Abstract
Objective. Impaired trunk stability is frequent in spinal cord injury (SCI), but there is a lack of quantitative measures for assessing trunk function. Our objectives were to: (a) evaluate trunk muscle activity and movement patterns during a reaching task in SCI patients, (b) compare the impact of cervical (cSCI) and thoracic (tSCI) injuries in trunk function, and (c) investigate the effects of a startling acoustic stimulus (SAS) in these patients. Approach. Electromyographic (EMG) and smartphone accelerometer data were recorded from 15 cSCI patients, nine tSCI patients, and 24 healthy controls, during a reaching task requiring trunk tilting. We calculated the response time (RespT) until pressing a target button, EMG onset latencies and amplitudes, and trunk tilt, lateral deviation, and other movement features from accelerometry. Statistical analysis was applied to analyze the effects of group (cSCI, tSCI, control) and condition (SAS, non-SAS) in each outcome measure. Main results. SCI patients, especially those with cSCI, presented significantly longer RespT and EMG onset latencies than controls. Moreover, in SCI patients, forward trunk tilt was accompanied by significant lateral deviation. RespT and EMG latencies were remarkably shortened by the SAS (the so-called StartReact effect) in tSCI patients and controls, but not in cSCI patients, who also showed higher variability. Significance. The combination of EMG and smartphone accelerometer data can provide quantitative measures for the assessment of trunk function in SCI. Our results show deficits in postural control and compensatory strategies employed by SCI patients, including delayed responses and higher lateral deviations, possibly to improve sitting balance. This is the first study investigating the StartReact responses in trunk muscles in SCI patients and shows that the SAS significantly accelerates RespT in tSCI, but not in cSCI, suggesting an increased cortical control exerted by these patients.
Export citation and abstract BibTeX RIS

Original content from this work may be used under the terms of the Creative Commons Attribution 4.0 license. Any further distribution of this work must maintain attribution to the author(s) and the title of the work, journal citation and DOI.
1. Introduction
Trunk stability is a complex sensorimotor function necessary to maintain a balanced upright posture, both under static and dynamic loading conditions [1]. This requires an accurate control by the central nervous system to integrate proprioceptive information and coordinate abdominal, pelvic, and spinal muscle groups against extrinsic or intrinsic destabilizing forces [1, 2]. These motor commands are in most cases part of the motor plan to achieve an effective task-specific postural control and consist of a package of instructions to counteract perturbations and regulate deviations from a set position through postural adjustments relying on anticipatory and compensatory mechanisms, as well as real-time feedback from sensory inputs [2, 3].
Spinal cord injury (SCI) is a condition that causes impairment of motor and sensory functions below the injury and results in many health complications. A common consequence of cervical and high thoracic SCI is the lack of control over trunk muscles, leading to deficits in postural control and balance while sitting [4, 5]. Trunk stability is essential for sitting and standing balance, but also to support functional limb movements [5, 6], being a necessary component in activities of daily living (ADLs) such as eating, dressing, and transferring [7]. For these reasons, impaired trunk control sustained by patients with SCI constitutes a major cause of motor disability, which affects their independence and quality of life, and also increases the healthcare costs [8]. Actually, postural rehabilitation to improve trunk function has been identified as one of the highest priorities for optimizing the recovery process and increase the level of autonomy of individuals with SCI [9]. However, despite the importance of trunk function for balance and functional performance, trunk stability is rarely examined in studies of mobility after SCI [7]. One of the reasons is the lack of objective, standardized measures to assess trunk function.
The most common methods for the evaluation of trunk function and sitting balance after SCI are observational clinical tests, such as the Trunk Control Test [5], the Ottawa Sitting Scale [10], the Sitting Balance Measure [11], and the Modified Functional Reach Test [12]. More information about these tests and their validity can be found in a recent systematic review on unsupported sitting balance in subjects with SCI [13]. However, the main disadvantage of these tests is that they are qualitative assessment methods, as they depend on the clinician performing the test and are therefore prone to subjectivity. On the other hand, quantitative measures of trunk performance can be obtained using biomedical instrumentation, and some authors have tested their application in patients with SCI in the laboratory setting. Most of these studies rely on motion capture technologies to record movement kinematics and then measure trunk orientation, trunk excursion, limits of stability, maximum reaching distance, or movement velocity, among other parameters [4, 14]. Surface electromyography (EMG) has also been proposed as an assessment tool to measure trunk muscle activity and coordination in patients with SCI [15–17]. Other studies have measured reaction forces and changes in the center of pressure (COP) with force plates on the seating surface, to investigate postural control and the limits of stability in individuals with SCI [14, 17, 18]. Dynamometers and inclinometers have also been used to measure trunk strength [7] and active cervical range of motion [19], respectively, in these patients.
A recent study investigated trunk flexion during a reaching task in healthy subjects [20]. In such a task, which is common in ADLs, trunk muscles play a double role, as they are involved both in postural control to prevent destabilization and in movement execution. In that study, a startling acoustic stimulus (SAS) was applied in some trials for its potentially destabilizing effects on trunk postural control while reaching. It was shown that the response time (RespT) was markedly shortened when the SAS was added to the imperative signal (IS) for task execution. This so-called StartReact effect appears when healthy subjects are highly prepared for voluntary movement execution [21, 22]. The StartReact effect has been described in a wide range of tasks, including finger [23] and hand [21] movements, arm reaching [22], step initiation [24], sit-to-stand maneuvers [25], obstacle avoidance during walking [26], and standing postural reactions [27]. The control mechanisms underlying this phenomenon may involve subcortical pathways such as the reticulospinal tract [21, 23], although some cortical processing cannot be ruled out [28–30]. Some of the strongest evidence for the subcortical explanation comes from studies involving clinical populations [31]. For instance, in an ankle dorsiflexion task, patients with pure hereditary spastic paraplegia (HSP) showed delayed voluntary responses attributable to the corticospinal damage, but a SAS accelerated their responses, completely normalizing their latencies [32]. The authors hypothesized that this intact StartReact effect, leading to faster and more normalized movements, occurred due to the release of a subcortically stored motor program conveyed by the preserved reticulospinal tract [32]. Similar results have been reported for patients with HSP in gait initiation [33], and other clinical populations with cortical deficits such as patients with chronic stroke in hand movements [34], ballistic elbow movements [35], reaching movements [36], and lower limb movements [37], or Parkinson's disease in elbow extension [38] and gait initiation [39]. Baker and Perez [40] examined the StartReact response to study the contribution of the reticulospinal tract in motor tasks requiring different degrees of hand dexterity in individuals with incomplete chronic cervical SCI, finding that reaction times were shorter when the SAS was presented while performing a power grip, but not index finger abduction or precision grip. To our knowledge, the study by Baker and Perez [40] is the only one so far reporting on the StartReact phenomenon in patients with SCI.
