Abstract
We describe measurements of thermodynamic temperature in the range 5 K to 24.5561 K (the triple point of neon) using single-pressure refractive-index gas thermometry (SPRIGT) with 4He. In the wake of the May 2019 re-definition of the kelvin and its associated mise en pratique, the main purpose of the work is to provide values of T–T90, the discrepancy between thermodynamic temperature and that of the International Temperature Scale of 1990 (ITS-90). The link to ITS-90 is made via calibrated rhodium-iron resistance thermometers. Innovations required to reach the level of accuracy required for meaningful measurements (uncertainty in T–T90 less than the expected deviation) include the suppression of temperature oscillations in a cryogen-free cryostat, a pressure stabilization scheme based on a non-rotating piston balance, modelling of the hydrostatic head correction and refinements of the measurement of microwave resonances in a quasi-spherical copper resonator. The accuracy of measurements varies from 0.05 mK to 0.17 mK and is competitive with that of all previous ones in this temperature range using other techniques. The improvement stems partly from the new techniques used for the new definition of the kelvin as well as ab initio calculations of the thermophysical properties ofgaseous 4He. In addition to confirming the validity of SPRIGT as an accurate, easier-to-implement alternative to other low-temperature primary thermometry techniques (e.g. acoustic gas thermometry) yet with scope for improvement, the results should provide important input data for any future revision of ITS-90.
Export citation and abstract BibTeX RIS

Original content from this work may be used under the terms of the Creative Commons Attribution 4.0 license. Any further distribution of this work must maintain attribution to the author(s) and the title of the work, journal citation and DOI.
1. Introduction
This article describes work to measure absolute temperature in the range 5 K (a little above the boiling point of liquid 4He) to 24.5561 K (the triple point of neon). The purpose is twofold: to demonstrate the potential of single-pressure refractive-index gas thermometry (SPRIGT) while using it to improve the thermodynamic temperature scale in this region. In this section we review the present situation of low temperature scales to show why such measurements are desirable. This is followed by a description of the principle of SPRIGT which has been developed in an ongoing collaboration between the Technical Institute of Physics and Chemistry of Chinese Academy of Sciences (TIPC-CAS) in China and the Laboratoire National de Métrologie et d'Essais-Conservatoire National des Arts et Métiers (LNE-Cnam) in France [1].
1.1. Overview
Prior to the change of definition of the SI unit, the kelvin, temperature was defined via a set of two temperature scales, one of which, the International Temperature Scale of 1990 (ITS-90) [2] covers all temperatures above 0.65 K. (The other, the Provisional Low Temperature Scale of 2000, relates to temperatures from 0.902 mK to 1 K [3]). ITS-90 provides an approximation to thermodynamic temperature, through defining instruments (especially resistance thermometers) and a set of interpolation equations (polynomials of resistance ratios versus temperature). Ever since the adoption of ITS-90 in 1990, research has been carried out to quantify the discrepancy between the thermodynamic temperature T and the approximation T90 provided by the scale. Such an enterprise is challenging. Indeed, it was not until 2010 that the first set of equations to estimate T–T90 [4] was officially made available by the Consultative Committee for Thermometry (CCT) and was introduced in the mise en pratique of the kelvin (MeP-K). These equations allow one to calculate T from T90 measurements, albeit with an uncertainty that can be as large as 2 mK for temperatures below 273 K. For future revisions of the MeP-K, to improve the interpolating polynomials and thereby reduce the uncertainties associated with the correction, CCT recommends additional experimental determinations of T–T90 be performed. This task is far from trivial: two European Joint Research Projects have already been conducted on the determination of T–T90 (InK [5] and InK2 [6]) and further studies are anticipated in the coming years (for example, Real-K [7]).
The results presented in this paper are dealt with a set of measurements of T–T90 between 5 K and 24.5561 K, to contribute to the improvement of the MeP-K. In this temperature range, T90 can be realized in two different ways: either with a constant-volume gas thermometer (CVGT) specifically designed for this range, or else with a capsule standard platinum resistance thermometer (CSPRT) calibrated at specified sets of defined temperature fixed points, usable between 13 K and 24.5561 K. At temperatures below 13 K, the tiny resistance of CSPRTs compared with that of the measurement cables (<30 mΩ versus ≈ 30 Ω) means they are rarely used. Constant-volume gas thermometry is rather complex and time-consuming, so much so that NMIs equipped with a CVGT usually prefer to calibrate new thermometers by comparison with previously calibrated ones, to avoid running new CVGT experiments. The last international comparison on the realization of the ITS-90 between 0.65 K and 24.5561 K, CCT-K1 [8], took place between 1997 and 2001, with results being published in 2006. In this comparison, 'Only two participants ... submitted calibrations based on direct realizations of the ITS-90 using an interpolating constant-volume gas thermometer. Four other participants ... based their submissions on maintained gas thermometer scales'. Fixed-point calibrations with CSPRTs are far more current, several NMIs regularly using their facilities to implement ITS-90 from 13 K upwards. Thanks to the availability of sealed triple-point cells [9], the implementation of the ITS-90 above 13 K is somewhat easier than with CVGT. Even so, the realization of the scale includes two vapour pressure points of hydrogen at temperatures close to 17.035 K and 20.27 K that are difficult to realize, requiring a CVGT or open vapour pressure hydrogen cells. As with CVGT, laboratories prefer to realize these two fixed points by comparison with previously calibrated thermometers. The most recent international comparison on the realization of ITS-90 between 13 K and 273 K CCT-K2 took place between 1997 and 1999 with results published in 2002 [10]. (A few subsequent bilateral comparisons have not altered the situation). Even there, only two of the seven laboratories were able to provide at least one thermometer calibrated at 17.035 K and 20.27 K according to the ITS-90 prescriptions. Two more used other methods while the remaining three supplied no calibration at these temperatures whatsoever.
The complexity of the realization of ITS-90 between 5 K and 13 K, and at 17 K and 20 K, shows just how beneficial the new definition of the kelvin and its mise en pratique are. They allow the unit to be materialized by methods other than those prescribed in the ITS-90, often with a gain in simplicity and accuracy. One of these is SPRIGT.
With respect to the main aim of this paper, namely the measurement of T–T90 between 5 K and 24.5561 K, it was still possible, though not simple, to find a set of thermometers providing traceability to T90 at the best accuracy level between 5 K and 24.5561 K.
1.2. Principle of SPRIGT
The working principle of SPRIGT, detailed in Gao et al [1], is derived from the Lorentz—Lorenz equation providing, for a gas, a relationship linking thermodynamic temperature T, refractive-index n, pressure p, polarizability A
, and the universal gas constant R:

In the new SI the values of the Avogadro constant NA and the Boltzmann constant k are fixed, hence so is that of R= NA·k.
In SPRIGT, an unknown thermodynamic temperature T is determined from the ratio of gas refractive-indices n measured at both the unknown temperature T and at a known reference temperature Tref. The ratio is therefore:

where Tref is determined by another thermodynamic temperature measurement method. In the present work, Acoustic Gas Thermometry (AGT) [11, 12] was employed for Tref and a transfer standard (a standard platinum-cobalt resistance thermometer) used to transfer Tref value to the SPRIGT apparatus.
The refractive-index is obtained from the ratio of microwave resonance frequencies in the resonator under vacuum f(T,0) and filled with gas at a pressure p, f(T,p):

where f is the resonance frequency and kT (T) is the isothermal compressibility of the resonator at temperature T.
Equations (2) and (3) provide only approximate relationships. Analytical solutions for T are then far too complex to be useful. Instead, T is determined by numerical iteration, by minimizing the difference between experimental and calculated values of refractive-indices, hereafter referred to as nexp and ncalc respectively.
The refractive-index n is a function of the relative electric permittivity r and the relative magnetic permeability μr of the gas [13, 14]:

The former r is related to the molar gas density ρ via the Clausius-Mossotti equation:

where A, B
and C
are dielectric virial coefficients which, in the case of 4He, have been accurately determined by ab initio calculation (cf. those values in table 2 of Rourke [15], i.e.: A
, [16]; B
, [17]; C
, [18–22]).
The relative magnetic permeability μr is obtained from the analogous Clausius-Mossotti equation for magnetism:

where only the first term is required for the level of accuracy sought after here.
To calculate a value for the refractive index, we combine equations (4)–(6), to obtain:

where the molar density is related to the pressure p and temperature T, via:

Here B, C and D are the second, third and fourth density virial coefficients respectively.
For SPRIGT between 5 K and 24.5561 K, the thermometric gas is 4He, helium being the only species possible. With the development of ab initio calculations, the uncertainties in 4He properties, especially those of the density virial coefficients, have been reduced by nearly two orders of magnitude over the last two decades [23, 24], and there is still room for improvement.
The refractive-index given by equation (7) can be estimated numerically with an uncertainty lower than 10–11 at all temperatures in the pressure range 30 kPa to 120 kPa. From a knowledge of n2 and p, the value of the temperature T is extracted.
In SPRIGT, the refractive index nexp is determined experimentally using the following equation:
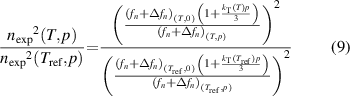
where fn is the measured frequency of a given mode and Δfn is a correction term discussed in Gao et al [1]. High-accuracy microwave resonance-frequency measurements with an uncertainty of 2 parts in 109 are possible (coverage factor k= 1), as has been successfully demonstrated in a very recent determination of the Boltzmann constant [25]. Note that in the present article all uncertainties are the k = 1 standard uncertainty unless otherwise indicated.
Equation (9) requires that resonance frequency measurements be performed at both T and Tref, first under vacuum, to determine and
, then at a pressure p to determine the other terms. To minimize measurement uncertainties, it is preferable to perform measurements on single isobars, i.e. with the same pressure at both T and Tref. In this way, first-order pressure terms cancel except for a residual term proportional to the variation of isothermal compressibility kT between Tref and T. The uncertainty in the temperature dependence of kT is calculated using the data published in Simon et al [26]. The pressure can be maintained stable to better than 4 parts in 106 with an appropriate pressure-control system as in Pitre et al [25].
Once nexp measurements have been completed, T is determined as the value of ϑ that minimizes the difference , calculated with the Newton-Raphson iteration method, wherein the present work a 10–10 K convergence criterion is imposed.
At the outset of this work, considering all the aforementioned points, a standard uncertainty of 0.25 mK was expected for SPRIGT in the temperature range 5 K to 24.5561 K. Furthermore, the measurement speed of SPRIGT is ten times faster than that of other primary thermometry methods, thanks to the use of ratios, rather than direct temperature measurements. Given the progress in the ab initio calculation of the properties of 4He, full isotherms are no longer required in principle in AGT or RIGT experiments. For these reasons, we consider that SPRIGT has strong potential for temperature measurements in the range 5 K to 24.5561 K.
The rest of the paper is structured as follows. In section 2 we describe the apparatus and experimental procedures. The resistance thermometry for T90 and Tref is described in section 3. Section 4 deals with the microwave measurements, while in section 5 we present the uncertainty budget and the results for T–T90 in the range 5 K to 24.5561 K. A conclusion and perspectives in section 6 round off the paper.
2. Apparatus and procedures
2.1. Cryostat
For stable long-term operation at low temperatures, a cryogen-free cryostat cooled by a pulse tube cryocooler (Sumitomo model RP-082B2) was used. The cryostat (shown in figure 1) was designed to house the microwave resonator and maintain its temperature in the range 5 K to 24.5561 K. The most challenging specification was to obtain a configuration, at the bottom near the resonator, where the temperature oscillation generated by the pulse tube (typically 1.72 Hz) was not detected by any of the thermometers installed in the environment near the resonator. The use of fast multimeter to measure the resistance thermometer (integration time less than 0.1 s ) and an Allan variance analysis were the main tools used to optimize performance [27].
Figure 1. Cross-sectional view of the cryostat.
Download figure:
Standard image High-resolution imageThe cryogen-free cryostat used here has been described in detail in an earlier paper [28] while the optimization of thermal parameters is the subject of a separate publication [29]. Here we shall not describe its structure but rather give a brief summary of the research performed using it. Radiation shields and multi-layer insulation materials were used to reduce the radiation heat loss. Thanks to the high vacuum in the cryostat, around 2 × 10−5 Pa, convection is negligible. In addition, a custom-made thermal link (estimated thermal resistance 3.3 K⋅W−1 [29]) and a gas-type heat-switch (using 4He at around 10 Pa [29]) were used to damp the temperature oscillation from the cryocooler. For thermal regulation, we developed a novel active control temperature oscillation method [30, 31] to stabilize the temperature at the microkelvin level from 5 K to 25 K. In addition, to reduce the heat loss from the pressure tube and microwave cable, heat-sinks were used wherever these components passed through a flange. In this way, the main element, the microwave resonator, can be cooled to as low as 4.3 K with no apparent temperature oscillation (for SPRIGT, measurements were all made above 5 K to avoid pre-condensation effects [32]). The resonator temperature stability can be maintained to within better than 8 µK of its set point (anywhere between 5 K and 25 K) over at least 180 h [27].
2.2. Microwave measurement
2.2.1. Resonator.
In order to perform SPRIGT at the 0.1 mK level, because of the small change of the values of the gas refractive-index for different pressures compared with those under vacuum, one needs to be able to measure the relative change of refractive-index with a resolution of the order of 10–9 [1]. To do this, we use a microwave cavity. Based on previous experience [25, 33], we have chosen to use a quasi-spherical copper cavity [34]. Copper is used because of its high electrical conductivity and physical properties at low temperature. High electrical conductivity enables a high signal-to-noise ratio frequency measurement and reduces the skin effect. In addition, the small heat capacity of copper favours rapid heat transfer and thereby realizes a uniform temperature distribution. The small linear expansion coefficient reduces the thermal effect on the frequency measurement under vacuum.
A spherical shape has an intrinsic symmetry, moreover, the quality factor of electromagnetic resonances is the highest of any shape. In a perfect sphere, the first electromagnetic resonances are three-fold degenerate. Since it is impossible to produce such a shape, however, uncontrolled departures from a perfect sphere would yield a distorted line shape. To lift the degeneracy of the microwave resonances, instead we use a tri-axial ellipsoid whose three resonances for the modes TM1j and TE1j (j ≥ 1) are clearly resolved. This improves the frequency resonance resolution by a factor of 1000 [35] compared with a perfect sphere [36].
The experimental work described here was carried out in a laboratory of TIPC-CAS in Langfang, China. To perform SPRIGT, a new quasi-spherical resonator (QSR) was built. It consists of two hemispheres of nominal shell thickness of 10.0 mm. The dimensions of the inner surfaces machined by precision diamond turning are the same dimensions as the of the resonator used at LNE-Cnam for measurements of the Boltzmann constant [37]. The inner shape of the resonator was designed to be a tri-axial ellipsoid which is defined by:

with a = 49.50 mm, 1 = 0.001, and
2 = 0.0005.
The resonator was closed successfully by monitoring the change of the relative excess half-width of three resonances at room temperature [38, 39]. Once the excess half-width for the z-axis has no obvious decrease when the resonator bolts are tightened, we assume there is no longer any shape change with increasing of torque, at which point we consider the resonator to be completely and correctly closed.
2.2.2. Cables.
The measurement of the resonance of the quasi-sphere [35] requires three elements: the resonator, a network analyzer and cables. A network analyzer is used to obtain the frequency response of the cavity as a complex number with in-phase and quadrature components. The microwave antennas are connected to the network analyzer via four pairs of coaxial cables connected by three pairs of feedthroughs (see table 1 and figure 2). No single type of cable or feedthrough works well enough at both room temperature and in the cryogenic regime. All cables are 2.4 mm in diameter, a compromise to minimize the signal attenuation (small diameter) cable and unwanted heat conduction (large diameter). The feedthroughs work down to low temperature (4 K) and are leak tight even if 200 kPa is applied on one side. To reduce cross-talk and maximize the signal-to-noise ratio, microwave cables and feedthroughs were selected according to the amplitude of the triple peaks by measuring the S21 scattering parameters at room temperature.
Figure 2. Distribution of cables and feedthroughs used in the present work.
Download figure:
Standard image High-resolution imageTable 1. Information on cables and feedthroughs used in the present work.
Cables | Type | Serial No. | Number × Length |
---|---|---|---|
C1 | MICRO-COAX UTIFLEX 205 series 503796-V | 00000042-49 00000038 − 43 | 2 × 2 m |
C2 | REBES-UT-085C-LL-SMAMSMAM | 1712152, 1712153, 1712154, 1712155 | 4 × 1 m |
C3 | REBES | ULT-05-SMA(m) SMA(m)–2000 | 2 × 2 m |
C4 | ROHS-RG405-AL-TP | 064579 | 2 × 1 m |
Feedthroughs | Type | Serial No. | Flange type |
F1, F2 | ALLECTRA: 242-SMAD 18G-C40-2 | WO5576 | CF40 |
F3, F4 | ALLECTRA: 242-SMAD 18G-C16 | WO5368 | CF16 |
2.2.3. Measurement of the resonance frequencies.
To measure the scattering parameters S21, a two-port vector network analyzer (Keysight Technologies N5241A PNA-X) was connected to two loop antennas, one in each hemisphere, the far ends of which are both flush with the inner surface of the resonator, used for signal emission and reception. Loop antennas are preferred to pin antennas since they allow excitation and reception of both TE and TM modes microwave signal [35]. A rubidium frequency standard (Stanford Research Systems FS725) was used to provide a 10 MHz reference signal to the vector network analyzer. For each microwave mode, frequencies are scanned over about 3 MHz for measurements at room temperatures and 1.5 MHz for those at low temperatures so as to cover the whole triplets. Each scan consists of a total of 144 points. The scanning pattern around the peaks is chosen for optimal determination of the resonance line shape and off-resonant background. Frequencies close to line centres are unequally spaced, so as to produce a regular angular separation in the (fn, gn ) polar diagram of the triplet. Between two peaks, equally spaced frequencies are used. Such a pattern is referred to here as a mixed scan (figure 3). To determine the optimal balance between on-peak and background-type scans, we used the matching ratio rm defined as the fraction of uniformly spaced points Nuniform compared with the total number, i.e. rm = Nuniform / Ntotal. It was optimized from microwave measurements under vacuum at 5 K, the optimum value being roughly 0.25 [40]. Once the scans have been completed, microwave resonance frequencies fn and half-widths gn are determined via non-linear least-squares fitting of the measured complex scattering parameters S21 using the following function [35]:

Figure 3. Modulus of the complex scattering amplitude parameter S21 for three different scanning patterns. When equally spaced frequencies are used, the background is well determined at the expense of too few points around resonance frequencies. With equally spaced phases, the converse is true. The mixed scanning pattern provides the best of both worlds.
Download figure:
Standard image High-resolution imagewhere f is the source frequency and the indices n = 1, 2 and 3 correspond to the three non-degenerate modes. The fitted parameters are the complex constants
An, B, C
and
D
, the centre frequencies of each resonance fn
and the corresponding half-width gn
of the resonance at half-maximum. The variable is an arbitrary frequency, here set equal to fn
.
The optimal microwave emission power, PMW = –10 dBm, was deduced from an Allan variance analysis of the temperature of the resonator at 5 K under vacuum for three different powers: 0 dBm, −7 dBm and −10 dBm. From figure 4, it is clear that for PMW = 0 dBm, the initial power used, the temperature displays a large oscillation with a period of 600 s, equal to the scanning time over the microwave modes TM11-TE11-TM12-TE13. This implies the microwave power was too great, so thermal stability was studied at lower powers of −7 dBm and −10 dBm. One can see a microwave power of –10 dBm has a negligible effect on the stability of the resonator temperature (see figures 5 and 6). All subsequent microwave measurements were carried out using this power.
Figure 4. Microwave self-heating effect with microwave emission power PMW = 0 dBm. Every 600 s, there appears a peak in the resonator temperature, corresponding to a less density filled area in the upper part of the figure.
Download figure:
Standard image High-resolution imageFigure 5. Allan deviation of the resonator temperature for a microwave power PMW = 0 dBm. The thermal oscillations with a period of 600 s (equal to the scanning period) are indicative of self-heating.
Download figure:
Standard image High-resolution imageFigure 6. Allan deviation of the resonator temperature for microwave powers −7 dBm and –10 dBm.
Download figure:
Standard image High-resolution image2.3. Gas handling and pressure measurement
2.3.1. Gas handling system.
For accurate SPRIGT, the gas composition must remain identical throughout the measurement. To ensure this, high purity 4He gas is used with highly clean pipelines. Moreover, any residual impurities present in the bottle are removed when the gas is used at temperatures below 25 K. To confirm this, a specific experiment was carried out by adding a cold trap at 4.2 K (see figure 7).
Figure 7. Schematic diagram of the gas handling system with two source bottles used in parallel, cold trap and pressure control system based on a piston balance.
Download figure:
Standard image High-resolution imageTo provide uninterrupted operation, the high purity (>99.9999%) 4He gas was sourced at high pressure (20 MPa) from two cylinders used in parallel (Air Liquide No 1151147 and No 1261172). Table 2 shows the gas analysis report supplied by Air Liquide for both. At temperatures below 25 K, where all the present measurements were performed, all impurities except hydrogen and neon are liquified or condensed. In order to verify that all impurities are indeed removed to an imperceptible level, the working gas was passed through a liquid helium cold trap at 4.2 K by closing valve V2 and opening valve V3 and V4. The trap removes the molecules O2, H2O, CO2, CO, H2, N2 and hydrocarbons such as CH4 to a level of <0.1 parts per billion (ppb). Heavier noble gas impurities (Ar, Kr, Xe) are also removed from the source gas and typically not listed in manufacturers' gas analysis reports. Between each measurement, the entire system was evacuated and replenished with fresh gas.
To avoid the build-up of impurities, a flowing gas system is used. The gas flows from the source bottles to pumps through the pressure regulator, cold trap, ballast volumes, mass flow controllers (MFC) and absolute pressure gauge. The gas-handling system is evacuated using a dry turbomolecular pump. Prior to any experiments, the system is flushed with helium at least five times.
A comparison of resonant frequencies with and without cold trap is shown in figure 8. The relative frequency shift (0.5 ppb ± 0.5 ppb), compatible with zero, confirms the assumption that the gas quality is indistinguishable in both cases, as in Pitre et al [25] and Purer et al [41]. The good agreement with and without cold trap proves that the concentrations of hydrogen and neon can be safely neglected. Given this, no cold trap was deemed necessary for the remainder of the work described here.
Figure 8. Comparison of the relative frequency shift in parts per billion (10–9) of four microwave modes between with a cold trap (twice) and without one (once), T = 24.5 K and p = 60 kPa.
Download figure:
Standard image High-resolution imageTable 2. Gas analysis report for the two helium gas bottles (Air Liquide) used in the present work.
Impurity species | Concentration (ppm) | Methods | |
---|---|---|---|
No 1151147 | No 1261172 | ||
Ne | ND a | ND | GC PDHID |
H2 | ND | ND | GC PDHID |
O2 + Ar | 0.604 | 0.428 | GC PDHID |
N2 | 0.678 | 0.568 | GC PDHID |
CO | ND | ND | GC PDHID |
CO2 | ND | ND | GC PDHID |
H2O | 0.055 | 0.038 | Chemiluminescence detector with NH3 converter |
CnHm (CH4) | ND | ND | GC PDHID |
a Less than the limits that the GC PDHID method can detect, namely 1 ppm for Ne, 0.01 ppm for H2, 0.025 ppm for CO, 0.01 ppm for CO2, and 0.01 ppm for CH4.
2.3.2. Pressure control.
In SPRIGT, since a relative primary gas thermometry is used, the absolute pressure accuracy is not so critical. Pressure stability (<4 ppm (k= 1)), however, is critical. Moreover, a constant pressure must be maintained throughout the duration of the measurement, here days or weeks, which is also a challenge [1]. To satisfy both these constraints, a pressure control system was developed. The present configuration implemented at TIPC [42] (figure 9) is an improvement upon the system developed at LNE-Cnam for a measurement of the Boltzmann constant described in Pitre et al [33]. It consists of a temperature stabilized enclosure containing a piston gauge and a home-made gas compensation system. To maintain the piston at a constant height, a servo control loop written in LabVIEWTM software is used that automatically determines in real-time the amount of extra gas required.
Figure 9. The schematic diagram of the pressure control system.
Download figure:
Standard image High-resolution imageThe thermostated enclosure is designed to provide a stable working temperature for the instruments and thereby reduce the impact of external temperature variations on the measured pressure. The design is based on that of Berg et al [43]. At 24.5 °C, while the temperature can vary by as much as 0.5 °C within the volume of the enclosure, at any given point, it is stable to better than 1.5 mK [44].
The reading of the piston gauge (Fluke PG 7601) is used as the input for a Proportional Differential (PD) feedback loop. The piston moves vertically in the cylinder, changing the pressure in the volume beneath the piston until the weight of the piston and load masses are counterbalanced by the force of the pressure acting upon the lower surface. While a rotating piston is the most commonly employed mode for pressure measurement, this turned out to be unsuitable for present purposes [44] and 'regulation non-rotation mode' is used instead. The piston gauge is operated in absolute mode, a turbomolecular pump (Pfeiffer Hipace 300) backed by an oil-free pump (Pfeiffer ACP 28) providing the vacuum above the piston (pvac = 0.4 Pa, u(pvac) = 0.1 Pa).
The gas compensation system is used to supplement the micro-leak of the piston gauge. A first mass flow controller MFC1 (Horiba S48 300/HMT) is used to fix the flow rate. The gas flow is split into two parts using a tee: one going to supplement the piston gauge leakage while the other is evacuated by a dry pump (Pfeiffer ACP 15). The pressure within the system is adjusted using the second mass flow controller MFC2 (Bronkhorst FG-201CV ). A four-way cross is used to connect ballast volume 2 (1 L), the pressure vessel and the piston gauge. In normal operation, since the flow rate from the four-way cross to the piston gauge is very small (about 10–2 sccm 1 ) with almost no gas flowing to the pressure vessel and ballast volume 2, the pressure in the whole system is nearly uniform aside from some hydrostatic head difference. All the test experiments were performed at room temperature (about 24.5 °C), independent of the microwave resonator, the effect of which was simulated using the 1 L ballast volume 2.
In normal operation, MFC1 is used to fix the flow rate at 2 sccm (or some other small values) while the flow rate of MFC2 is actively controlled by the PD loop to maintain the piston height set point. Whenever the piston height lies above the set value, the set-point of MFC2 is reduced, causing the piston to rise. The reverse happens whenever the piston height lies below the set value. Thus, the piston height remains almost constant with only a very small oscillation. The piston height is measured not only by the LVDT height sensor internal to the piston gauge (resolution 10 µm) but also more finely using an external laser interferometer (Keyence LK-G80, resolution 0.1 µm). To estimate the stability of the regulated pressure, two absolute pressure transducers (Paroscientific Digiquartz 745) of range 0–23 psig with parts-per-billion (10–9) resolution, were used to track it in real-time. At pressures close to 60 kPa, the resolution was several millipascals for an integration time τ = 0.7 s. The long-term stability of the pressure near 60 kPa is shown in figure 10 for resonator temperatures of 24.5 K and 5 K.
Figure 10. Long-term pressure stability at 60 kPa for the highest and lowest temperatures used in the present work.
Download figure:
Standard image High-resolution imageAs shown in figure 11, the relative fluctuations at pressures of 30 kPa, 60 kPa, 90 kPa and 120 kPa in the temperature range 5 K–24.5561 K are all less than 0.16 ppm, i.e. 25 times lower than the 4 ppm pressure stability required for SPRIGT at the 0.25 mK level of uncertainty. The improvement over the stability reported in Gao et al [44] is due to a better optimization of the sensor, the use of a larger area piston gauge (10 cm2 versus 1 cm2) and a temperature-controlled enclosure to house it. Note that the use of a piston gauge automatically provides an absolute measurement of the pressure, once the gauge has been calibrated. This aspect is discussed below.
Figure 11. Experimental pressure stability at pressures of 30, 60, 90 and 120 kPa for the temperature range 5 K to 24.5561 K. The apparently lower fluctuations at higher pressure are most likely due to the resolution of the piston gauge. Details of the fluctuations at 5 K and 25.5 K are visible in figure 10 above.
Download figure:
Standard image High-resolution image2.3.3. Pressure calibration.
To obtain thermodynamic temperature, the relation (equation (8) in section 1.2) explicitly requires a value of absolute pressure. The pressure ppiston imposed by the piston balance is given by

where Aθ ,p is the effective area of the piston-cylinder assembly, m the mass load, g the local gravitational acceleration and pvac the residual pressure above the piston. The effective area is affected by thermal expansion and elastic deformation under pressure, and is equal to

where A24.5,0 (in m2) is the effective area at 24.5 °C and zero pressure, θ the piston temperature, °C, αP and αC the respective thermal expansion coefficients of piston and cylinder, °C−1; λ the elastic deformation coefficient of the piston-cylinder assembly, Pa−1; and pnom is the nominal pressure, Pa.
The effective area of the piston was calibrated by the Mass and Force Department at National Institute of Metrology (NIM) of China in 2019, with a pressure-independent value of 980.5225×10−6 m2 at 24.5 °C. The temperature of the gauge is measured using a standard 100 Ω platinum resistance thermometer (Pt 100) inserted into the base, the sensor having being calibrated at NIM in 2019 with an uncertainty of 0.01 °C. In the present work, the working temperature of the piston gauge, was 24.7 °C. A correction for thermal expansion of piston and cylinder supplied by manufacturer is applied to calculate A24.7, p . The k= 1 relative uncertainty for the effective area is 5 ppm.
The masses of the piston and carrying bell were calibrated by the Mass and Force Department at NIM in 2019, with uncertainties better than 2 ppm. The acceleration due to gravity at the height of the piston gauge effective reference plane, ggravity, obtained from on-site measurements made by NIM in 2018, is 9.80111291(49) m · s−2. The vacuum gauge in the piston balance was calibrated at NIM in 2019 with a standard uncertainty of 0.1 Pa. For completeness, an extra uncertainty component to account for the small pressure difference (0.1 Pa) between different piston balance operating modes is included in the uncertainty budget as in Gao et al [44].
The uncertainty budget for the pressure measurement of the piston gauge ppiston at room temperature is shown in table 3. The dominant component at all pressures measured in the present study is the 5 ppm (k = 1) uncertainty in the effective area of the balance piston.
Table 3. Uncertainty budget for determination of pressure using a piston gauge (k = 1).
u(x)/Pa | ||||
---|---|---|---|---|
Source of uncertainty | p = 30 kPa | p = 60 kPa | p = 90 kPa | p = 120 kPa |
Piston mass | 0.006 | 0.006 | 0.006 | 0.006 |
Carrying bell mass | 0.005 | 0.005 | 0.005 | 0.005 |
Mass of weights | 0.030 | 0.08 | 0.087 | 0.11 |
ggravity | 0.003 | 0.006 | 0.009 | 0.012 |
Piston area A24.5,0 | 0.15 | 0.30 | 0.45 | 0.6 |
Piston temeprature θ | 0.003 | 0.006 | 0.01 | 0.013 |
αP+ αC | 0.006 | 0.012 | 0.017 | 0.024 |
pvac | 0.1 | 0.1 | 0.1 | 0.1 |
pmode | 0.1 | 0.1 | 0.1 | 0.1 |
ptotal | 0.2 | 0.34 | 0.48 | 0.63 |
2.3.4. Hydrostatic head correction.
As described above, the pressure control system of SPRIGT is maintained at 24.7 °C, and the pressure ppiston measured by the piston gauge is the gas pressure at the height of the reference plane of the pressure balance. The resonator by contrast is at low temperature (5 K–24.5561 K) and also a lower altitude, so to obtain the pressure p inside the resonator, a hydrostatic pressure head correction (HPC) Δphydrostatic must be evaluated:

To simplify the calculation of the HPC, a design due to Astrov et al [45] was adopted (figure 12). It consists of a mixture of four short, high-thermal conductivity vertical sections (VCT-1, VCT-2, VCT-3, VCT-4) and three long, low-thermal-conductivity horizontal ones (HSST-1, HSST-2, HSST-3). Such that temperature gradients occur only in the horizontal tubing parts. The design of the pressure tube, similar to that described by Sparasci et al [46], helps keep the working gas located at difference altitudes at a constant temperature while working gas volumes at different temperatures are kept at the same altitude.
Figure 12. The model of the pressure tube used to calculate the hydrostatic head correction.
Download figure:
Standard image High-resolution imageEach vertical tube is modelled by sub-dividing it into n straight tube segments i. Before the cryostat was closed, the lengths of all tubing sections were measured and the corresponding uncertainties of each length estimated. For the vertical tubes, copper blocks were used to provide thermal links to the flanges, the surface of each copper-block being set to the same temperature as the flange to which it was connected. The temperature distribution for each segment is assumed to be linear. The calculation was verified in a recent paper [47]. The hydrostatic head correction is thus

where Δhi is the change in height of segment i for each part, and ρi is the corresponding average gas density within it. For p = 60 kPa, we find a relative correction Δphydrostatic/p of 89.61(1.1) ppm at 24.5561 K and 427(4.9) ppm at 5 K. Although these corrections are the largest applied to SPRIGT, the low uncertainty in this correction still has a negligible impact on the final uncertainty.
3. Resistance thermometry for T 90 and T ref
In the following sections, we present the experimental set-up and methods employed to transfer T90 to the SPRIGT experiment via calibrated resistance thermometers. After that, we describe how the reference thermodynamic temperature Tref was transferred from AGT to SPRIGT, via a calibrated platinum-cobalt resistance thermometer.
3.1. Experimental set-up for resistance thermometry to measure T90
In the present work, three calibrated rhodium-iron resistance thermometers (RIRTs) were used to provide traceability to the ITS-90: two had been calibrated by NPL and one by NIST. They are referred to hereafter as RIRT_NPL1, RIRT_NPL3 and RIRT_NIST. The two NPL RIRT thermometers were calibrated with respect to the NPL-75 CVGT scale. The uncertainties in NPL-75 are specified by Berry (1979) [48] with an approximate standard uncertainty u = (0.125 + 0.0125T) in mK. In addition to these uncertainties in the gas thermometer we have to consider the uncertainties in the RIRT calibrations which are used to transfer and apply the scale to other experiments with a standard uncertainty component of about 0.1 mK. A component was added to account for the long-term stability of the RIRT calibrations which were done at this time, 1973–76 with an upper limit of 0.3 mK. Typical final standard uncertainties of 0.25 mK at 5 K and 0.445 mK at 24.55 K were achieved.
Calibration equations linking electrical resistance to temperature were supplied by both laboratories. Table 4 summarizes the main calibration information. RIRT_NPL1 and RIRT_NPL3 were manufactured few decades ago by the British company Tinsley, while RIRT_NIST is a recently-manufactured thermometer from the Chinese company (Kunming Dafang Science and Technology Ltd.). Tinsley has since ceased production of the RIRT so the present work provides a test of the only equivalent currently available. As it turned out, all showed excellent stability and similar behavior, as described below in section 3.4.
Table 4. Bridges and standard resistors used for implementation of ITS-90 temperatures by rhodium-iron resistance thermometers (RIRTs).
RIRT | Bridge | Standard 10 Ω resistor |
---|---|---|
RIRT_NIST RIRT_NPL3 | Wika F18 | Tinsley 5685A, SN 7800BP0 |
RIRT_NPL1 | Wika F900 | Tinsley 5685A, SN 1580409 |
The three RIRTs were mounted in contact with the external face of the microwave resonator, inside thermometer wells bored in the bulk copper (as in Pitre et al [33]). They were connected to the measuring equipment by cryogenic four-wire cables, thermally anchored to every thermal stages of the cryostat. Once installed, thermometers were left in place to avoid any mechanical stress and thereby preserve their stability.
The resistances of the RIRTs were measured using two WIKA (formerly ASL) AC bridges, models F900 and F18, with two 10 Ω standard resistors (Tinsley model 5685A, serial numbers 7800BP0 and 1580409, respectively), see table 4. The resistors were immersed in an oil bath (Aikom Instruments MR 5100-L) with temperature stability better than 1 mK. During the experiment, RIRT_NPL1 was used as the reference thermometer and its resistance measured continuously with the F900 bridge. The F18 bridge was used to measure all the other thermometers. A manual scan procedure was implemented, following the sequence shown in figure 13. Usually, the manual procedure was repeated for each pressure and temperature. Whenever the self-heating measurement for each thermometer was performed, one of the other reference thermometers (RIRT_NPL1 or RIRT_NPL3), was used not only to monitor temperature change of the temperature control but also to compare with the self-heating measuring thermometer. The values of the two currents of 0.5 mA (run 8) and 1.0 mA (runs 9 and 10) were used for the measuring and self-heating tests for each thermometer. All measurements were corrected to remove self-heating effects.
Figure 13. Self-heating measurements and comparisons (I = 0.5 mA or 1.0 mA). The gap in the lower part corresponds to the time taken to change from RIRT_NIST to RIRT_NPL3 using the same bridge.
Download figure:
Standard image High-resolution image3.2. T90 calibration of the rhodium-iron resistance thermometers
The basic calibration information for the three RIRTs is listed in Table 5. RIRT_NPL1 and RIRT_NPL3 were originally calibrated between 2.6 K and 27.1 K by NPL in 1975, using the NPL-75 temperature scale [48], gas thermometry scale realized at NPL in 1975, which can be converted to ITS-90 using the quadratic function supplied in Rusby et al [8]:

Table 5. Basic calibration information for the three RIRTs.
Name | Calibrated by | SN No. | Temperature range | Input format |
---|---|---|---|---|
RIRT_NPL1 | NPL | 226242 | NPL-75 with adjustment to ITS-90 for T < 24.5561 K | Equations for T = f(R) at 0.3 mA |
RIRT_NPL3 | 226950 | |||
RIRT_NIST1 | NIST | 20107 | 0.65 K < T < 24.5561 K | Equation for R = f(T) at 0 mA |
Values of TNPL-75 were obtained for RIRT_NPL1 and RIRT_NPL3 by fitting the measured resistance values with an 11th order calibration polynomial determined by NPL. No self-heating correction was applied during the calibration, so calibration results were given in terms of resistances for the current of 0.3 mA employed. While this is not the usual situation, as generally calibrations are specified for 0 mA, we estimate self-heating should not exceed 0.8 mK. Calibration standard uncertainties lie below 0.50 mK for 2.6 K < T90 < 27.1 K.
RIRT_NIST was calibrated by NIST at temperatures from 0.65 K to 24.5561 K (the neon triple point) and at additional temperatures of 83.806 K (the argon triple point) and 273.16 K (the water triple point). It was calibrated under isothermal conditions in vacuum, by comparison with both a reference thermometer and a control thermometer maintained at NIST [49]. For the range 0.65 K–24.556 K, the reference and control thermometers were RIRT from Tinsley B174 and RIRT from Tinsley A128 respectively, while for temperatures above 24.5561 K, they were SPRT 1812282 and SPRT 1842385, respectively. The reference and control thermometers had been calibrated at NIST from direct realizations of the ITS-90: 3He vapour pressure (0.65 K–2.0 K), 4He vapour pressure (2 K–5 K), an Interpolating Constant Volume Gas Thermometer (5 K–24.556 K), and NIST realizations of the ITS-90 fixed points of equilibrium hydrogen (e-H2), neon, argon, and water. The resistance of the thermometer was corrected for self-heating, to provide values at 0 mA, and fitted with an 11th order polynomial with respect to temperature. For a detailed description of the uncertainties see the NIST special publication vol 250, p91, (2013) [50]. Calibration standard uncertainties of the RIRT_NIST1 were less than 0.58 mK for 0.65 K < T90 < 24.5561 K.
3.3. Implementation of T90 for SPRIGT
The ITS-90 is realized at TIPC-CAS using, T90,CAS, a weighted average of the values yielded by the three calibrated RIRTs described above. We define:

with a standard uncertainty

where TNPL1, TNPL3, and TNIST are the T90 temperatures provided by RIRT_NPL1, RIRT_NPL3 and RIRT_NIST respectively. The corresponding standard uncertainties u (TNPL1), u (TNPL3) and u (TNIST) comprise both calibration and measurement uncertainties. The latter includes uncertainties originating from measurement noise, self-heating corrections, heat flow, resistance bridge linearity, reference resistor calibration, thermometer stability and temperature set-point stability.
The realization of the ITS-90 within the SPRIGT apparatus was checked by performing three independent experimental runs, named 'run 8', 'run 9' and 'run 10'. There, the temperature of the resonator was controlled by a servo loop, in which another RIRT was employed as the sensitive element. To eliminate possible instabilities of the sensing thermometer, the resistance of RIRT_NPL1 was recorded in parallel, to verify the temperature remained stable around the desired set point. As a precaution, an uncertainty contribution of a few microkelvin was still included in the uncertainty budget, to account for any (small) instability of RIRT_NPL1.
In each run, temperature set-points were decreased stepwise from 25 K to 5 K. In run 8, temperatures were measured at 10 set points, as opposed to 20 for runs 9 and 10. For all set points, once the temperature was stable to within few microkelvin tens, data from the three calibrated RIRTs were acquired.
For each measured value of Tx
, the difference Tx
–T90,CAS and the associated uncertainty were evaluated. A surprisingly good agreement was observed between all three thermometers, with 93% of Tx
–T90,CAS measurements lying within ± 0.2 mK of the mean. The calculated standard uncertainty u (T90,CAS) turned out to be less than 0.21 mK over the whole temperature range from 5 K to 25 K (see table 9 below). The present uncertainties u (T90,CAS) are generally consistent with the CCT-K1 Key Comparison [8] in this range where the standard uncertainty was 0.12 mK at 5 K and 0.16 mK up to 24.5 K [51]. Figure 14 summarizes these results of the three RIRTs during the three measurement runs 8, 9 and 10. At each temperature for each thermometer, there are two or three plotted points because they were measured in two or three different runs, where the measured temperature points were not exactly the same. This can be also seen in figure 15.
Figure 14. Measured values of Tx –T90,CAS. Uncertainty bars represent u (Tx –T90,CAS), while the green shaded area corresponds to its standard uncertainty u (T90,CAS).
Download figure:
Standard image High-resolution imageFigure 15. Stability of the three RIRTs during the three measurement runs 8, 9 and 10. Whatever the thermometer, the standard deviation of the data never exceeds 0.03 mK.
Download figure:
Standard image High-resolution imageThe stability and the reproducibility of the three RIRTs were verified in the three runs 8, 9 and 10 not only at the same temperature (5 K to 24.5561 K) under vacuum but also, albeit pairwise, at different pressures. While the stability on an isotherm is less than 0.5 mK, it was possible to reproduce exactly the same temperature at a given setpoint. The mean temperatures for each run differed slightly (by a few millikelvin). For this reason, a small correction was applied to the experimental data, so as to align the mean values of the temperatures yielded by a given thermometry from one run to another.
The scatter of measurements for the three RIRTs is reproduced in figure 15. Whatever the thermometer, the standard deviation of the results never exceeds 0.03 mK. We take this value as the uncertainty related to the stability of the RIRTs.
In principle, because the RIRTs are embedded within the resonator, self-heating should be unaffected by the conductivity of the surrounding gas, itself related to gas pressure. Nevertheless, to quantify its possible impact on the present work, in run 10, the self-heating of RIRT_NPL1 was measured not only under vacuum but also at the four pressures used for SPRIGT (30, 60, 90 and 120 kPa). Figure 16 shows how the self-heating of the RIRT_NPL1 varies between 5 K and 24.5561 K, under vacuum and on four isobars. We noticed that self-heating is reduced whenever the pressure is increased, especially at lower temperatures as clearly shown in figure 17, where the self-heating under vacuum is taken as reference. From these measurements we inferred that the heat transfer between the thermometer and the resonator is improved by the presence of helium, especially at low temperatures where condensation of 4He sealed in the RIRTs further increases the thermal link. Such an effect had never been observed previously at this level with other capsule thermometers of nominally identically diameter, which suggests the presence of trapped gas within the thermometer wells.
Figure 16. Self-heating effect of the NPL1_RIRT at different isobars. A function was fitted to quantify the self-heating of RIRT_NPL1 under vacuum as a function of its temperature, Tsh/mK = 1.684 × exp(− 0.5232 × TNPL1/K) + 0.7249 × exp(− 0.0204 × TNPL1/K). This was then used to estimate the self-heating of NPL1 at different pressures, the difference being the experimental self-heating values under pressure minus those calculated for the vacuum based on the above relationship.
Download figure:
Standard image High-resolution imageFigure 17. Self-heating difference of the thermometer NPL1_RIRT with respect to vacuum measured at four different isobars. At higher pressures, the greater conductivity of the surrounding gas most likely explains the reduction of the self-heating effect.
Download figure:
Standard image High-resolution imageTo conclude this sub-section, thanks to the use of calibrated RIRTs, ITS-90 has been implemented in the SPRIGT set-up at TIPC-CAS with an uncertainty of 0.25 mK using the mean of two independent laboratory scales established over three decades apart, using thermometers from two totally unrelated manufacturers. Moreover, the repeatability of the scale is a mere 0.01 mK. Given that one of the manufacturers is still in business, this bodes well for the future of low temperature thermometry.
3.4. Transfer of Tref from AGT to SPRIGT
A Standard Platinum Cobalt Resistance Thermometer (PCRT), serial number RS144.08 produced by Chino Corporation in 2016, was used to transfer the reference thermodynamic temperature Tref from the AGT experiment realized at LNE-Cnam to the SPRIGT apparatus at TIPC. In this work, we chose a Tref near 24.5561 K, i.e. the temperature of the neon triple point defined by ITS-90.
To verify the stability of the PCRT, two calibrations at the triple point of neon were performed at LNE-Cnam in 2018 and 2019. The difference between the two calibrations amounted to 0.19(45) mK i.e. less than half of the standard uncertainty. The thermometer was considered stable within measurement uncertainties. Thereafter, the PCRT was installed in one of the AGT resonators at LNE-Cnam, specifically TCU1 [52]. Calibration was carried out in isothermal conditions with 4He, at 10 pressures between 30 kPa and 120 kPa. The AGT uncertainty included contributions related to repeatability between two cooling and warming experiments, pressure calibration, gas impurities estimated with a liquid helium cold trap, microwave and mode fitting resolution, as detailed in references [11, 25, 53]. It was estimated to be 0.15 mK at 24.554948 K. Combined with the uncertainty from CPRT self-heating and stability, this yields a final combined uncertainty u (Tref) = 0.158 mK in AGT (see table 6). After the calibration, the PCRT was immediately shipped to China and transferred to the wire-scale at TIPC composed of the three RIRTs and two CSPRTs.
Table 6. The uncertainty budget (in mK unless otherwise indicated) for calibration of the platinum-cobalt resistance thermometer (PCRT) using acoustic gas thermometry (AGT).
Component | T = 24.554948 K |
---|---|
AGT | |
Repeatability between runs1 and 2 | 0.098 |
Microwave determination | 0.093 |
Pressure calibration | 0.045 |
Impurity | 0.025 |
Acoustic mode inconsistency | 0.007 |
Others | <1 μK |
PCRT | |
Repeatability and self-heating | 0.049 |
Standard resistance | ≪1 μK |
Bridge | ≪1 μK |
Combined standard uncertainty | 0.158 |
Concerning the use of PCRTs in the SPRIGT system, an additional uncertainty of about 0.021 mK for Tref was introduced, the quadratic sum of uncertainties due to the transfer of the PCRT (standard uncertainty 0.020 mK) and its stability (0.007 mK). Finally, a combined standard uncertainty u (Tref) = 0.159 mK was obtained for SPRIGT measurements.
Following the successful implementation of ITS-90 at the desired level of accuracy and subsequent transfer to the SPRIGT apparatus of reference temperatures measured using AGT, an extensive series of tests was undertaken to evaluate the performance of the microwave resonator and associated apparatus. These are described below in section 4.
4. Test of the resonant cavity and associated microwave apparatus
SPRIGT is based on the change of the resonance frequency of a gas-filled microwave cavity. In the present experiment, the relative change to be detected is small (a few parts in 103), so even tiny spurious effects could impact measurement uncertainty. Here we present three different analyses carried to this end. They are based upon the measurements of four microwave modes (i.e. triplets) yielding the lowest fitted frequency uncertainty among the eight ones accessible within the frequency range covered by the network analyzer [35].
First, we present a measurement of thermal expansion under vacuum. Secondly, we describe a comparison of frequency measurements when 4He gas is introduced into the cavity. Finally, we provide a comparison of nominally identical temperatures determined by SPRIGT, using different pressures and mode.
4.1. Microwave data in vacuum
The test under vacuum was comprised of three series of measurements. In the first, the thermal expansion of the copper resonator was measured and values were compared with data from the literature. In the second, the mechanical stability of the resonator was assessed [54] when it was subjected to several temperature cycles between 5 K and 25 K. In the third, the reproducibility of microwave data was studied after both cables and feedthroughs were changed. Based on the results of the first three tests and input data on thermal expansion, the consistency of microwave spectra at several temperatures was checked.
4.1.1. Thermal expansion analysis in vacuum.
The experimental linear thermal expansion of the resonator was calculated from microwave measurements carried out sequentially under vacuum during the 30 h or so it took to cool the system from 290 K to 5 K. From the experimental microwave data, resonance frequencies fn and half-widths gn of each microwave mode n (here n= {TM11, TE11, TM12, TE13) were determined, using the Levenberg-Marquardt least-squares fitting algorithm. Then, applying a second-order shape correction [55, 56], a waveguide correction and equation (2) presented in the literature [57], the equivalent spherical radius aeq of the quasi-spherical resonator was obtained for each mode. Figure 18 shows the values of aeq obtained from the four microwave modes. The uncertainty bars would be smaller than the data symbols and are thus omitted. Although measurements were carried out while the system temperature was varying, the variation was slow enough for one to consider that the measured aeq values approximate the steady-state ones well enough.
Figure 18. The equivalent radii aeq for the four modes TM11, TE11, TM12, TE13 measured in vacuum during cooling down. (Note: for greater clarity only some of the measurements are plotted).
Download figure:
Standard image High-resolution imageThe linear thermal expansion αL was calculated for each mode as the derivative of aeq with respect to the temperature. Three experimental values of aeq, recorded at incrementally separated temperatures T90, p , T90, p+1 and T90, p+2 (subscripts p, p+ 1 and p + 2 identify the three measurements), were combined to determine αL:

To extract a value of the linear thermal expansion of copper from the literature, a logarithmic polynomial equation based on published data for temperature from 4 K to 300 K was used [26], the corrected coefficients are listed in Table 4 in reference [58].
Literature αL,literature and experimental αL data are plotted in figure 19, and their differences αL in figure 20. For modes TM11, TE11 and TM12, the differences are less than ±σ(αL, fit) at temperatures from 5 K to 200 K, and less than ±2σ(αL, fit) from 210 K to 290 K while for mode TE13 mode, they are less than ±σ(αL, fit) at temperatures from 5 K to 150 K and less than ±2σ(αL, fit) from 150 K to 200 K. Globally, αL agrees better with αL,literature at relatively low temperatures than at higher ones. We attribute this observation to the non-steady temperature during the measurement. The observed increased noise at higher temperatures is related to the microwave scanning bandwidth.
Figure 19. Experimental linear thermal expansion αL determined from modes TM11, TE11, TM12, and TE13 and those calculated from using the formula of Simon et al [26].
Download figure:
Standard image High-resolution imageFigure 20. Difference between experimental linear thermal expansion coefficient and those calculated using the formula of Simon et al [26].
Download figure:
Standard image High-resolution imageThe differences between αL obtained in the present work and αL, literature, plotted in figure 20, are the same order of magnitude as those in Rourke and Hill [58], which is one of the most accurate experimental determinations of αL performed by microwave measurements. We take the good agreement between our results for αL and literature values as an indicator of the quality of our microwave measurements performed under vacuum.
4.1.2. Mechanical stability: thermal cycling under vacuum.
A test of the mechanical stability of the resonator was carried out by performing several thermal cycles under vacuum, where the temperature was raised from 5 K to 25 K and then lowered back to 5 K several times.
Three runs data were used to study mechanical stability, referred to hereafter as runs 8, 9 and 10. In the process, it became clear that the behavior of microwave cables and antennas had to be better understood. The parameter measured was the average amplitude |A| given by equation (11) for mode TM11. In run 8, an unexpected drop in signal amplitude was observed after the second thermal cycle (figure 21). This was found to be due to a loose antenna. In run 9, with the antenna solidly re-attached, no such jumps were detected but the stability was still insufficient. In both runs 8 and 9, the apparatus was configured with rigid coaxial cables, connected directly to the resonator antennas.
Figure 21. Effect of thermal cycles on the modulus of the average of the resonance amplitude of the TM11 microwave mode under vacuum as defined in equation (11) at temperatures from 5 K to 25 K (run 8). The unexpected fall in signal amplitude in the after the second cycle was found to be due to a loose antenna. The increased amplitude compared with figure 22 and later is due to the use of pre-amplifiers [54] that were subsequently removed.
Download figure:
Standard image High-resolution imageAfter run 9, the rigid coaxial cables were replaced with semi-rigid ones and, to increase the quality of microwave signals, the microwave feedthroughs used up to then were replaced with improved, low-noise versions (Allectra:242-SMAD 18G-C16). Following this modification, a new measurement campaign, identified as run 10, was begun. The system was cooled down to 5 K, after which temperature cycles between 25 K and 5 K were repeated. Figure 22 shows the evolution of |A| in the new configuration. This time, no sudden change in |A| was observed from one cycle to another. In the light of the above observations, the experimental procedure was tightened. Henceforth, even with the improved cables and feedthroughs definitively in place, as a precautionary measure, five thermal cycles under vacuum are performed systematically as a prelude to SPRIGT in the gas-filled resonator.
Figure 22. Evolution of the average amplitude of |A| in equation (11) measured from the vacuum frequency of mode TM11 during run 10 i.e. with semi-rigid cables linked to antennas.
Download figure:
Standard image High-resolution imageThe main conclusion from the results exposed here is that the new microwave configuration, with semi-rigid coaxial cables connected directly to antennas, appears much more stable than the previous one with rigid ones. A further confirmation is given in the next section, where the effect of cable and feedthrough changes on resonance frequencies and half-widths is presented.
4.1.3. Reproducibility of microwave measurements after change of cables and feedthroughs.
The effect of the aforementioned configuration changes (section 4.1.2) on reproducibility of microwave resonance half-widths and frequencies, was calculated by comparing the data of runs 9 and 10. The comparison shows that microwave measurements remain essentially consistent, as relative changes in resonance frequencies fn and half-widths gn were small, of the order of a few parts in 109. To avoid any perturbation related to volume change, the comparison was performed with the resonator below 25 K, i.e. in a temperature range where thermal expansion of copper is minimum. In this range, the relative change in the radius of the quasi-spherical resonator is less than 3 × 10−6 for a temperature change of 20 K, i.e. from 25 K down to 5 K. This compares with a relative shift of 330 × 10−6 for a change from 310 K to 290 K.
The stability of the half-width gn
was determined by comparing the measurements of runs 9 and 10, both performed at temperatures between 5 K and 24.5561 K. In figure 23 the relative half-width change is plotted as a function of temperature:

Figure 23. Relative half-width changes of the four microwave modes between two independent runs in vacuum using different microwave cables and feedthroughs.
Download figure:
Standard image High-resolution imageRelative half-width changes varied differently according to the mode in question. For modes TM11, TE11 and TE13, the relative changes were small, within 0.2%, 0.1% and 0.3%, respectively. By contrast, for mode TM12, the relative half-width changes were, unexpectedly 40 to 270 times larger than those for the other three modes.
As regards modes TM11, TE11 and TE13, the impact of the changes is very limited in terms of the perturbations to gn/fn . Below 25 K, the electrical conductivity of copper is 8.6 × 108 Ω–1 · m–1 at 25 K, therefore gn is already small and gn/fn amounts to only a few parts in 106. This in turn means a variation of few parts in 103 in gn corresponding to an even tinier change in gn/fn of a few parts in 109. The net result is that temperatures measured using the present SPRIGT apparatus are essentially immune to alterations of the configuration of microwave cables and feedthroughs.
The stability of resonance frequencies fn, with respect to the changes of microwaves cables and feedthroughs performed between runs 9 and 10, was verified using the following equation:
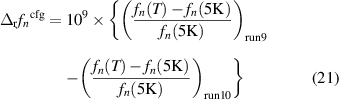
Figure 24 shows a plot of as a function of temperature. For modes TM11, TE11, TE13 the changes are all below 3 parts in 109. Once again, the behavior for mode TM12, is anomalous, with a ramp increasing to 20 parts in 109 at 25 K.
Figure 24. Relative change of mode frequencies in parts per billion between two runs (9 and 10) after improvements to cables and feedthroughs.
Download figure:
Standard image High-resolution imageOn the basis of the aforementioned observations, it was decided to reject mode TM12 for thermometric measurements.
4.1.4. Comparison of experimental microwave data in vacuum with theory.
To determine whether electromagnetic field perturbations remained constant with temperature, the excess half-widths were analyzed with the resonator under vacuum. Tests were performed in the configuration of run 10, (semi-rigid coaxial cables and improved cable feedthroughs). This approach is standard in AGT [11]. Excess half-widths correspond to the difference between experimental (gn ,meas) and calculated (gn ,calc) half-widths. Relative excess half-widths (in parts per million) are defined here as:

where the subscripts x, y, and z indicate that x-, y- and z- components of each mode are treated separately.
To determine gn,calc, equations (6) and (7) in May et al [35] were used, which require as input the electrical conductivity of the resonator surface. In a similar way to the procedure described in Pitre et al [33], the latter was determined from the experimental half-width of the mode TE11z (z-axis component of TE11), by setting (gTE11,meas –gTE11,calc)z = 0. This mode was used because of its low sensitivity to the perturbation generated by the equatorial seam of the resonator.
The relative excess half-widths of the triplet components of the other modes (including TE11x,y) were measured at several temperatures between 5 K and 24.5 K. Figure 25 shows the result. For modes TE11 and TE13, the relative excess half-widths are small, at most 0.05 × 10-6 and 0.19 × 10-6 respectively. For mode TM11 they are larger, but still less than 0.65 × 10-6. For mode TM12, however, they can be as large as 1.60 × 10-6. Aside from this mode, the other excess half-widths are comparable with those observed in measurements of the Boltzmann constant using AGT [33].
Figure 25. Variation of the relative excess half-width as a function of the resonator temperature.
Download figure:
Standard image High-resolution imageThis analysis confirms that mode TM12 is unsuitable for accurate thermometry, as indicated previously by the results described in section 4.1.3. At present no satisfactory explanation for the behavior has been found. An interaction with a cable resonance was hypothesized, similar to that described in Underwood et al [59].
Consequently, we decided to discard mode TM12 and henceforth base all SPRIGT thermodynamic temperature measurements on modes TM11, TE11 and TE13.
4.2. Consistency of microwave data with pressure
As the letters SP in the acronym suggest, SPRIGT is intended to be used at a single gas pressure at any temperature. To identify the most suitable working pressure, tests were performed in the configuration of run 10 at four different pressures pisobar = {30, 60, 90, 120} kPa, using high purity 4He as described in section 2.3. Figure 26 shows the relative half-width change on the four isobars mentioned , with respect to the microwave measurement under vacuum (p = 0).

Figure 26. Relative half-width changes of the four isobars comparing with those in vacuum, compared for four microwave modes.
Download figure:
Standard image High-resolution imageIt amounted to less than 0.25% for modes TM11 and TE13 and less than 1.5% for mode TE11. Mode TM12 changed by 1 to 2 orders of magnitude more than the other three, confirming once again its unsuitability for accurate thermometry. Surprisingly, on a given isobar, all the modes showed a larger change at low temperatures than at higher ones, while, at a given temperature, the half-width change was larger at higher pressures than at lower ones. No convincing explanation for both these temperature and pressure dependencies could be found, however, further investigations are in progress. Nevertheless, the impact on the final temperature measurement is rather limited. Below 25 K, the electrical conductivity of copper is large, so gn is small and gn/fn amounts to few parts in 106. In this case, a variation of few parts in 103 in gn corresponds to one of a few parts in 109 in gn/fn , with a very limited impact on the thermodynamic temperature uncertainty, less than 0.03 mK as shown later in figure 28.
These results are satisfactory and prove that the present SPRIGT apparatus can be used at all pressures between 30 kPa and 120 kPa with a similar accuracy.
4.3. Redundancy at several pressures
As done for gn in the previous section 4.2, we checked the consistency of resonance frequency (fn ) measurements at the four pressures pisobar mentioned above. To facilitate interpretation, data are plotted as variations of thermodynamic temperature rather than of resonance frequency. To extract temperature values, the procedure described in section 1.2 was employed. The reference temperature Tref was measured by a Platinum Cobalt resistance Thermometer (PCRT) calibrated by Acoustic Gas Thermometry at LNE-Cnam. In the present work, measurements were made first in vacuum to determine < fn + gn > for the microwave modes TM11, TE11 and TE13 at each T90 setpoint from 5 K to 24.5561 K. Thereafter, the same modes were studied with a resonator filled with high-purity 4He at 30, 60, 90, and 120 kPa.
Figure 27 plots the difference between the thermodynamic temperature Tn calculated individually using each mode and the average temperature Tavg of the three modes, for each isobar. The absolute value of most of the differences (Tmode–Tavg) lies below 0.08 mK. The mutual agreement of thermodynamic temperature of different microwave modes for 30 kPa and 60 kPa is better than that at 90 kPa and 120 kPa. A possible reason is that the relative half-width changes of the three modes at low pressures are smaller than those at higher ones. Another reason is the relative half-width change of mode TE11, which is larger than that of TM11 and TE13, especially at lower temperatures, as shown in figure 27. To account for these thermodynamic temperature differences, we introduce an additional uncertainty component u(Tn ). The uncertainty was estimated by the standard deviation of T determined from the three microwave modes for each measurement point. Figure 28 shows the evolution of the values u(Tn ) for each isobar, almost all absolute values lying below 0.07 mK. Combining data from the four isobars, we obtain a weighted uncertainty uwm(Tmode) that never exceeds 0.026 mK at temperatures from 5 K to 24.5 K.
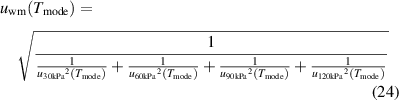
Figure 27. Comparison of thermodynamic temperature deduced from three microwave modes with respect to their average values for four different pressures of 4He.
Download figure:
Standard image High-resolution imageFigure 28. Thermodynamic temperature uncertainty component from average of three microwave modes at four different isobars together with the weighted uncertainty.
Download figure:
Standard image High-resolution imageThe results presented here show that the measurement of thermodynamic temperature is independent of the frequency, especially if we consider that the frequency of mode TE13 (10.4 GHz) is about four times that of TM11 (2.6 GHz).
5. Determination of T – T 90
In this section, we present the results of our measurements of T–T90 at 17 different temperatures between 5 K and 24.5561 K. As measurements were performed on the four isobars at 30 kPa, 60 kPa, 90 kPa and 120 kPa, a total of 68 temperatures were measured. Each temperature measurement takes six hours, which includes changing the temperature from the previous set-point to the new one, allowing it to stabilize, scanning and recording the three microwave modes (TM11, TE11 and TE13) and taking data from the three RIRTs. Work was performed in shifts so that, in one day, up to four values of T–T90 could be determined. SPRIGT turned out to be much quicker to perform than traditional RIGT or AGT, where the measurement of even a single temperature typically takes between four and seven days [13, 37].
In this section, for each measurement of T, the uncertainty budget is estimated. Thereafter, values of T–T90 are calculated. To calculate their uncertainties correctly, we include correlations between measurements. Finally, these differences are compared with the results of the equation provided by Fischer et al [4].
5.1. Uncertainty budget for thermodynamic temperature
The thermodynamic temperature T is determined from experimental microwave measurements with a numerical iteration method, as presented in section 1.2. The same numerical relation can also be used to estimate the derivatives of T, which is necessary to calculate the uncertainty budget. T is a function of the uncorrelated input parameters . A suitable expression for T in terms of them can be obtained by iterative substitution using equations (6)–(9). Using the same equations, its derivatives with respect to each parameter can also be evaluated numerically. Each input parameter has its own standard uncertainty
, and these components are combined using the law of uncertainty propagation for uncorrelated variables [60]:

where

Here we provide a brief explanation on how the values of are determined in the present work. Ideally, a presentation in decreasing order of importance would be desirable. However, the relative weight of a given contribution depends on the temperature and pressure in question. At the highest temperatures, near 24.5561 K, the uncertainty of the reference temperature Tref is dominant. At the lowest temperatures near 5 K, the uncertainty of the dielectric virial coefficient
predominates. The next largest contribution, though much smaller than the two above, arises from the uncertainties of pressure and frequency measurements as ascertained from the dispersion among the three different microwave modes employed. Thereafter, follows the uncertainty of the isothermal compressibility of the resonator kT. Although the remaining components have a negligible impact, they are still quantified for completeness. Detailed explanations are as follows:
- Values of the coefficients B, C, D,
,
,
,
of 4He and their uncertainties are taken from the most up-to-date ab initio calculations (B [70], C [61], D [62], Aμ [63], {A
, B
, C
} as presented in section 1.2). Given that the effect of the uncertainty in A
and Aµ is well below 1 µK (∀ p, T) their contribution is omitted from the graphs and tables below.
- The uncertainty of the reference thermodynamic temperature,
, is the combination of uncertainties coming from the PCRT AGT calibration (158 µK) and additional uncertainty of about 20 µK for transfer uncertainty and the stability of the temperature control system 7 µK.
- The uncertainty of the pressure, up , is a combination of that of the absolute pressure calibration up, ab, pressure stability up, st and the uncertainty of the static head correction up, hy, described in sections 2.4 and 2.5.
- The frequency uncertainties
and
for a given microwave mode are the standard deviations of the frequency measurements performed at a given temperature under pressure and in vacuum respectively. They are the combined standard deviation of 22 to 66 repeated frequency measurements and the fitting uncertainty of each measurement for the same mode. No uncertainty related to the reference frequency standard is included since it is negligible (the relative Allan standard deviation in the frequency after 100 s is less than 2 × 10−12).
- The temperature variation of the isothermal compressibility kT of the OFHC copper resonator is calculated using equation (25) of Gaiser and Fellmuth [64] relating the value of kT to that of the thermal expansion coefficient αL. In the present work, the value of the latter was determined from the relative shift of microwave frequencies as a function of temperature in (see section 4.1). The relative uncertainty in kT,
is determined by applying the law of propagation of uncertainty to the same equation and amounts to 0.28%. Note that in SPRIGT, thanks to the weak temperature dependence of the isothermal compressibility, the uncertainty in the measured temperature is largely insensitive to the value of
, because the same gas pressure is used for measurements performed at different temperatures.
- The uncertainty contributions related to the average temperature are deduced using the three selected modes TM11, TE11 and TE13 and are calculated from their standard deviations.
The uncertainty budget for temperature measurements is shown in table 7. Combining the uncertainty components in quadrature from all sources listed in the table, we obtain the standard uncertainties in the thermodynamic temperature at a single pressure. As an example, figures 29, 30, 31 and 32 show the uncertainty components for measurements performed on each isobar. In the temperature range 15 K–25 K, the dominant contribution is the uncertainty of the reference temperature. It can be seen that is the main component at high temperature (9 K–25 K). At lower temperatures, the uncertainties of the second dielectric virial coefficient B
and the third density virial coefficient C become ever more significant as the helium departs from ideal gas behavior. At the highest measured temperature near 24.5561 K, the total uncertainty on a given isobar ranges from 171 µK to 241 µK. At the lowest temperature, near 5 K, measurements at 30 kPa have by far the lowest uncertainty, namely 68 µK.
Figure 29. Uncertainty components at 30 kPa.
Download figure:
Standard image High-resolution imageFigure 30. Uncertainty components at 60 kPa.
Download figure:
Standard image High-resolution imageFigure 31. Uncertainty components at 90 kPa.
Download figure:
Standard image High-resolution imageFigure 32. Uncertainty components at 120 kPa.
Download figure:
Standard image High-resolution imageTable 7. Uncertainty budget for the determination of thermodynamic temperature by SPRIGT at temperatures close to 5 K, 13.8 K and 24.5561 K on four different isobars. All values are in microkelvin. The five largest contributions in each column are in boldface.
T = 24.55559 K | T = 13.80435 K | T = 5.00023 K | ||||||||||
---|---|---|---|---|---|---|---|---|---|---|---|---|
Uncertainty component | 30 kPa | 60 kPa | 90 kPa | 120 kPa | 30 kPa | 60 kPa | 90 kPa | 120 kPa | 30 kPa | 60 kPa | 90 kPa | 120 kPa |
Reference temperature ![]() | 159 | 159 | 159 | 159 | 50 | 50 | 50 | 50 | 6 | 5 | 5 | 4 |
Temperature stability | 7 | 8 | 10 | 8 | 4 | 5 | 5 | 3 | 2 | 2 | 2 | 2 |
Microwave self-heating | 2 | 2 | 2 | 2 | 2 | 2 | 2 | 2 | 2 | 2 | 2 | 2 |
Vacuum frequency ![]() | 114 | 57 | 38 | 29 | 41 | 20 | 13 | 10 | 4 | 2 | 1 | 1 |
Frequency under pressure ![]() | 134 | 55 | 35 | 24 | 40 | 18 | 11 | 8 | 5 | 2 | 1 | 1 |
Compressibility ![]() | 43 | 43 | 43 | 43 | 13 | 13 | 13 | 13 | 2 | 1 | 1 | 1 |
Absolute pressure ![]() | <1 | <1 | <1 | <1 | 42 | 35 | 33 | 33 | 28 | 24 | 23 | 23 |
Hydrostatic head ![]() | <1 | <1 | <1 | <1 | 5 | 4 | 4 | 3 | 10 | 8 | 7 | 6 |
Pressure stability ![]() | 2 | 2 | 1 | 1 | 2 | 1 | 1 | 1 | 1 | <1 | <1 | 1 |
Second dielectric virial coefficient ![]() | 0 | <1 | 0 | <1 | 26 | 53 | 79 | 106 | 37 | 73 | 109 | 141 |
Third dielectric virial coefficient ![]() | <1 | <1 | <1 | <1 | 1 | 2 | 5 | 10 | 2 | 9 | 21 | 39 |
Second density virial coefficient ![]() | <1 | <1 | <1 | <1 | 7 | 15 | 22 | 30 | 29 | 65 | 111 | 175 |
Third density virial coefficient ![]() | <1 | <1 | <1 | <1 | 1 | 4 | 9 | 16 | 30 | 143 | 393 | 894 |
Fourth density virial coefficient ![]() | <1 | <1 | <1 | <1 | <1 | <1 | <1 | <1 | 1 | 9 | 40 | 133 |
T from mode average (TM11, TE11, TE13) | <1 | <1 | <1 | <1 | 34 | 51 | 61 | 69 | 25 | 34 | 30 | 14 |
T combined standard uncertainty (single pressure) | 241 | 183 | 173 | 171 | 99 | 101 | 121 | 146 | 68 | 179 | 427 | 933 |
T standard uncertainty 2 | 164 | 70 | 63 |
2 Averaged over isobars, including correlations with reference temperature measurement.
To evaluate the uncertainty of the weighted average value of TSPRIGT obtained by combining measurements on the four isobars, we need to include covariances. Specifically, values of T on each isobar are correlated with those of the reference temperature obtained using the AGT value and platinum-cobalt resistance thermometer transfer standard. To perform the calculation, we write the 4 × 4 variance-covariance matrix for measurements of TSPRIGT on the four isobars (equation (27), with an example for 13.8 K). The diagonal elements are the variances of TSPRIGT at the four different pressures. The off-diagonal elements are the covariances between measurements of TSPRIGT at two different pressures. Assuming the only correlated variable is the reference temperature, whose uncertainty is constant on each isobar, we can write the off-diagonal elements as the square of the uncertainty of Tref. This approach was inspired by that used by Moldover et al [65] to calculate an average value of the Boltzmann constant from several AGT experiments.
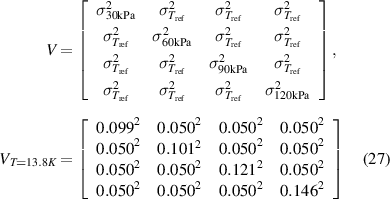
The correlated weighted uncertainty is always larger than the uncorrelated one. A subtle point here is that, at temperatures lower than Tref = 24.5561 K, although the uncertainty of the reference temperature remains constant at 161 µK, its contribution to the uncertainty in TSPRIGT gets smaller. This is due to the effect of the factor (n2(T, p) − 1)/(n2(Tref, p) − 1) in equation (2), which is a function of both temperature and pressure. Table 8 gives corresponding values for the temperatures and pressures used in table 7.
Table 8. Values of the ratio (n2(Tref, p) − 1)/(n2(T, p) − 1) at the working temperatures and pressures listed in in table 7.
p(kPa)/T(K) | 24.5561 | 13.80443 | 5.00025 |
---|---|---|---|
30 | 1 | 0.560286(9) | 0.193668(4) |
60 | 1 | 0.558465(7) | 0.182913(9) |
90 | 1 | 0.556641(8) | 0.171007(2) |
120 | 1 | 0.554841(8) | 0.157442(56) |
5.2. Estimation of TSPRIGT –T90,CAS
The thermodynamic temperature TSPRIGT and its standard uncertainty u(TSPRIGT) of the working gas in the resonator cavity are determined on each isobar by averaging microwave frequency measurements from three modes (TM11, TE11 and TE13). Using three calibrated rhodium-iron resistance thermometers, ITS-90 was established locally to provide temperatures T90,CAS, as described in section 3. Using these values the differences TSPRIGT –T90,CAS were evaluated for each isobar. Figure 33 shows the results. Data from the four isobars are in good agreement for the whole temperature range studied, differences never exceed 270 µK and are always less than their standard uncertainties. The fact that data at different pressures are so consistent provides strong evidence to suggest that the hydrostatic-pressure corrections were correctly estimated. The increased scatter of TSPRIGT –T90,CAS data at 5 K and the greater uncertainty at 120 kPa at this temperature are most likely due to the non-ideal behavior of 4He.
