 3). These findings were confirmed by FTIR analysis, where no new peaks were observed compared to the as-prepared scaffolds (figure 4(a)), which indicates that zein lacks bioactive behavior in SBF [11, 17, 18]. The lack of mineralization of zein upon incubation in SBF could be attributed to its hydrophobicity [11], the lack of relevant elements (Ca, P) and the slow dissolution of zein in SBF, which hinders the formation of HA [11, 18].
Zein scaffolds containing both undoped and silver-doped sol-gel BGs showed evidence of mineralization and formation of the characteristic morphology of HA crystals at both 7 and 14 days (figure 3). Bioactivity of the incubated scaffolds was confirmed by FTIR analysis, which showed the existence of new peaks characteristic of HCA layer formation, as shown by the increasing intensity of the P–O vibrational band (565 and 601 cm−1) [42, 43]. In addition, the new carbonate () peak observed at around 875 cm−1 suggests the formation of HCA, which resembles the mineralized inorganic component of bone [42, 46, 47] as well as the weak silanol peak observed near 960 cm−1, is related to the bioactive surface reactions occurring in contact with SBF [41, 42] (figures 4(b) + (c)).
It is evident from SEM micrographs that newly formed HA crystals have grown on top of BG particles only, which confirms that the added BG is the only constituent responsible for the bioactive behavior of the composite scaffolds (figure 3). The mechanism of HA formation is suggested to be based on the rapid initial release of Ca2+ from the BG, coupled with an increase in local pH, which attacks the silica glass network and subsequent formation of silica-rich layer [48, 49]. This layer enhances the formation of the HA nuclei crystallization from the high concentration of Ca2+ and PO43− present [47].
Disc diffusion assay results showed that only zein:Ag-BG scaffolds were antibacterial against E. coli and S. aureus (figure 5). The antibacterial activity of these scaffolds could be attributed to the leaching out of Ag+ ions from the glass matrix. The antibacterial activity of silver nanoparticles is well-documented [22–28, 50], and the release of silver ions from the scaffolds is a diffusion-controlled mechanism and depends on the BG content of the scaffolds [48]. The addition of Ag-doped BG nanoparticles as filler in these nanocomposite scaffolds is aimed primarily at imparting antibacterial activity to the scaffolds through releasing antibacterial silver ions during their degradation. Scaffolds containing lower Ag-doped BG ratio (10 wt%), failed to show antibacterial effect with absence of inhibition zone formation (data not shown), which could be due to the lower amount of silver ions released, incapable of inhibiting bacterial growth.
Cellular attachment and interaction with the scaffold surfaces are essential processes for cell proliferation, differentiation and their normal functions [51]. Thus, MG-63 cell viability, differentiation and mineralization were assessed using WST-8, specific ALP activity, and Alizarin Red S staining assays, respectively.
Zein protein has been proven to be biocompatible in previous in vitro and in vivo investigations [3, 12–16, 52, 53]. In the current study, scaffolds were preconditioned in culture medium for two days before seeding the cells; this was performed because BGs, particularly the sol-gel derived, release a high initial burst concentration of Ca ions following their immersion in physiological fluids with a pH rise in the first three days [54]. Such burst Ca release and pH change have been reported to adversely affect cell attachment and growth [35, 54, 55].
WST-8 assay at day 1, 3, 7, and 14 of culture showed the ability of MG-63 cells to proliferate on the pure zein scaffolds and zein scaffolds containing sol-gel-derived BGs (figure 6). At day 14, zein:BG scaffolds exhibited the highest mean cell count among all groups. However, both pure zein and zein:Ag-BG were proven suitable for supporting MG-63 cell viability and growth and had no negative effect on proliferation. A possible explanation of the enhanced cellular proliferation with zein:BG scaffolds would be the markedly higher specific surface area of the sol-gel-derived glasses with subsequent higher dissolution and ion release rate and higher bioactivity. The higher specific surface area could provide more sites for adsorption of proteins and growth factors, enhancing the subsequent cell attachment and proliferation. In addition, the effect of Si species released during BG dissolution on enhancing osteoblast proliferation and activity, e.g. gene expression, have been previously reported [51, 56, 57]. The lower proliferation of MG-63 on zein:Ag-BG scaffolds could be induced by the release of Ag+ ions, which could have a possible slight cytotoxic effect in such static culture conditions. Further studies using dynamic culture conditions, which would provide better nutrient and mass transfer, could show different cellular response.
Specific ALP is the most widely recognized marker of osteoblastic differentiation and activity [51, 58–60]. ALP is an essential enzyme for mineralization, associated with the plasma membrane of osteoblasts, which is present in high levels in matrix vesicles (MV) observed in developing bone [58, 61]. The differentiation behavior and activity of MG-63 cells on the scaffolds were investigated using specific ALP activity assay. Results showed that ALP activity followed a different trend to the proliferation behavior observed with WST-8 assay, where no statistically significant difference in specific ALP activity was observed among the investigated groups at most time points, except for day 7 (figure 7). An abrupt decrease in ALP activity on zein:BG scaffolds was observed after day 1, although cells cultured on the zein:BG group showed significantly higher total protein content (at days 3, 7 and 14), which indicates higher cellular proliferation compared to pure zein and zein:Ag-BG groups (supplementary information S1). Similar findings were reported by Sadat-Shojai et al [62], who used mouse pre-osteoblastic MC3T3-E1 cells, and Nuttelman et al [63], who used human mesenchymal stem cells, where ALP activity decreased with progressive increase in mineralization.