Despite the variety of studies on the StartReact effect, there have only been very few publications in tasks involving trunk muscles or movements [25, 27], and none of them in clinical populations. A previous study in healthy subjects [20] showed that the SAS markedly reduced the RespT and EMG onset latencies in both prime movers and postural muscles when performing a reaching task requiring trunk tilting. We considered worth examining how patients with SCI executed this task, as their trunk control impairment may impact performance, and whether this performance depended on the level of SCI (cervical or thoracic SCI). We were also interested in a StartReact paradigm for two main reasons: (a) to investigate the potentially destabilizing effect of the SAS in SCI patients, whose trunk stability is impaired, and examine how they compensate for the loss of balance to control their posture, and (b) to determine whether the StartReact effect was present in trunk muscles of SCI patients.
We hypothesize that trunk stability and sitting balance during the execution of the reaching task will be impaired in patients with SCI, and this will probably depend on the level and severity of SCI. Due to the higher level of the injury, patients with cervical SCI (cSCI) will likely perform slower and more unbalanced movements than patients with thoracic SCI (tSCI). However, it is not clear whether the SAS will accelerate the RespT in these two groups of individuals with impaired trunk stability or, on the contrary, it will induce a higher destabilization and thus hinder postural control and task execution and/or induce compensatory strategies. We hypothesize that the effect of the SAS will also be related to the injury level, so, presumably, the StartReact effect could be absent or reduced in patients with higher SCI level (i.e. cSCI), in comparison to those with less severe or lower SCI level (i.e. tSCI).
With all these ideas in mind, we embarked upon this study. Our main objectives were: (a) to quantitatively characterize trunk function during a reaching task in patients with SCI in terms of muscle activity and movement patterns, (b) to compare patients with cervical and thoracic SCI, and (c) to investigate the effects of a SAS both as a destabilizing stimulus and as trigger for the StartReact phenomenon in these patients. To accomplish these goals, we recorded EMG signals, to evaluate trunk muscle activation, and smartphone accelerometer data, to analyze movement patterns, as a simpler cost-effective alternative to motion capture systems. Then we extracted and analyzed different outcome measures to determine what information they can provide to quantitatively assess trunk function in individuals with cSCI or tSCI.
2. Materials and methods
2.1. Participants
Twenty-four patients with SCI (16 men, eight women, mean age 41 ± 16 years, range 19–71 years) and 24 age and gender matched healthy subjects (16 men, eight women, mean age 42 ± 14 years, range 20–69 years, p = 0.87), who served as control group (control), were recruited to participate in the study. The inclusion criteria were: clinical diagnosis of SCI, either traumatic or non-traumatic in origin, including complete and incomplete injuries, classified as AIS A, B, C, or D according to the American Spinal Injury Association Impairment Scale (AIS) [41]. Fifteen subjects had a cervical SCI (cSCI), with injury levels ranging from C4 to C8 (2 AIS A, 4 AIS B, 7 AIS C, 2 AIS D), while nine subjects had a thoracic SCI (tSCI), between levels T1 and T12 (5 AIS A, 2 AIS C, 2 AIS D). The time after injury ranged from 1 to 20 months. The clinical characteristics of all the individuals with SCI can be found in table 1. Exclusion criteria included pacemaker dependency, requiring for mechanical ventilatory support, arrhythmia and other cardiovascular conditions, any other neurological disorder, inability to lift the arm, hearing impairments, and other comorbidities that would contraindicate the test. Healthy subjects were excluded if they had any neurological or musculoskeletal disease.
Table 1. Clinical characteristics of the enrolled SCI patients.
Patient ID | Gender | Age (years) | Level | AIS | AIS motor score | SCIM | Etiology | Time after injury (months) |
---|---|---|---|---|---|---|---|---|
SCI 1 | M | 25 | C4 | A | 11 | 13 | Traumatic | 4.1 |
SCI 2 | M | 36 | C4 | B | 14 | 9 | Traumatic | 5.3 |
SCI 3 | M | 46 | C4 | C | 19 | 14 | Traumatic | 4.6 |
SCI 4 | F | 48 | C4 | D | 46 | 63 | Non-traumatic | 2.6 |
SCI 5 | M | 21 | C5 | A | 9 | 24 | Traumatic | 19.5 |
SCI 6 | F | 52 | C5 | C | 32 | 14 | Traumatic | 5.8 |
SCI 7 | F | 35 | C5 | C | 40 | 34 | Non-traumatic | 11.9 |
SCI 8 | M | 28 | C5 | C | 28 | 32 | Traumatic | 4.1 |
SCI 9 | M | 58 | C6 | C | 51 | 26 | Non-traumatic | 2.8 |
SCI 10 | M | 22 | C6 | C | 52 | 63 | Traumatic | 6.4 |
SCI 11 | M | 70 | C6 | D | 100 | 94 | Traumatic | 1.8 |
SCI 12 | M | 19 | C7 | B | 28 | 40 | Traumatic | 8.8 |
SCI 13 | M | 19 | C7 | B | 37 | 20 | Traumatic | 3.8 |
SCI 14 | M | 36 | C7 | C | 53 | 24 | Traumatic | 4.0 |
SCI 15 | F | 22 | C8 | B | 50 | 66 | Traumatic | 19.9 |
SCI 16 | M | 71 | T1 | C | 58 | 59 | Non-traumatic | 5.3 |
SCI 17 | F | 46 | T1 | D | 89 | 92 | Non-traumatic | 1.8 |
SCI 18 | F | 58 | T4 | D | 96 | 78 | Non-traumatic | 2.1 |
SCI 19 | F | 49 | T6 | A | 50 | 32 | Non-traumatic | 2.4 |
SCI 20 | F | 41 | T9 | A | 55 | 28 | Traumatic | 9.8 |
SCI 21 | M | 58 | T9 | C | 86 | 77 | Non-traumatic | 3.1 |
SCI 22 | M | 58 | T10 | A | 50 | 48 | Traumatic | 4.1 |
SCI 23 | M | 33 | T10 | A | 50 | 58 | Non-traumatic | 0.8 |
SCI 24 | M | 35 | T12 | A | 50 | 16 | Traumatic | 3.3 |
The study was approved by the Ethics Committee of the Guttmann Institute and was conducted in accordance with the Declaration of Helsinki. All participants gave written informed consent prior to enrollment.
2.2. Experimental setup and protocol
Participants were instructed to perform a simple reaction time task to reach a switch on the wall (figure 1). In the initial position, subjects were sitting in a wheelchair, with the hip flexed at 90°, the knees flexed at 90°, the arms resting on their legs or the armrests, and the soles of the feet on the floor or on the footrests, which were adjusted so that the knees were at the level of the pelvis. The target switch button was placed in front of them, aligned with the subject's midline, at a distance of 15 cm of the index fingertip (or the fist, in SCI subjects with limited finger mobility) with the arm extended.
