Abstract
Random lasers (RLs) rely on obtaining laser emission in disordered systems with gain. The characterization of the RLs are performed by the observations of bandwidth narrowing, increasing in the slope efficiency, time shortening of the upper level of the RL transition, intensity and spectral fluctuations, and even photon statistics transitions when increasing the optical gain. However, the gain clamping, which is a known phenomenon in conventional lasers, i.e. lasers with well-defined cavity, was never investigated in RLs. Here we realize an experimental-theoretical investigation of the gain clamping in RLs, and demonstrate that it can be used as an alternative tool to characterize the transition from spontaneous emission to the RL regime.
Export citation and abstract BibTeX RIS
1. Introduction
Random lasers (RLs) are based on the propagation of light in scattering materials with gain [1–4]. Due to the absence of a well-defined optical cavity, which usually is composed of a couple of mirrors, the RL emission was considered an amplification of the spontaneous emission rather than a true laser in the sense of oscillations [5]. One definitely demonstration of oscillations was provided by the photon statistics, in which the photon number distribution changes from Bose–Einstein to Poisson [6] as the gain is increased. Actually, the RLs are characterized by the increasing of the input–output slope efficiency, bandwidth narrowing, and shortening of the decay time of the upper level of the RL transition [7, 8]. Furthermore, the RL threshold can be found by statistical mechanics analyses [9]. Upon identical experimental realizations, the output spectra of the RLs can change dramatically from one experimental to another [10–14]. For optical gain below the threshold, the probability distribution for the output intensity is Gaussian. At the onset of laser emission the distribution can present Lévy statistics, which can be used to characterize the RL threshold [15]. However, it seems that it is not a universal feature, since some RLs (those for larger scattering strength) do not present this feature [14]. In another interdisciplinary study, there is an analogy between the optical modes of the RLs and the spins of magnetic systems [16, 17]. In the experimental realization, instead of looking only to the output intensity, the full spectra of the RL transition are analyzed [18]. By defining a correlation function (q) among the spectra obtained under the same starting conditions (replicas), the shape of the probability distribution of q is the analogue of the Edward–Anderson parameter of the spin-glass theory, and it has been experimentally observed a phase transition from a photonic-paramagnetic to a photonic spin-glass phase at the onset of the RL emission [18–20]. This parameter has been demonstrated to be robust over the different RL architectures [21–23]. Although there are many ways to characterize the RL action, as mentioned recently by Sapienza [24], the phenomenon called 'gain clamping', which is known for conventional lasers, remains unexplored for RLs. The gain clamping is the reduction of the population inversion (and the gain) to the threshold value due to the increase of the lasing field.
Here we investigate the gain clamping in different kinds of RLs. The systems analyzed here present distinct features such as lifetime of the upper level of the RL transition and quantum efficiency. Despite of that, the gain clamping was observed in both systems, and the aspects are discussed based on a rate equation model for the atomic 5 population, the energy density at the RL transition and a fluorescence channel.
2. Theoretical considerations and numerical results
In order to make clear what the gain clamping is, consider a four-level laser system in which the optical excitation is resonant with the ground-state transition |0> → |3> (see inset of figure 1). Consider also that the state |2> is so close to the state |3> that very fast nonradiative relaxations from |3> populate the state |2> (relaxation time τ3 ≈ 10 ns). Taking |2> as a metastable state (total lifetime τ2 ≈ 20 µs—including both radiative and nonradiative transitions), and considering fast nonradiative relaxation from |1> to |0> (τ1 ≈ 10 ns), it is known that this system can work very well for laser emission. The following rate equation system can cope with the system dynamics, where n0, n1, n2, and n3 are the population densities of the nondegenerate states |0>, |1>, |2>, and |3>, respectively, and the dot above nj represents time derivative
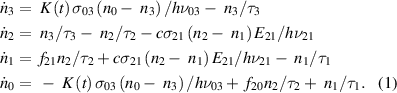
Figure 1. Inset: simplified energy level diagram describing the operation of a four-level laser. The resonant ground-state excitation promotes the ions from the state |0> to |3>, which populates the metastable state |2> by fast nonradiative relaxations. From the state |2> one has the random laser (RL) transition (|2> → |1>) and a fluorescence emission transition (|2> → |0>). The figure shows the simulated output energies for the transitions |2> → |1> (E21) and |2> → |0> (E20). While the former presents an increasing in the slope efficiency, the last presents a saturation, which is attributed to the gain clamping.