A possible explanation of the decreased ALP activity with progressive mineralization was proposed by Genge et al [61], who reported that a loss in ALP activity (up to 65%–70%) may accompany Ca2+ accumulation during MV-mediated mineralization. Such decline in ALP activity was suggested to be the result of the combined action of several factors, including loss of enzyme activity through adsorption of the mineral to the enzyme. Other factors include loss of zinc (Zn2+) and magnesium (Mg2+) metal ions from the active site of the enzyme. ALP enzyme is a metalloenzyme with both Zn2+ and Mg2+ at its active site, their loss might result in irreversible denaturation of the enzyme during mineralization [61].
Osteoblast differentiation either in vitro or in vivo can be characterized in three stages: cell proliferation, matrix maturation, and matrix mineralization [64]. Alizarin red S staining assay used to evaluate extracellular mineralization after 14 days of culture showed mineral deposition on the three scaffold groups (figure 8). However, pure zein (figure 8(a)) and zein:Ag-BG (figure 8(c)) groups showed little mineral deposition, as indicated by nodules of orange–red color formed (Alizarin red S positive nodules), while zein:BG (figure 8(b)) showed markedly abundant heavily stained Alizarin Red S positive nodules, suggesting a higher extent of mineralization. The higher MG-63 mineralization on zein:BG scaffolds indicates the higher activity of cells, which suggests the promising potential of these scaffolds for bone tissue engineering [35]. Mineral nodule formation on zein:Ag-BG scaffolds was also evident, although to a less extent than that formed on zein:BG scaffolds. The lower mineralization activity of MG-63 on zein:Ag-BG scaffolds could be induced by the release of Ag+ ions, as discussed earlier.
Among the requirements of BTE scaffolds, mechanical properties are of prime importance; the implanted scaffold should exhibit adequate mechanical stability enabling a suitable environment for new bone tissue formation [65]. BTE scaffolds should provide and maintain structural support during cellular proliferation and subsequent mineralization, leaving space for new bone tissue growth during scaffold degradation [3]. The compressive strength values of the investigated groups (table 1) were in the range of those of cancellous bone (2–12 MPa for compressive strength) [12], which indicates their suitability for BTE in non-load bearing areas. There was no significant difference between the compressive strength values of the three fabricated groups, pure zein, zein:BG, and zein:Ag-BG. The addition of sol-gel-derived nano-sized BG (in 20 wt%) did not affect the relatively good mechanical properties of zein scaffolds.
The addition of 10 wt% Ag-doped BG significantly enhanced the compressive strength of the prepared scaffolds, compared to the pure zein scaffolds (supplementary information S2). However, increasing the amount of the added BGs to 20 wt% to impart antibacterial activity to the prepared scaffolds was needed, which resulted in decrease in the mechanical strength of the composite scaffolds. Higher ratios of BG fillers have been often reported to result in a decrease of composite scaffolds compressive strength and stiffness [11, 17]. The interference of the added BG with zein plasticization process during salt-leaching could be the cause for such decrease [17]. However, it is noteworthy that the addition of sol-gel-derived BG (in 20 wt% concentration) in the present study did not affect the relatively good mechanical properties of zein scaffolds.
The interconnected porosity (Øc) of the prepared pure zein, zein:BG, and zein:Ag-BG scaffolds was analyzed using helium pycnometer. This method was selected among the different methods available for porosity characterization as Archimedes' method is not suitable for the hydrophobic zein polymer [66], and the theoretical gravimetric method necessitates accurate determination of the true density of the scaffold material. The density of zein (1.22 g cm−3) commonly used with this method [17] and in the apparent density method [3, 12, 13, 67] is not an accurate representative of the true zein density after the plasticization and salt-leaching method which affect the degree of crystallinity of the polymer. Hence, gas pycnometry, using helium as the analysis gas, was used for determination of the open porosity of the prepared scaffolds.
Scaffolds for TE applications require a total porosity of at least 70%, which should be highly interconnected to aid in the delivery of nutrients to the cells and removal of metabolic waste in addition to support tissue in-growth [68]. High pore interconnectivity facilitates uniform cell seeding and migration and allows for nutrients diffusion into and metabolites diffusion out of the TE construct [65]. The measurement of the mean interconnected porosity showed that there was statistically significant difference among the three scaffold groups (table 2).
The porous structure is created during the leaching of the NaCl porogens during the salt-leaching process. The higher mean porosity of the zein:sol-gel-derived groups, compared to pure zein scaffolds, could be attributed to the possible dissolution of some of the BG particles during the leaching process [17]. The higher porosity of zein:BG group compared to the zein:Ag-BG group might be explained by the fact that the BG powder had higher volume/mass ratio (lower density) compared to the Ag-BG powder [69]. As a result, the amount of Ag-BG particles incorporated in zein scaffolds was lower than that of undoped BG, hence, a possible explanation for the lower open porosity of zein:Ag-BG scaffolds is the dissolution of lower amount of Ag-BG than BG.
5. Conclusions
Zein scaffolds containing undoped and Ag-doped sol-gel-derived BG were shown to offer in vitro biocompatibility, exhibiting comparable mechanical properties, in addition to providing enhanced in vitro bioactive properties. Moreover, addition of Ag-doped sol-gel-derived BG to the zein scaffolds induces additional antibacterial activity against E. coli and S. aureus microorganisms. Future studies must evaluate these newly prepared scaffolds in vivo.
Acknowledgments
The authors would like to thank Professor Bassem Nabawy for his experienced and valuable assistance with the porosity measurement. They also thank Alina Grünewald for her valuable technical assistance with cell culture. Financial support provided by the Egyptian Ministry of Higher Education (MoHE) and the German Academic Exchange Service (DAAD) for Aiah A El-Rashidy is acknowledged.