Figure 1. Schematic representation of the subject's task. In response to the IS, the subject flexes the trunk forward to reach the switch button. A smartphone is placed at the subject's chest to record accelerometer data and derive the trunk tilt and lateral angles. The orientation of the x (brown), y (red), and z (blue) axes is shown, as well as the ZY plane, where the tilt angle (θ) is calculated, and the XY plane, where the lateral angle (ϕ) is calculated.
Download figure:
Standard image High-resolution imageIn response to the IS, the participants had to raise their arm and flex the trunk forward to reach the button as fast as possible but trying not to lose balance. The IS was a low-intensity electrical signal (3.6 mA, 0.2 ms duration) applied to the right little finger. In SCI subjects with impaired sensory perception in the hand, the IS was delivered on the right shoulder. Subjects were forewarned to be prepared a few seconds before the IS. Participants performed 20 trials, in 25% of which a SAS was presented simultaneously with the IS. The SAS was obtained by discharging a magnetic coil on top of a metallic platform, reaching a sound intensity of 125 dB for 250 ms [42]. During all the test, participants were closely supervised by two assistants to ensure optimal safety.
2.3. Data collection
Electromyographic (EMG) data were collected from eight neck, shoulder, and trunk muscles on the left side of the body: sternocleidomastoid (SCM), middle deltoid (DEL), trapezius (TRA), pectoralis major (PECT), upper abdominal Th6 (ABD), and paraspinal muscles at cervical C3 (PC), thoracic T6 (PT), and lumbar L2 (PL) levels. When both upper limbs were similarly impaired, we asked the patients to use their left hand to press the button, as we did with healthy subjects. If the left hand was more affected, the patient reached with the right hand. This was the case of the first two patients, so EMG data was recorded from the right side. EMG signals were recorded at a sampling rate of 10 kHz by means of a ten-channel EMG system (Synergy, VIASYS Healthcare UK Ltd, 2005), using disposable adhesive surface electrodes (outer diameter 20 mm; Technomed) that were attached over the muscle belly. The remaining two channels of the EMG system were used to record (a) the activity of the orbicularis oculi muscle (OOc) and thus measure the blink reflex, and (b) the electrical artifact generated when pressing the wired switch button. Each trial was recorded for 3 s, starting 600 ms prior to the IS to evaluate the basal activity.
In addition, a smartphone (Samsung Galaxy S5) was placed on the subjects' chest, over the sternum, using an elastic band, to collect triaxial accelerometer data for motion analysis with the smartphone built-in sensor (MPU-6500 three-axis MEMS accelerometer with 16-bits analog-to-digital converter ADC), TDK InvenSense, San Jose, CA, United States). As depicted in figure 1, the smartphone accelerometer x-axis was in the transverse (left-to-right) direction, the y-axis in the longitudinal (superior-to-inferior) direction, and the z-axis in the anteroposterior direction. Accelerometer data were sampled at 200 Hz and stored as a text file.
2.4. Data processing and analysis
The time to complete the task was calculated as the time from the IS to the button press and will be referred to as the RespT.
A band pass filter between 40 and 500 Hz was applied to the EMG signals to remove baseline oscillations and high-frequency noise, followed by a Notch filter (50 Hz and harmonics) for power-line interference cancellation. EMG traces contaminated by artifacts were removed by visual inspection.
EMG onset latencies were calculated for each muscle and trial, by using an automatic custom-made algorithm [43] based on the highest ratios of consecutive peaks of the Teager–Kaiser energy operator [44, 45]. Onsets were first determined by the computer algorithm, and then visually approved and corrected when necessary [25].
To calculate the EMG envelopes, EMG signals were full-wave rectified and low-pass filtered at 20 Hz. The baseline activity was calculated over the 600 ms prior to the IS and subtracted from all signals. Then, for each muscle and trial, the mean absolute value (MAV) of the EMG envelope from the onset of the muscle to the RespT was calculated, as a measure of the EMG amplitude.
EMG and smartphone data were manually synchronized offline, based on the time stamps in the extracted text files. The smartphone accelerometer data were used to monitor the trunk tilt and lateral angle signals, which are the angles calculated in the ZY plane, and the XY plane, respectively (figure 1). These angles were estimated based on the projection of the gravity acceleration on the axes of the accelerometer. As the trunk flexion movement is mainly rotational, linear acceleration can be neglected, and the tilt (θ) and lateral (φ) angles with respect to the longitudinal axis of the body can be calculated at each time point as:
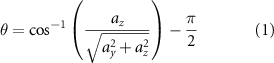
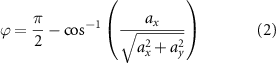
being ax, ay , and az the acceleration values of the x, y, and z axes at each time point (in m s−2). Defined this way, the angles are expressed in radians, and positive tilt angles (θ > 0) correspond to forward tilt, while positive lateral angles (ϕ > 0) indicate a lateral deviation to the right.
The angle signals were smoothed by a low pass filter of 2 Hz, and then movement features were extracted. To measure the trunk lateral deviation from the central sacral line, we calculated the maximum absolute value of the lateral angle signal. From the tilt angle signal, we calculated the maximum trunk inclination angle, the duration and angular velocity of the forward movement (from the beginning of the movement to the time of the maximum tilt angle), and the maximum peak-to-peak distance in the first 200 ms, a feature related to movement initiation proposed in a previous work [20] that we will refer to as PeakAng. Data processing and analysis was performed with custom written Matlab code (r2018a, Mathworks Inc.).
2.5. Statistical analysis
For each trial with a SAS, the OOc EMG activity was examined for the presence of a SAS-elicited blink reflex, identified as a short EMG burst larger than 50 μV at a latency of 40–50 ms. Differences between groups in the rate of occurrence of blink reflexes in SAS trials were tested using a chi-square test.
For each feature, data were averaged across trials to obtain a single measure for each subject and condition (non-SAS vs SAS). Then, descriptive statistics, i.e. means and standard deviations (SDs), were calculated for each group (cSCI, tSCI, and control).
Normality was assessed using the Shapiro–Wilk test. Since the assumptions for parametric analyses were not met, the outcome measures were tested using a robust two-way mixed analysis of variance (ANOVA) on the 20% trimmed means (bwtrim function, WRS2 R package [46]) with Condition (non-SAS vs SAS) as a within-subjects factor and group (cSCI, tSCI, and control) as a between-subjects factor. Trimmed means are a robust measure of central tendency, especially in asymmetric distributions, since they reduce the influence of outliers or data points on the tails by removing a percentage of the largest and smallest values before calculating the average. The robust ANOVA was run on the 20% trimmed means, because this achieves nearly the same amount of power as the mean when sampling from a normal distribution and, when there are outliers, a 20% trimmed mean can have a substantially smaller standard error [46].