Download figure:
Standard image High-resolution imageIn these equations, K(t) is the excitation power density (∫K(t)dt gives the excitation pulse energy (EPE) density), σ03 the resonant absorption cross-section at the excitation wavelength, σ21 the stimulated emission cross-section at the RL transition, c the speed of light, and hν03 and hν21 are the photon energy at the absorption and lasing wavelengths, respectively. We considered relaxations (radiative and nonradiative) from the state |2> with fractions f21 and f20 ending at levels |1> and |0>, respectively. Then, we introduced equations for the energy density (E21) at the RL transition, and for the fluorescence (E20) at the transition |2> → |0>:

where β (0 < β ⩽ 1) is a dimensionless parameter introduced to consider that an ion relaxing at the transition |2> → |1> does not necessarily create a photon at the random medium (for example due to nonradiative relaxations), and τ21 is the relaxation time of the photons out of the random medium, which in a conventional laser is linked to the mirrors reflectivity [25]. This approach is based on that introduced by Noginov et al for RLs [26], which has been describing quite well the experimental observations [27] including parametric effects [28, 29]. In their approach, τ21 is related to the dwell time (≈10 ps) of photons in the disordered materials. The main difference from here to [26] is that we did not simplify the equations for the ionic densities to only one equation for the upper level of the RL transition (|2>), and we introduced an equation for the spontaneous emission (E20) due to other transition (|2> → |0>) starting from same level (|2>) of the RL transition. For simplicity, we considered the same relaxation time for E21 (τ21 = τ20), but neglected any possibility of stimulated emission at this transition, which assure only fluorescence at the transition |2> → |0>. We considered typical parameters for the Nd3+ ions [26]: σ03 = 3 × 10−21 cm2; σ21 = 1 × 10−18 cm2; n0 + n1 + n2+ n3 = 5 × 1021 cm−3; hν03 = 4 × 10−19 J; hν21 = 2 × 10−19 J; β = 0.01. The parameters f21 and f20 were fixed at 0.6 and 0.4, respectively. In most of the investigated RLs, the excitation source was due to Q-switched lasers with pulse duration of the order of ≈10 ns. In order to mimic their temporal behavior, the photon flux K(t) in equation (1) is considered a Gaussian pulse with duration of 4.1 ns. The integration of K(t) over time provides the energy density of the excitation pulse.
Most of the observed RL features are described by the model above as follows. Monitoring the output energy at the RL transition (power integrated over time) one has an increasing in the slope efficiency, which transition at a given EPE threshold (EPEth) is abrupt (figure 1). Also, by monitoring the time decay of the upper level of the RL transition (|2>) one has for an EPE lower than the threshold a single exponential decay, which is typical of fluorescence; on the other hand, crossing the EPE threshold one has the shortening of the decay time as follows. The temporal behavior of n2 is shown in figure 2(a) for three EPE. For an EPE lower than the threshold, one can see the build-up of n2 in the showed time scale. Due to the large lifetime (τ2 = 20 µs) of the level |2>, n2 decays exponentially on this time scale. Similarly, the intensities at the transitions from the |2> state to the |1> and |0> ones decay exponentially because for EPE below the threshold one has only spontaneous emission and the trapping of light is weak (τ21 = τ20 = 10 ps). On the other hand, when n2 is larger than the threshold condition (gain = losses for conventional lasers; and number of generated photons per unit of time equals the number of escaping photons of a given excited volume [1, 2]) the stimulated emission takes place (figure 2(b)) pushing the gain down to the threshold (indicated in figure 2(a) by a horizontal dashed line)—that is the gain clamping. At the onset of RL emission, one has the emergence of a short pulse with duration of ≈400 ps, which coincides with the decrease of the gain due to the stimulated emission (figure 2(b)). Under gain above threshold, n2 relaxes mainly by stimulated emission at the RL transition. Thus, the stimulated emission inhibits the other transition from the level |2> to level |0> as well as the nonradiative relaxations which do not create photons at the random medium (accounted by the parameter β). As a consequence, the intensity of the emission due to the transition |2> → |1> increases abruptly (figure 1). Increasing the EPE further the threshold, one has a competition between the stimulated emission to decrease the gain, and the excitation pulse to increase the gain. As a consequence, the time behavior of n2 presents some oscillations (figure 2(a)), and the RL intensity possess several short peaks, which are known as relaxation oscillations (figure 2(b)). Since the fluorescence at the transition |2> → |0> depends on n2, it would also show the same oscillations (figure 2(c)). But, usually, the intensity of the fluorescence is so weak that is not measured experimentally (in order to resolve it experimentally, fast detection systems are required which work well for large intensities). At the end of the excitation pulse the n2 reaches back the threshold (figure 2(a)) and the system relaxes as if there were no stimulated emission. Then, the fluorescence (energy) at the transition |2> → |0> presents a saturation behavior (figure 1), which is an evidence of the gain clamping.