Figure 33. Values of (TSPRIGT –T90,CAS) along four isobars together with standard uncertainties. TSPRIGT is the weighted average calculated using the covariance matrix (26) in Moldover et al [65].
Download figure:
Standard image High-resolution imageTable 9 presents TSPRIGT –T90,CAS evaluations performed with the SPRIGT system at several temperatures between 5 K and 24.5561 K. We notice that the uncertainty of TSPRIGT is much smaller than that of T90,CAS in this range.
Table 9. TSPRIGT –T90,CAS in the present work.
TSPRIGT | u(TSPRIGT) | T90,CAS | u(T90,CAS) | TSPRIGT –T90,CAS | u(TSPRIGT –T90,CAS) |
---|---|---|---|---|---|
(K) | (mK) | (K) | (mK) | (mK) | (mK) |
24.55559 | 0.16 | 24.55570 | 0.21 | −0.11 | 0.26 |
23.00046 | 0.15 | 23.00046 | 0.20 | 0.00 | 0.25 |
21.99992 | 0.13 | 21.99978 | 0.18 | 0.14 | 0.22 |
20.99846 | 0.12 | 20.99813 | 0.16 | 0.33 | 0.20 |
20.26806 | 0.11 | 20.26762 | 0.16 | 0.44 | 0.19 |
18.99887 | 0.10 | 18.99817 | 0.18 | 0.70 | 0.21 |
17.99990 | 0.09 | 17.99907 | 0.20 | 0.83 | 0.22 |
17.03400 | 0.09 | 17.03310 | 0.20 | 0.90 | 0.22 |
16.00022 | 0.08 | 15.99931 | 0.20 | 0.91 | 0.22 |
15.00070 | 0.07 | 14.99986 | 0.18 | 0.84 | 0.19 |
13.80435 | 0.07 | 13.80366 | 0.14 | 0.69 | 0.16 |
12.00058 | 0.06 | 12.00015 | 0.12 | 0.43 | 0.13 |
10.00098 | 0.05 | 10.00070 | 0.12 | 0.28 | 0.13 |
8.00100 | 0.05 | 8.00067 | 0.10 | 0.33 | 0.11 |
7.00028 | 0.05 | 6.99989 | 0.09 | 0.39 | 0.10 |
6.00008 | 0.05 | 5.99966 | 0.10 | 0.42 | 0.11 |
5.00023 | 0.06 | 4.99988 | 0.08 | 0.35 | 0.10 |
Finally, figure 34 shows the weighted mean of T–T90 derived from measurements performed at the four different pressures. Vertical lines correspond to the k= 1 standard uncertainty for each temperature. SPRIGT data are compared with the world average value of T–T90 estimated by Fischer et al [4]. With the exception of points near 10 K, 12 K, 22 K, 23 K and 24.5 K, values of TSPRIGT –T90,CAS always lie above those of the current world average value, the deviation being most noticeable for the range 13.8 K to 19 K. Nevertheless, the difference between the values of T–T90 from the current work and the estimate of Fischer et al [4] is always less than 0.5 mK, which was the spread of results in the key comparison of ITS-90 CCT-K1 [8].
Figure 34. Weighted mean of (T–T90) and comparison with the most recently available world average values (grey lines) calculated using the equation developed by Fischer et al [4] and recent results obtained by AGT [53], DCGT [66] and RIGT [67] in the same temperature range.
Download figure:
Standard image High-resolution image6. Conclusion and perspectives
On 20 May 2019, World Metrology Day, the General Conference on Weights and Measures issued a major revision of the International System of Units (SI), changing the world's definition of the kilogram, the ampere and the kelvin. Henceforth, all SI units are defined in terms of constants that describe the natural world. The kelvin is now defined in terms of a fixed value of the Boltzmann constant k and a new 'Mise en pratique for the definition of the kelvin in the SI' (MeP-K) has been drawn up. MeP-K [68] recommends certain primary thermometry methods be used to disseminate the new kelvin, among them is Single-Pressure Refractive-Index Gas Thermometry (SPRIGT) [1, 14].
In this article, we have described how SPRIGT has been implemented for the first time in the range 5 K to 24.5561 K using 4He as the thermometric gas at pressures from 30 kPa to 120 kPa. The lower limit is imposed by the fact that 4He liquefies at temperatures between 3 K and 4 K at such pressures so behaves increasingly less as an ideal gas. The purpose of the work was twofold: first, to demonstrate the accuracy of the technique to measure the thermodynamic temperature T, secondly, to determine thereby the deviation between T and T90 (the International Temperature Scale of 1990 (ITS-90) [2]). The latter is based on a series of fixed points, most of which correspond to phase transitions of pure substances, hence the use of the neon triple point as the upper temperature in the present study. The highest temperature fixed point of ITS-90 is the freezing point of copper (1084.62 °C). In thermodynamic temperature measurements by optical pyrometry, at temperatures above this value, T is determined by extrapolation using the Planck radiation law [69]. Similarly, in the work described here, extrapolation is performed to determine thermodynamic temperatures below the neon fixed point, the physical basis being provided in this instance by the electromagnetic properties of helium gas. Thanks to the remarkable advances in ab initio atomic structure calculations, the latter are now calculable with accuracies surpassing those of experiment, with potential room for improvement.
In the present work, the thermodynamic temperature was determined at 17 points with uncertainties from 63 µK near 5 K to 164 µK near 24.5561 K. Such uncertainties are competitive with those of acoustic gas thermometry (AGT) in this temperature region. More importantly, compared with AGT, the increased robustness stems from the fact that no microphone is needed and the resonator is no longer subjected to acoustic vibration. Simple, rapid refractive-index measurements are possible because one need only compare four microwave resonance frequencies at two temperatures (reference and unknown) at a single common pressure and under vacuum. This alleviates the need for absolute pressure measurements and attenuates the uncertainty associated with the isothermal compressibility of the resonator. While measurements here were performed on four isobars to characterize the apparatus, in the light of this work, the optimum single pressure for the temperature range 5 K to 24.5561 K would appear to lie between 30 kPa and 45 kPa.
To determine T–T90, a local temperature scale was established (at TIPC-CAS near Beijing) using three rhodium-iron resistance thermometers (RIRT), two previously calibrated at NPL (in 1975 and 2018), the other at NIST (2015). The thermometers were manufactured by two different companies, one of which (that for NPL) has since ceased production. During temperature cycles from 5 K to 300 K and back, a repeatability of 10 µK was observed for all three thermometers, a most encouraging result that demonstrates the high quality of the more recently manufactured RIRT with regard to the conservation of T90. Given the implementation of T90 in the work described here was based on the use of only three RIRTs, an obvious extension of the present study will be to establish a reference scale using a larger number of thermometers to place it on a firmer footing. Work to this end is currently underway.
As far as the uncertainties of measurements of thermodynamic temperature are concerned, we believe there is some scope for improvement. There remains a question mark as to the origin of the unexpected pressure dependence of the excess half-widths of microwave resonances. At the same time, ongoing progress in ab initio calculations of thermodynamic properties of helium worldwide will gradually lead to a reduction in the uncertainty arising from the density and dielectric virial coefficient which dominates at the lowest temperature. While such an improvement would enable accurate primary thermometry at temperatures even lower than 5 K, to extend the temperature range down to 2 K, 3He must be used instead.
Extrapolation to higher temperatures i.e. above the neon triple point is less appealing, however, at least with a copper resonator, due to the increase of the linear thermal expansion coefficient in this temperature domain. Materials for quasi-spherical resonators combining mechanical hardness and high electrical conductivity would be highly desirable in this respect.
In view of all the results presented in this article, we recommend that Single-Pressure Refractive-Index Gas Thermometry be used henceforth in place of ITS-90 for the temperature range 5 K-24.5561 K.
Acknowledgments
This work was supported by the National Key Research and Development Program of China (Grant No. 2016YFE0204200), the National Natural Science Foundation of China (Grant No. 51627809), the International Partnership Program of the Chinese Academy of Sciences (Grant No. 1A1111KYSB20160017) and the European Metrology Research Program (EMRP) Joint Research Project 18SIB02 'Real K'. The work was also supported by the project '15SIB02 InK 2' which has received funding from the EMPIR programme co-financed by the Participating States and from the European Union's Horizon 2020 research and innovation programme. Laurent Pitre was supported by funding provided by the CAS President's International Fellowship Initiative (No. 2019VEA0015)
The authors are grateful for the expert technical assistance of Zhen Zhang and Ying Ma. We acknowledge helpful discussions with colleagues in our own institute and elsewhere, especially, R F Berg, G Bonnier, C Gaiser, R M Gavioso, K Gillis, E F May and R Underwood. In addition, these results would not have been obtained without the constant support of R Rusby and M R Moldover.
Footnotes
- 1
sccm = standard cubic centimetres per minute, corresponding to 1.6667 × 10–8 m3 s–1 in the International System of Units (SI). We define the volumetric flow 1 sccm as the flow of 1 cubic centimetre per minute of argon at a pressure 103 kPa and temperature 20 °C.