When significant effects in the robust ANOVA were found, Wilcoxon signed-rank tests were conducted to compare non-SAS and SAS trials in each group, while multiple pairwise Mann–Whitney U tests were performed to determine differences between groups. In addition to the p-values, the estimated difference in medians and the 95% confidence intervals (CIs) are reported.
Finally, the correlations of each feature in the baseline condition (non-SAS) with the total AIS motor score, the spinal cord independence measure (SCIM), and the injury level were analyzed with Spearman's rank correlation coefficient. Statistical analyses were performed using R (version 3.6.2; www.r-project.org). The alpha level was set at 0.05 for all comparisons.
3. Results
All subjects were able to raise the arm and flex the trunk forward and succeeded in reaching the target. The RespT and EMG signals from the eight muscles described in '2.3. Data Collection' were analyzed for all subjects. For technical reasons, in one of the cSCI patients (SCI 15), the channels corresponding to the PECT and the RespT could not be recorded. Therefore, for this patient, we had no measures of the EMG amplitudes (as they were calculated from the EMG onset to RespT). On the other hand, in one of the patients with tSCI (SCI 20), data from SAS trials could not be collected, because the patient was too scared of the SAS and could not react. Artifact-contaminated EMG data were discarded, including all data of ABD and PT from one of the patients with tSCI (SCI 23), data of ABD from one of the control subjects, and data of PC from another one. Patients with SCI showed EMG activity in all the recorded muscles, except for some patients with cSCI: the PECT in SCI 6, and the ABD in SCI 5 and SCI 7.
Table 2 summarizes all data, with the values of each feature across groups and conditions, as well as the main effects examined by the two-way mixed ANOVAs. As shown in table 2, the analysis of outcome measures revealed a significant effect of the group in RespT, movement duration, maximum trunk tilt angle, lateral deviation, angular velocity, EMG onset latencies of all muscles, and EMG amplitudes of SCM and TRA. A significant effect of the SAS was found in RespT, PeakAng, EMG onset latencies of all muscles, and EMG amplitudes of the PECT, and PC. A significant interaction between group and SAS was only found for the EMG MAV of PC (F = 5.2, p = 0.02). Below, we describe more in detail the effects of the group in the baseline condition (non-SAS), and then the effects of the SAS. Table 3 contains the results of the post-hoc tests, including the pairwise comparisons between groups (table 3(a)) and the comparison between non-SAS and SAS trials at each group (table 3(b)).
Table 2. Outcome measures in the control, cSCI, and tSCI groups, both in SAS and non-SAS trials. The first six columns show the mean (SD) for each condition, and the last four columns show the results of the statistical analysis, using a robust two-way mixed ANOVA design with group and SAS as factors.
Mean (SD) non-SAS | Mean (SD) SAS | p-values | F-statistic | |||||||
---|---|---|---|---|---|---|---|---|---|---|
Parameter | cSCI | tSCI | Control | cSCI | tSCI | Control | Group | SAS | Group | SAS |
Switch button | ||||||||||
RespT (ms) | 1100 (295) | 801 (136) | 671 (86) | 1015 (264) | 653 (143) | 587 (80) | 0.002 | <0.001 | 12.18 | 26.82 |
Accelerometry | ||||||||||
Duration (ms) | 1.02 (0.35) | 0.68 (0.1) | 0.54 (0.05) | 1.03 (0.33) | 0.64 (0.12) | 0.54 (0.06) | <0.001 | 0.39 | 18.29 | 0.84 |
Tilt angle (°) | 31 (8) | 18 (5) | 26 (5) | 33 (9) | 19 (7) | 27 (7) | 0.011 | 0.16 | 6.15 | 2.19 |
Lat. deviation (°) | 7.1 (4.8) | 4.5 (1.6) | 2.9 (1.3) | 6.9 (4.5) | 5.5 (2.2) | 2.9 (1.4) | 0.012 | 0.14 | 6.75 | 2.40 |
Velocity (°/s) | 33 (9) | 28 (8) | 50 (12) | 34 (9) | 31 (11) | 51 (16) | <0.001 | 0.051 | 13.97 | 4.28 |
PeakAng (°) | 10 (7) | 11 (12) | 12 (6) | 15 (12) | 21 (21) | 18 (9) | 0.37 | <0.001 | 1.04 | 27.97 |
EMG latencies (ms) | ||||||||||
SCM | 285 (157) | 206 (55) | 175 (43) | 245 (193) | 94 (34) | 112 (43) | 0.027 | <0.001 | 5.05 | 30.80 |
DEL | 423 (230) | 357 (158) | 213 (55) | 368 (265) | 253 (153) | 151 (39) | 0.031 | <0.001 | 4.96 | 35.12 |
TRA | 306 (139) | 213 (57) | 173 (50) | 228 (148) | 138 (52) | 115 (28) | 0.034 | <0.001 | 4.61 | 36.22 |
PECT | 285 (140) | 231 (72) | 166 (45) | 237 (194) | 151 (64) | 107 (28) | 0.012 | <0.001 | 6.69 | 41.53 |
ABD | 386 (97) | 269 (100) | 227 (70) | 307 (156) | 153 (81) | 160 (75) | <0.001 | <0.001 | 22.23 | 31.31 |
PC | 360 (133) | 246(58) | 225(60) | 265(141) | 133(46) | 145(56) | 0.028 | <0.001 | 4.87 | 78.32 |
PT | 425(108) | 264 (75) | 256 (68) | 326 (122) | 143 (78) | 168 (55) | 0.002 | <0.001 | 11.84 | 42.15 |
PL | 609 (173) | 414 (122) | 368 (78) | 508 (219) | 315 (140) | 278 (82) | 0.002 | <0.001 | 10.96 | 45.71 |
EMG MAV (μV) | ||||||||||
SCM | 101 (94) | 41 (41) | 34 (29) | 101 (86) | 53 (47) | 41 (35) | 0.019 | 0.14 | 5.84 | 2.52 |
DEL | 416 (317) | 321 (146) | 343 (178) | 425 (328) | 339 (196) | 372 (190) | 0.86 | 0.31 | 0.15 | 1.25 |
TRA | 268 (147) | 167 (102) | 106 (67) | 296 (177) | 184 (108) | 114 (74) | 0.006 | 0.056 | 7.78 | 4.35 |
PECT | 101 (119) | 77 (57) | 71 (56) | 112 (124) | 96 (63) | 85 (67) | 0.78 | <0.001 | 0.25 | 16.73 |
ABD | 32 (43) | 29 (42) | 30 (29) | 36 (55) | 34 (41) | 37 (38) | 0.79 | 0.79 | 0.08 | 0.24 |
PC | 46 (29) | 19 (9) | 27 (33) | 49 (33) | 27 (10) | 29 (31) | 0.2 | <0.001 | 2.61 | 16.42 |
PT | 92 (105) | 24 (12) | 42 (17) | 89 (109) | 32 (16) | 43 (22) | 0.067 | 0.47 | 3.61 | 0.55 |
PL | 38 (32) | 24 (16) | 35 (19) | 44 (50) | 30 (17) | 33 (16) | 0.76 | 0.24 | 0.28 | 1.51 |
Table 3. Estimated median differences, 95% confidence intervals (CIs), and statistical significance of the post-hoc tests comparing the three groups at each condition by pairwise Mann–Whitney U-tests with Bonferroni corrections (a), and comparing non-SAS and SAS trials at each group with paired samples Wilcoxon signed-rank tests (b). Only the features with significant main effects in the robust mixed ANOVA are shown.