Figure 2. Temporal dynamics of a four-level random laser (represented in the inset of figure 1) under pulsed excitation (ns) for the three excitation pulse energies (EPEs): lower (<), slightly larger (≈) and larger (>) than the EPE threshold (EPEth). (a) Population (n2) of the upper level of the laser transition (|2>). The horizontal dashed line indicates the threshold population for laser action. (b) Normalized output intensity at the RL transition (|2> → |1>). The Gaussian curve (red) corresponds to the temporal profile of the excitation pulse. (c) Output intensity at the transition |2> → |0> corresponding to the fluorescence. The vertical dashed line indicates the coincidence of the emergence of the RL short pulse (figure 2(b)) with the point where n2 starts to decrease due to the stimulated emission—gain clamping.
Download figure:
Standard image High-resolution imageAnother evidence of RL emission, not coped with the model above, is the bandwidth narrowing, i.e. increasing the gain, the RL spectra narrow due to the exponential amplification of the gain curve. For homogeneous broadening lasers, the gain presents a Lorentzian dependence with the light frequency (due to the finite lifetime), and it collapses to a single frequency at an EPE larger than the threshold. For nonhomogeneous broadening, one can have more than one lasing wavelength [30, 31]. For example, due to aggregates formation in dyes [30] or non-equivalent site symmetries for Nd3+ ions in crystalline structure [31].
At this point, it worth emphasizing the gain clamping is one mechanism of gain saturation. The clamping is a tendency of the stimulated emission to push down the gain towards the threshold because the growing of the optical field increases the stimulated emission rate [32]. On the other hand, one can have gain saturation by population redistribution among the energy levels [33]. For example, in the rate equation system (equation (1)), if one consider a large time (τ1) of the lower level of the laser transition (|1>), as the ions at the level |2> relax, one has accumulation at the lower level, which decreases the population inversion (n2 − n1), a requirement for amplification. In the following, we present the experimental results concerning the gain clamping in RLs.
3. Experimental results and discussion
3.1. Experimental setup
The experimental setup consisted in excite the samples using the second-harmonic (532 nm) of a Q-switch Nd:YAG laser operating at 1064 nm with pulse duration of ≈10 ns and spatial profile close to the Transverse Eletromagnetic Mode 00 (TEM00). A pair of polarizers were used to control the EPE, while keeping the fluctuation of the excitation laser the same for all investigated EPE [25]. The excitation light was focused onto the samples by a 5 cm focal length lens at an incidence angle of 20° with respect to the normal to the sample surface. It provided an excited area of 0.053 mm2. The generated light was collected at the normal direction to the sample surface with a pair of lenses, collimating the light and focusing onto a multimode optical fiber coupled to a spectrometer equipped with a charge-coupled device camera, which allowed single-shot measurements from 350 to 1100 nm with resolution of 2.0 nm. The frequency of the excitation laser was fixed at 5 Hz. Thus, the time between consecutive shots is much larger than the time for relaxations of the Nd3+ (≈100 µs), and rhodamine 6G molecules (≈10 ns). Under these circumstances, for a given EPE, each shot is considered an identical experimental realization under fairly the same starting conditions.
The samples used in this work were: (a) powders of Nd0.60Y0.40Al3(BO3)4 nanocrystals (broad particle size distribution between 30 nm and 600 nm with peak at ≈100 nm) gently pressed in a sample holder—see [34] for the synthesis, morphological and structural characterization; (b) a poly(methyl methacrylate) (PMMA) film (thickness = 1 mm) with 5 mM of rhodamine 6G, and titanium dioxide (TiO2) nanoparticles (average size distribution 250 nm) at 2500 ppm mass concentration [35].
3.2. Experimental results for the Nd0.60Y0.40Al3(BO3)4 nanocrystals
Solid-state RLs based on Nd3+ ions have been investigated [7] since the first experimental evidences in 1986 [3], and one promising application is in near-infrared biomedical imaging systems [36]. Here, the excitation at 532 nm (ground-state absorption 4I9/2 → 4G7/2) of Nd0.6Y0.40Al3(BO3)4 provided down-conversion fluorescence around 885 nm, corresponding to the Nd3+ electronic transition 4F3/2 → 4I9/2, and RL emission at around 1064 nm due to the 4F3/2 → 4I11/2 transition—see [37, 38] for detailed description of the excitation scheme. This excitation route can be simplified to a four-level system, in which the states 4I9/2, 4I11/2, 4F3/2 and 4G7/2 are represented by the |0>, |1>, |2> and |3>, respectively, in the inset of figure 1. Other down-conversion emissions are expected at wavelengths lower than 885 nm and large than 1100 nm. For example, at 1320 nm due to the Nd3+ transition 4F3/2 → 4I13/2. It is also possible to observe up-conversion emissions, in which the energy of the generated photons are larger than the excitation ones [39]. However, those emissions are out of the scope of the present letter. Figure 3 shows single-shot spectra of the excitation laser at 532 nm, the fluorescence (around 885 nm), and the RL transition (around 1064 nm) for three different EPEs. For each EPE, four independent experimental realizations are displayed.
Figure 3. Results corresponding to the excitation of the Nd0.60Y0.40Al3(BO3)4 powder. Four single-shot spectra from the excitation laser around 532 nm, fluorescence around 885 nm (Nd3+ transition 4F3/2 → 4I9/2), and random laser (RL) emission around 1064 nm (Nd3+ transition 4F3/2 → 4I11/2). The excitation energy 2.52 mJ (first row) is below; 4.13 mJ (middle row) is around; and 5.75 mJ (third row) is above the RL threshold.
Download figure:
Standard image High-resolution imageThe output fluctuation analyses constitute a current trend in the research of lasers/RLs [12, 15, 18, 25, 40, 41]. One can see in figure 3 that the excitation laser is unstable from shot-to-shot. That characteristic is intrinsic for some lasers, and is attributed to gain competition and nonlinear interaction among the optical modes [25, 41]. For an EPE below the onset of RL emission (2.52 mJ), the fluctuation of the fluorescence and the emission at around 1064 nm is small and correlated with the excitation pulse (first row of figure 3). On the other hand, for an EPE (5.75 mJ) larger than a given threshold (≈4.0 mJ—discussed below), one can notice the narrowing of the spectra around 1064 nm (second row of figure 3), which is an evidence of RL emission. Notice also that the fluorescence around 885 nm is quite stable, while the RL presents strong and uncorrelated fluctuations with the excitation laser. As in a conventional laser, the fluctuation of the RL spectra is associated with the gain competition and nonlinear interaction among the optical modes [14, 16]. On the other hand, the fluctuation of the fluorescence is small for gain above threshold because of the gain clamping [32, 42]. According to figure 1 the slope efficiency of the fluorescence for gain above the threshold is close to zero, which implies that the fluctuation of the excitation energy would provide almost zero fluctuation for the fluorescence at the transition |2> → |0> (4F3/2 → 4I9/2 for the Nd3+). For large EPE the fluctuation of the RL shows some correlation with the excitation laser (third row), which we associate to a self-averaging mechanism in the competition of the optical modes from the available gain [14]. Our observations about the correlations between the RL and the excitation laser are in agreement with the work of Zhu et al [13], where the authors performed a detailed study about those correlations.