(a) Group effect—estimated median difference (95% CI) and adjusted p-values | ||||||
---|---|---|---|---|---|---|
Non-SAS | SAS | |||||
Parameter | cSCI vs tSCI | cSCI vs Control | tSCI vs Control | cSCI vs tSCI | cSCI vs Control | tSCI vs Control |
Switch button | ||||||
RespT (ms) | −242 (−511, −83)c | −386 (−527, −259) a | −147 (−233, −59)c | −331 (−595, −133) b | −379 (−507, −272) a | −67 (−189, 53) |
Accelerometry | ||||||
Duration (ms) | −267 (−449, −99) b | −371 (−569, −279) a | −158 (−209, −99) b | −310 (−584, −126) b | −381 (−631, −257) a | −94 (−181, −18) |
Tilt angle (°) | −12 (−17, −7) a | −4 (−8, −1) | 8 (3, 12) b | −14 (−18, −5) b | −5 (−9, −1) | 8 (1, 13) |
Lat. deviation (°) | −2 (−6, 1) | −3 (−7, −1) b | −1.5 (−3, −0.4) c | −0.7 (−4, 2) | −3 (−6, −1) b | −3 (−4, −1) c |
Velocity (°/s) | −5 (−13, 2) | 14 (8, 21) a | 19 (13, 28) a | −3 (−13, 6) | 15 (7, 23) a | 17 (8, 29) b |
EMG latencies (ms) | ||||||
SCM | −34 (−126, 22) | −57 (−130, −21) b | −29 (−69, 7) | −86 (−244, −18) b | −73 (−141, −20) c | 15 (−15, 46) |
DEL | −32 (−277, 91) | −125 (−356, −44) a | −102 (−290, −39) c | −47 (−295, 76) | −136 (−350, −33) b | −67 (−161, 4) |
TRA | −76 (−179, 14) | −116 (−178, −48) b | −45 (−79, 6) | −43 (−198, 19) | −63 (−156, −9) c | −16 (−49, 15) |
PECT | −30 (−163, 56) | −90 (−195, −30) c | −62 (−110, −18) c | −44 (−148, 39) | −77 (−155, −21) c | −27 (−103, 3) |
ABD | −119 (−205, −30) | −159 (−210, −99) a | −39 (−108, 32) | −134 (−248, −32) c | −130 (−203, −58) b | 4 (−54, 69) |
PC | −114 (−201, −11) | −138 (−207, −55) b | −17 (−73, 28) | −117 (−227, −25) c | −96 (−182, −32) b | 11 (−27, 50) |
PT | −160 (−234, −72) a | −158 (−225, −102) a | −6 (−69, 54) | −172 (−279, −80) b | −163 (−223, −86) a | 30 (−34, 88) |
PL | −158 (−291, −62) c | −215 (−288, −138) a | −45 (−139, 44) | −167 (−323, −26) | −182 (−295, −97) a | −18 (−122, 61) |
EMG MAV (μV) | ||||||
SCM | −51 (−97, 1) | −62 (−92, −12) b | −0.7 (−17, 11) | −33 (−94, 10) | −42 (−86, −13) b | −5 (−34, 10) |
TRA | −90 (−198, 23) | −141 (−220, −78) a | −48 (−139, 7) | −95 (−231, 24) | −157 (−242, −83) a | −64 (−149, 10) |
Switch button | ||||||
RespT (ms) | 70 (−7, 166) | 140 (61, 224) b | 80 (64, 100) c | |||
Accelerometry | ||||||
PeakAng (°) | −4 (−8, −1) b | −8 (−18, −2) b | −5 (−9, −3) c | |||
EMG latencies (ms) | ||||||
SCM | 46 (−16, 102) | 119 (65, 175) b | 62 (44, 83) c | |||
DEL | 61 (24, 106) b | 121 (19, 225) b | 61 (38, 86) c | |||
TRA | 76 (24, 133) a | 68 (2, 136) a | 55 (38, 76) c | |||
PECT | 64 (−2, 96) | 80 (25, 140) a | 54 (42, 69) c | |||
ABD | 91 (5, 134) a | 105 (29, 225) a | 65 (39, 93) c | |||
PC | 89 (31, 145) b | 109 (44, 181) a | 76 (57, 103) c | |||
PT | 97 (53, 144) b | 117 (48, 202) a | 83 (61, 113) c | |||
PL | 103 (37, 160) b | 66 (11, 151) a | 93 (61, 119) c | |||
EMG MAV (μV) | ||||||
PECT | −6 (−14, 1) | −12 (−25, −1) a | −8 (−16, −5) c | |||
PC | −2 (−5, 0.5) | −7 (−10, −5) b | −2 (−3, −0.5) a |
3.1. Group effect
Figure 2 shows the RespT, and figure 3 the EMG onset latencies for all participants in non-SAS and SAS trials. Regarding the muscle activation pattern, it can be inferred from table 2 and figure 3 that, in average, for all the groups and conditions, the SCM, TRA, and PECT were the first muscles to be activated, and the paraspinal muscles were sequentially activated in a rostro-caudal order: first PC, then PT, and lastly PL. However, according to the group means, the DEL was remarkably delayed in both cSCI and tSCI patients, as compared to controls, being the second to last muscle to be activated in SCI patients.
Figure 2. RespT for each group, in non-SAS and SAS trials (a), and RespT of each SCI patient in non-SAS (circle) and SAS (square) condition, as a function of the injury level and severity (b).
Download figure:
Standard image High-resolution imageFigure 3. EMG onset latencies for all muscles in all the participants, in non-SAS and SAS trials. Dotted lines represent individual participant data, wide lines indicate the group mean values (cSCI in red, tSCI in blue, controls in black), and error bars indicate standard deviation. Asterisks and hashes indicate statistical differences between groups and between non-SAS and SAS trials, respectively (*p < 0.05, **p < 0.01, ***p < 0.001).