The input–output energy characterization of the Nd3+ based RL is presented in figure 4. Increasing the EPE, one has the abrupt increase of the slope efficiency, which defines the threshold (≈4.0 mJ). On the other hand, the fluorescence around 885 nm presents a decrease in the slope efficiency, which evidences the gain clamping. Notice the good agreement between the experimental results of figure 4 and the theoretical ones in figure 1. It is worth emphasizing that the experimental results display a small growth of the fluorescence for gain above threshold, which is attributed to the fact that only a fraction of the excited volume participates from the RL. It is assigned to the spatial profile of the excitation laser, which is close to a Gaussian (TEM00), as well as the diffusion of excitation photons, which increases the excited volume. As a consequence, the RL takes place near the center of the excitation beam, which implies that the excited volume out of the center is not subject to the gain clamping, and thus presents a linear contribution to the fluorescence at 885 nm as the EPE is increased further the RL threshold. Similar evidences were found by Noginov et al [26] by monitoring the fluorescence at 1054.4 nm (due to Stark levels transitions between the 4F3/2 and 4I11/2 manifolds) close to the RL emission at 1063.1 nm in NdAl3(BO3)4 powders. The authors observed the saturation behavior without mentioning the clamping as the responsible mechanism.
Figure 4. Intensity of spontaneous emission (885 nm) and stimulated emission (1064 nm) for Nd0.60Y0.40Al3(BO3)4 nanoparticles. The transition from the spontaneous emission to the random laser regime is evident by the increasing of the slope efficiency at the excitation pulse energy threshold. On the other hand, the spontaneous emission behavior presents a saturation behavior above the threshold, which is an indication of gain clamping.
Download figure:
Standard image High-resolution image3.3. Experimental results for rhodamine 6G and TiO2 nanoparticles in PMMA
Due to the large quantum yields of laser dyes, RLs based on them are among the most investigated. Several architectures have been demonstrated to support dye based RLs. For example, colloidal solutions of laser dyes and TiO2 nanoparticles [4, 20], porous systems infiltrated with laser dyes [43, 44], hollow-core photonic optical fibers infiltrated with dye and TiO2 nanoparticles [45], biological tissues [46–49], and exotic materials as butterfly [50] and cicada wings [51]. In this section, we investigate the gain clamping in a RL based on a PMMA film with rhodamine 6G molecules, and TiO2 nanoparticles to provide the scattering. The excitation of rhodamine 6G molecules at 532 nm provides singlet-singlet (S0 → S1) absorption to some energy level (|3>) within the S1 band, which, followed by nonradiative relaxations, reach the bottom of the excited S1 band (level |2>). From that, one has relaxations to the S0 manifold, and the RL transition ends in a level (|1>) above the ground state (|0>). Because of that, the four-level model presented above can be used for the rhodamine 6G based RL.
The excitation of the PMMA sample with rhodamine 6G and TiO2 nanoparticles was accompanied with the bandwidth narrowing and increasing in the slope efficiency for the RL peak. Three emission spectra are shown in figure 5(a) for different EPEs. For low EPE the spectrum is broad (from ≈560 nm to ≈700 nm). Increasing the EPE one can notice the bandwidth narrowing and the emergence of a short peak (≈7 nm), which is characteristic of dye based RLs [4, 20, 45]. In contrast with the Nd3+ case, here there is just one emission band, i.e. other fluorescence band due to other relaxation transition from the same starting level is absent. Because of that, to compare the RL (600 nm) with the fluorescence in order to analyze the gain clamping, we select the intensity at 585 nm, which is close to the RL peak (600 nm), and thus expected to be clamped by the RL transition. To avoid any hidden contribution to the fluorescence analyses from molecular aggregates and reabsorption/reemission effects, which are known to redshift the RL wavelength [30, 52, 53], we selected the fluorescent emission at 585 nm whose wavelength is shorter than the RL peak.
Figure 5. Characterization of the rhodamine 6G based random laser (RL). (a) Photoluminescence spectra under pulsed excitation at 532 nm for excitation pulse energies (EPEs): lower (0.12 mJ), slightly larger (0.19 mJ) and larger (0.94 mJ) than the EPE threshold (EPEth ≈ 0.16 mJ). The spectral narrowing when increasing the EPE is an evidence of RL emission. (b) Intensities at 585 nm (fluorescence) and 600 nm (RL) as a function of the excitation pulse energy. The saturation behavior for the fluorescence is an evidence of the gain clamping, while the increasing of the slope efficiency for the emission at 600 nm is an evidence of the transition from the fluorescent to the RL regime. Inset: simulated input–output energy considering the lifetime of the |2> equals 4 ns, and β = 0.3.