Download figure:
Standard image High-resolution imagePatients with cSCI were slower than patients with tSCI, who, in turn, were slower than controls (figures 2, 3 and tables 2, 3). In terms of RespT and movement duration, there were significant between-group differences in non-SAS trials (table 3(a)). In addition, all muscles were activated later in SCI patients than in controls. The mean group onset latency values were higher in cSCI than in tSCI patients, but significant differences in non-SAS trials were only detected for PT and PL (table 3(a)). The EMG latencies of all muscles were significantly longer in cSCI patients than in controls, with estimated differences ranging from 57 ms in the SCM to 215 ms in the PL (table 3(a)). Although for most muscles there were no significant differences in the EMG onset latencies between tSCI patients and controls, the onset latencies of DEL and PECT were significantly longer in tSCI patients in non-SAS trials (DEL: estimated delay in medians 102 ms, 95% CI 39–290 ms, p = 0.011, PECT: estimated delay 62 ms, 95% CI 18–110 ms, p = 0.048). Regarding EMG amplitudes, the activity of SCM and TRA significantly increased in cSCI patients compared to controls, while no differences were found between cSCI and tSCI patients, nor between tSCI patients and controls (table 3(a)).
The features extracted from the smartphone accelerometer are displayed as boxplots in figure 4, for all the groups and conditions. Patients with SCI (including both cervical and thoracic injuries) presented a significantly higher lateral deviation when performing the trunk movement than control subjects (figure 4 and table 3(a)). This lateral deviation was, in average, slightly higher in patients with cSCI (table 2), but this group also showed a much higher dispersion. On the other hand, the maximum trunk tilt angle was lower in tSCI than in cSCI and controls. For this reason, even though the movement duration was shorter in tSCI than in cSCI, there were no differences between these two groups in terms of angular velocity (p = 0.33 in non-SAS trials, p > 0.99 in SAS trials), and both showed significantly lower angular velocities than controls (figure 4 and table 3(a)).
Figure 4. Boxplots of the features extracted from accelerometer data per group (cSCI, tSCI, and control) and condition (non-SAS vs SAS). Asterisks indicate statistically significant differences between groups, in non-SAS (black) and SAS (red) trials, while hashes denote statistically significant differences between SAS and non-SAS trials at each group. Red crosses correspond to outliers.
Download figure:
Standard image High-resolution image3.2. SAS effect
SAS-induced blink reflexes were recorded in 93% of SAS trials in control subjects, 80% in patients with tSCI, and 86% in patients with cSCI, showing no significant between-group differences (χ2(2) = 5.39, p = 0.068).
While the RespT and all EMG onset latencies were noticeably reduced in SAS trials in practically all healthy control subjects and tSCI patients, this was not the case for the cSCI patients, who showed much more variability (figures 2 and 3). While reductions could be seen for some of the patients, others executed the movement even slower in the SAS than in the non-SAS trials, so the StartReact effect was not as consistently observed in cSCI as in the other two groups.
A post-hoc analysis comparing the non-SAS and SAS trials in each group (table 3(b)) confirmed that the SAS significantly reduced RespT and all EMG onset latencies in healthy control subjects and in tSCI patients. However, in cSCI patients, the SAS did not significantly shorten the RespT (p = 0.09) nor the onset latencies of SCM (p = 0.14) and PECT (p = 0.06) (table 3(b)). The latency shortening was more pronounced in tSCI patients than in controls, which completely normalized the RespT and the latencies that were longer in tSCI patients than controls in non-SAS condition, i.e. those of DEL and PECT. For instance, the estimated difference between the medians of RespT in non-SAS vs SAS trials was 80 ms for controls (95% CI: 64–100 ms, p ≪ 0.001), and 140 ms for tSCI (95% CI: 61–224 ms, p = 0.008). For this reason, the differences between tSCI patients and controls disappeared when SAS trials were compared (table 3(a)).
The EMG amplitude of PECT and PC significantly increased in SAS trials in tSCI patients and controls, but no significant differences were seen in cSCI patients (table 3(b)). On the other hand, the PeakAng significantly increased with SAS in all groups (all p < 0.008, table 3(b)).
3.3. Correlation with AIS motor score, SCIM, and injury level
Significant correlations with the AIS motor score were found for the RespT (r = −0.52, p = 0.011), movement duration (r = −0.59, p = 0.002), the EMG onset latencies of TRA (r = −0.54, p = 0.007), ABD (r = −0.71, p < 0.001), PC (r = −0.61, p = 0.002), PT (r = −0.44, p = 0.035), and PL (r = −0.63, p < 0.001), and the EMG amplitude of SCM (r = −0.59, p = 0.003), TRA (r = −0.47, p = 0.025), PC (r = −0.50, p = 0.016), and PT (r = −0.63, p = 0.002). These negative correlations indicate that patients with low motor scores tended to perform slower movements and to show abnormally increased EMG activity. The SCIM was significantly correlated with the RespT (r = −0.50, p = 0.015), movement duration (r = −0.60, p = 0.002), lateral deviation (r = −0.52, p = 0.009), and EMG onset latencies of PC (r = −0.47, p = 0.025) and PL (r = −0.49, p = 0.015).
Finally, data were significant correlated with the injury level. Patients with lower SCI level had shorter RespT (r = 0.73, p < 0.001), movement duration (r = 0.77, p < 0.001), and EMG onset latencies for TRA (r = 0.50, p = 0.013), ABD (r = 0.59, p = 0.004), PC (r = 0.55, p = 0.006), PT (r = 0.71, p < 0.001), and PL (r = 0.69, p < 0.001). There was also a significant correlation with the maximum trunk tilt angle, which was smaller in lower SCI levels (r = 0.73, p < 0.001).
4. Discussion
In this study, we have investigated trunk function and the StartReact effect in patients with cervical and thoracic SCI performing a reaching task that required trunk tilting. The extracted outcome measures, derived from EMG and smartphone accelerometer data, revealed differences in the postural control strategies employed by SCI patients, in both non-SAS and SAS trials, according to their SCI level. First, as indicated by the RespT and movement duration measured by the accelerometer, SCI patients, especially those with cSCI, were slower than control subjects. Moreover, the results demonstrate that EMG onset latencies were significantly delayed in all muscles in the cSCI group compared to controls, whereas EMG delays in the tSCI group were only seen in the prime movers, DEL and PECT (table 3(a)). Slower movements and delayed activity of trunk muscles are also common after stroke [47], and it has been suggested that this could also be considered a compensatory strategy employed by patients with reduced trunk control to decrease intrinsic perturbations and increase postural stability [2, 6]. Regarding the sequence of muscle activation, of note is the fact that the deltoid was largely delayed in SCI patients, being one of the last muscles to be activated in the movement (table 2 and figure 3). This means that SCI patients lifted the arm much later than controls, probably because of the muscle weakness and/or to avoid destabilization during movement initiation, which results in defects to coordinate the movements of the trunk and the upper limb. This loss of fine motor coordination reflects the difficulties faced by patients with impaired trunk function when performing basic ADLs such as reaching and highlights the role of trunk stability during limb movements.