Download figure:
Standard image High-resolution imageThe input–output energies of the RL (600 nm) and the fluorescence (585 nm) as a function of the EPE present distinct features (figure 5(b)). The slope efficiency for the RL increased at a given threshold (≈0.16 mJ), while the fluorescence exhibited a saturation behavior, which we associate with the gain clamping. In contrast with the Nd3+ behavior, the increasing in the slope efficiency and the gain clamping are not pronounced. We attributed those differences to the large quantum yield and short lifetime of the rhodamine 6G molecules. In fact the simulation of the rate equation model by changing only the parameters β and τ2 to 0.3 and 4 ns, respectively, provides the results in the inset of figure 5(b), which are similar to the experimental ones in figure 5(b). β influences the increasing in the slope efficiency, since the stimulated emission inhibits nonradiative relaxations from the upper level of the RL transition [27]. The nonradiative relaxations are considerable for the Nd0.60Y0.40Al3(BO3)4 nanocrystals due to the low energy gap between the 4F3/5 and 4I15/2 states [54]. On the other hand, the rhodamine 6G presents only one main electronic transition (S1 → S0) whose energy gap is high. Because of that, β is considerably larger for the rhodamine 6G. Consequently, the increase of the slope efficiency is smooth for the rhodamine 6G and abrupt for the Nd3+ based RL. Regarding the differences in the gain clamping behavior, for the rhodamine 6G the clamping is less evident because the spontaneous emission decay rate (n2/τ2) is on the order of the stimulated emission one [cσ21(n2− n1)E21/hν21]. Increasing τ2 in the simulations, we observed a bending of the fluorescence intensity curve towards a horizontal line, as in figure 1. In spite of subtle change in the fluorescence emission for the rhodamine 6G based RL as the gain increases, the gain clamping, which concerns the present work, is observed and can be used as a tool to characterize the RL.
4. Conclusions
We investigated the gain clamping in two different RLs: the first, based on Nd3+ ions in crystalline nanoparticles [YAl3(BO3)4]; and the second based on rhodamine 6G molecules and titanium dioxide (TiO2) nanoparticles as scatterers. Despite these systems present distinct features such as quantum yields and fluorescence lifetimes, the gain clamping was observed in both, and corroborated with numerical simulations based on a rate equation system for the atomic populations, and the energies at the RL and a fluorescence emission, whose transitions departure from the same level. In both systems, at the onset of the RL emission, the fluorescence demonstrated a saturation behavior, evidencing the gain clamping. Because of that saturation behavior, the fluorescence presents low fluctuation for EPE larger than the threshold, on the other hand of the RL emission, which shows large fluctuations. The fluctuation of the RL emission, especially at the vicinity of the EPE threshold, is associated with mode competition for the available gain and nonlinear interaction among them, which has been investigated within the framework of the statistical mechanics of complex systems [11, 16, 18, 19, 25, 40]. Then, the gain clamping can be used not only to determine the RL threshold, but to support the discussion about the output fluctuations in RLs.
Acknowledgments
We acknowledge the financial support from the Brazilian agencies: Fundação de Amparo à Pesquisa do Estado de Alagoas (FAPEAL); Coordenação de Aperfeiçoamento de Pessoal de Nivel Superior (CAPES)—Finance Code 001, Fundação de Amparo à Pesquisa do Estado de Goiás (FAPEG), Financiadora de Estudos e Projetos (FINEP), Conselho Nacional de Desenvolvimento Científico e Tecnológico (CNPq) through the Grant No. 427606/2016-0; scholarships in Research Productivity 2 under the Nos. 303990/2019-8 and 305277/2017-0; National Institute of Photonics (INCT de Fotônica). C V T M and R F S thank CNPq for their Scientific Initiation scholarship. We thank to J G B Cavalcante, and M A Nascimento for technical support.
Footnotes
- 5
In more general terms, we call atomic population. But, it can refer to ions or molecules.