Although the mean values for the EMG amplitudes were higher in cSCI than in tSCI patients and controls (table 2), significant differences were only found for the SCM and TRA between cSCI patients and controls (table 3(a)). Therefore, from our results we cannot infer that there is a globally increased trunk EMG activity in SCI patients. Even so, increased EMG amplitudes were especially evident in patients 1, 2, 5, 7, 8, 10, 12, and 13, most of whom sustained motor complete injuries (AIS A–B, table 1). These results could be considered consistent with the reported review of surface EMG as a measure of trunk muscle activity in SCI patients [15]. In such review, the authors documented that trunk muscle activities for the sitting condition were greater in SCI patients than healthy subjects, and greater for patients with high-level than low-level injuries. For example, Desroches et al [17] found greater muscle activity of PECT during forward trunk flexion, and Louis and Gorce [48] indicated that subjects with SCI had a higher muscle activation of PECT and TRA during wheelchair propulsion. Conversely, other studies, such as that by McKay et al [49], report that EMG amplitudes were lower after SCI. Nevertheless, due to the differences in the protocols, especially regarding the task and muscles recorded, it is difficult to establish a comparison. Moreover, we have to remark that the EMG activity was not recorded bilaterally because of the limited number of EMG channels, so we could not determine what happened in the contralateral side of the body. In our study, the increased EMG activity in SCM and TRA in cSCI patients might be considered as an involuntary or reflex activity that arises when the trunk flexion movement is performed. However, we believe that a more plausible explanation would be that, because of the damage to the cervical spinal cord, cSCI patients have less muscle capacity available for the task, so they need a higher voluntary activation of the less affected muscles (e.g. neck muscles such as the SCM and TRA, innervated by higher spinal segments) to compensate for trunk instability and regulate sitting balance, as suggested in [18].
Smartphone accelerometer data allowed us to study trunk movement simultaneously in two planes (XY and ZY, figure 1), and thereby to monitor the trunk tilt angle and lateral deviation at each time point. The tilt angle measures the amplitude of trunk flexion, while the lateral angle indicates to what extent the movement is straight. On the one hand, the maximum tilt angle was significantly smaller in patients with tSCI than patients with cSCI and controls (figure 4). This could mean that tSCI patients, who have a certain degree of impairment in trunk function, prefer to limit the trunk range of motion to avoid losing balance, which is not possible for cSCI patients with more severely impaired trunk control. Able-bodied controls have an intact trunk stability, so they can reach higher tilt angles without risk of losing balance. The observed differences could also be explained by the fact that tSCI patients have been trained to move in a wheelchair, but this is not the case for controls, who were seated for the first time in a wheelchair to participate in the experiment. On the other hand, a significant finding was that patients with SCI (both cSCI and tSCI) had greater difficulty than control subjects in moving straight to the target, since forward trunk tilt was accompanied by a significant lateral deviation (table 2 and figure 4). The between-group median differences were not too high (2°–3°, table 3(a)), because the maximum lateral angle varied between subjects, and not all the SCI patients showed increased lateral deviations (figure 4). However, very high lateral deviations were observed in some SCI patients, and more notably in those with cervical injuries, than in controls (range: 1°–17° in cSCI, 2°–7° in tSCI, and 1°–6° in controls). Specifically, six out of 15 cSCI patients deviated laterally of 10° of more. Lateral deviation could reflect the deficit in balance control and could be a measure of trunk instability. Furthermore, this lateral deviation can also be thought as a compensatory strategy to increase the base of support and improve sitting balance during the reaching task requiring trunk tilting. This would be consistent with previous studies that have reported similar compensatory strategies to increase sitting stability after SCI [7, 50]. In any case, monitoring the trunk lateral angle could help rehabilitation professionals to guide therapeutic interventions, either to correct these lateral deviations (to prevent long-term contractures and lesions) or to promote them as compensatory strategies that may improve functional independence. Finally, we measured the maximum peak-to-peak distance in the tilt angle signal at the beginning of the movement (PeakAng), a feature proposed in a previous study as a measure of the anticipatory movement preceding trunk flexion [20]. While the magnitude of this peak did not differ between groups, in all cases (cSCI, tSCI, and controls) it was significantly higher in SAS trials (table 3(b)), reflecting the involuntary body movement induced by an unexpected SAS.
One of the strengths of this work is the addition of a SAS paradigm. Indeed, the study presented here is the first investigating the StartReact effect in trunk muscles in SCI patients. Previous works in clinical populations such as HSP [32, 33], stroke [34–37], and Parkinson's disease [38, 39] found an intact StartReact effect, compatible with the preservation of the reticulospinal tract in those diseases [34]. We observed a significant shortening of EMG onset latencies in the majority of muscles tested (including the paraspinal muscles) in all groups. However, a significant shortening of RespT, i.e. a normal StartReact effect, was only observed in healthy controls and in tSCI patients. In fact, the StartReact effect was variable across the group of cSCI patients. While some cSCI individuals exhibited relatively normal responses, in others the SAS negatively impacted movement execution and, instead of accelerating the RespT, made it slower (i.e. there was an abnormal StartReact effect).
Patients with cSCI had longer RespT and EMG onset latencies than those with tSCI both in trials with and without SAS. Obviously, one reason for this is the defective activation of relevant muscles in patients with upper cervical lesions, which explains in part the correlation between RespT and injury level. However, this does not justify the reduction or absence of the StartReact effect in cSCI patients. This alteration could be explained by different hypothesis. One possible explanation would be the deficit in muscle activation in patients with high cSCI, which could prevent the rapid responses elicited by the SAS. Another plausible hypothesis is that patients with cSCI must exert increased voluntary control over the muscles still available for the task, in comparison to patients with SCI at lower levels, and this could have constrained the action of subcortical reflex circuits, thus limiting the expression of the StartReact effect. For individuals with appropriate trunk control, letting muscles react to a SAS has a minimal risk, since they will not lose balance. However, cSCI patients with impaired trunk stability have less muscle reserve and increased risk of losing balance during displacement, so they have to execute the movement more cautiously and require more precise control to avoid falling and potential damage. Unlike other reaction time tasks, such as upper limb or finger movements, the task presented here requires high postural control of body balance to avoid the risk of falling, so there should be a trade-off between the speed of trunk flexion movement, amenable to increase with the StartReact effect, and postural control, which should maintain the trunk flexion within certain limits to avoid destabilization. While the control of posture in healthy subjects may rely entirely on subcortical motor tracts, the need for a higher degree of control over the still available trunk muscles in cSCI patients may require a voluntary effort and continuous evaluation of the scarce afferent inputs informing on trunk and neck position. In these conditions, the StartReact effect may be less likely to occur because of the increased cortical control of the involved muscles, as it has been described during fine dexterous finger movements [40]. The hypothesis of an increased cortical control over the remaining muscles after cSCI receives support on several observations: (a) cortical functions have been found enhanced in individuals with impaired postural responses, as a form of compensation for defective automated processes of subcortical control [51]. (b) Neuroplasticity and cortical reorganization after SCI occurs to various extents over periods ranging from weeks to years, especially increasing the movement representations of intact body areas [52, 53]. Therefore, in the de-efferented motor cortex areas of patients with cSCI, the cortical representation of preserved trunk muscles might be enlarged and hyperactive. (c) Cortical plasticity is required to relearn previous motor patterns using alternative mechanisms, which helps rehabilitation to promote recovery of function [52].
The 24 individuals with SCI participating in the present study presented a wide range of neurological injury levels (C4–T12), degrees of completeness (AIS A–D), and times post-injury, as often seen in clinical practice, which may reinforce the reliability of our findings. However, the high heterogeneity of the sample can also be considered as one of the main limitations of the study since, given the relatively small sample size, we cannot extract robust conclusions about the effects of each of these sources of variability. In fact, the heterogeneity among spinal cord injuries is also one of the major challenges for the rehabilitation of trunk and overall motor function of these patients, and it has been demonstrated that both functional reach and maintenance of posture are associated with neurological level and SCI type [5]. In our sample, we found that AIS motor score, SCIM, and injury level were significantly correlated with the RespT (figure 2(b)), movement duration measured by the accelerometer, and some EMG onset latencies, indicating that, as expected, patients with higher injuries and more severe motor impairment tend to perform slower movements. In addition, the patients with low motor scores tended to show abnormally increased EMG amplitudes, especially in the neck and back muscles. Moreover, the lateral deviation was positively correlated with SCIM, suggesting that patients with lower functional independence showed larger trunk lateral deviations during the functional task. However, the correlation between lateral deviation and AIS motor score was not significant, and we believe the reason is that the SCIM test assesses various ADLs where trunk function is clearly involved, while the AIS motor score measures motor impairment of only the upper and lower extremities. All these issues should be explored in future studies with an increased sample size.
Our study has several other limitations. First, we examined trunk flexion in SCI patients in the anteroposterior direction, but it would be interesting to explore lateral trunk control as well, since it might be affected differently. The reason for selecting a simpler protocol was that it allowed us to study trunk function in patients with a wide range of severities, including complete cervical injuries with high levels of paralysis, who might be unable to execute complex multidirectional maneuvers. Moreover, the simplification of the test minimizes the time and effort required to acquire data, and thus facilitates its eventual implementation in clinical practice. On the other hand, we did not document the rehabilitation program of the participants, which is another source of variability that could introduce bias into the data, as the type and duration of rehabilitation, especially if related to trunk strengthening, may be a key factor for the recovery of postural control [15]. Future studies are needed to further explore the association between the proposed outcome measures and rehabilitation.
Improving trunk stability after SCI is a priority in the recovery process to optimize patients' functional independence and quality of life, as it is essential for ADLs. However, there is a lack of objective standardized measures for trunk function evaluation. A few quantitative tests using laboratory instrumentation have been proposed to assess seated postural control in SCI patients, such as those based on kinematic measures or COP displacement [4, 14, 17, 18], but most of them present challenges that compromise their application in the clinical setting, including the need for specialized equipment, time and cost expenses, and limited scalability. Here we have combined surface EMG with smartphone accelerometer data to evaluate trunk flexion in individuals with cervical and thoracic SCI. Surface EMG is a non-invasive neurophysiological tool that is widely used in the clinics to measure muscle contraction and evaluate neuromuscular function in SCI and other disorders of the nervous system. This technique has already been recommended in previous works as an interesting objective marker of trunk function [15]. In our study, EMG allowed us to measure the activity of trunk muscles and compare the neuromuscular patterns of patients with cSCI, tSCI, and control subjects. Further, we monitored the trunk tilt and lateral angles using a smartphone built-in accelerometer, as a novel approach for trunk movement analysis in a simpler and cost-effective way. Smartphones have shown great potential as mHealth tools for different clinical applications, including the analysis of body movements, due to their wide availability, range of embedded sensors, and powerful processing capabilities. This is a proof of concept of how smartphones can be used to record movement signals and extract quantitative measures that reflect postural control and trunk stability in patients with SCI.
5. Conclusion
The analysis and interpretation of EMG and smartphone accelerometer signals have allowed us to obtain movement and neurophysiological information to quantitatively evaluate trunk function and the StartReact effect during a reaching task in patients with SCI. The proposed outcome measures revealed motor changes and compensatory postural strategies employed by individuals with SCI, and especially those with cervical injuries, including delayed responses and higher lateral deviations, which evidence the compromised postural control and might have important consequences for rehabilitation. This information could contribute to the understanding of trunk motor function and sitting postural stability after SCI, which should be considered when developing personalized treatment programs to maximize the patients' functional recovery. Furthermore, in the future, these findings may help to develop more suitable methods to quantitatively assess trunk function in terms of muscle activity and movement patterns in individuals with SCI, which would improve the follow-up and management of these patients.
Acknowledgments
This work was developed in the framework of the joint project 'Biomedical signal interpretation to study motor impairment, neurological disorders and novel personalised neurorehabilitation therapies', between the Fundación Institut Guttmann and the Institute for Bioengineering of Catalonia. This work was supported in part by a fellowship from 'La Caixa' Foundation (ID 100010434) with fellowship code LCF/BQ/DE18/11670019, in part by the Secretaria d'Universitats i Recerca de la Generalitat de Catalunya under Grant GRC 2017 SGR 01770, in part by the Agencia Estatal de Investigación, the Spanish Ministry of Science, Innovation and Universities, and the European Regional Development Fund under Grant RTI2018 098472-B-I00, in part by the CERCA Program/Generalitat de Catalunya, in part by H2020-ERA-NET Neuron under Grant AC16/00034, in part by La Marató de TV3 2017 under Grant 201713.31, and in part by Premi Beca 'Mike Lane' 2019—Castellers de la Vila de Gràcia. The authors declare no competing interests.
Data availability statement
The data that support the findings of this study are available upon reasonable request from the authors.