Abstract
The influence of optical centers concentration profiles on thermal fields under high-power end-face diode pumping is analyzed using numerical model. It is shown that non-uniform distribution profiles of optical centers concentration are very promising for high average pump power laser amplifiers. The possibility of creating concentration profiles of optical centers in gradient-activated crystals with YAG matrix with a specified distribution along the growth axis of the crystal is demonstrated. A technology of growing gradient-activated single crystal involving active mirror as active element is proposed and discussed.
Export citation and abstract BibTeX RIS
1. Introduction
Much of contemporary research in the field of laser physics is aimed at solving problems connected with the development of the element base for high-efficiency lasers generating extreme light fields [1]. Obtaining pulses of subpetawatt and petawatt level will make it possible to carry out some fundamental interdisciplinary research, such as the problems of extreme light fields interactions with matter, high-order harmonics generation, attosecond pulse generation, and laser acceleration of charged particles [2–5]. The creation of such laser systems is limited by such factors as thermally induced distortions of the radiation and self-focusing [6, 7]. With output and pulse frequency of a solid state laser increasing simultaneously, negative thermal effects develop which is the key factor in decreasing the efficiency of laser amplification. The conventional way to amplify the optical radiation of solid state YAG:Yb or YAG:Nd laser seed pulse involves increasing the concentration of optical centers. Yet this approach results in a reduction of the efficiency of longitudinal optical pumping because of cross-relaxation and reabsorption of laser radiation, accompanied by a temperature rise which cannot be compensated, especially in the case of multilayer cooling systems [8].
Modern research on these problems lies in two directions. The first direction is developing a technology that would allow obtaining optical material with non-uniform distribution of optical centers in an optically active medium in order to remove thermo-optic effects [9–13].
The second direction in research of high power systems is the development of amplification cascades using active mirrors which are thin YAG:Nd, YAG:Yb based single-crystal discs as active elements with an antireflection coating of one side and high-reflection coating on the other [14–16].
It should be noted that studies and analysis of Nd3+ and Yb3+ ions show that Yb3+ ion is much more suitable for high-efficiency diode pumping with high average power. First, due to the simple level structure characteristic of Yb3+ ion, in laser media using this ion, most of the negative effects (upconversion, absorption from the excited state, etc), inherent in media with Nd3+ are not observed. Secondly, the Yb3+ ion is characterized by a much lower value of quantum defect (about 5%) compared to the Nd3+ ion (about 30%). Together, these properties lead to a much smaller impact of thermal effects in the case of the Yb3+ ion, which is critical for solid-state laser systems with high peak and average power.
Significant thermal load on the active element is an important feature of systems with high pulse repetition rate since diode lasers with high average power are used for pumping, which leads to significant heating and, consequently, to a decrease in the laser characteristics of the medium and to phase aberrations in the output amplifier cascades [17].
For cryogenically cooled systems another problem arises: significant temperature gradients lead to wavefront distortions in amplified radiation. In the temperature range 80–300 K in YAG:Yb elements, for example, the radiation cross-section at the radiation wavelength (1030 nm) increases by ∼10 times, the absorption cross-section at the wavelength of pump radiation increases by ∼2 times, thermal conductivity—by 5–10 times depending on the degree of doping, and the heat capacity—by ∼5 times [16].
In terms of modeling, multiple changes in the parameters of YAG:Yb elements during the transition from cryogenic to room temperatures determine the nonlinear nature of the dependence of the gain on the pump radiation and the cooling system.
This paper is dedicated to modeling of active media with nonlinear distribution of ions aiming to reduce the temperature gradients and the average value of temperature in the active elements of cryogenically cooled laser amplifiers with high average pump power [10, 18].
2. Numerical model
A few-cycle high-intensity laser radiation source operating with a high pulse repetition rate with stabilization of pulse carrier-envelope offset (CEO) is developed. The source includes a Ti:Sa-based system [19] and a scalable subjoule-class Yb3+ media-based laser system [1]. Ti:Sa-based system generates CEO-stabilized pulses and Yb3+ media-based system produces high energy and high peak power pulses. Both systems operate at high pulse repetition rate and are used to provide signal and pump for parametric amplification, aimed at CEO-stabilized, high energy, high peak power pulses with high repetition rate. The scalable subjoule-class Yb3+ system consists of a Yb:Y2O3 master oscillator at cryogenic temperatures, a grating stretcher, an Yb:KYW regenerative amplifier, water cooled and cryogenically cooled multipass laser amplifiers [8, 20], and a nonlinear optical crystal for frequency doubling.
A two-stage cryogenically cooled multi-disk laser amplifier with powerful diode pumping is located at the output of the Yb3+ system [1]. The temperature difference between the cooled and the pumped sides of the 3.5 mm thick disk element in this amplifier can reach ΔT ∼150 K [8].
A numerical three-dimensional non-stationary model was developed, which includes balance equations and the thermal conductivity equation [17]. For high pulse repetition rates and continuous wave mode, the model could be simplified due to heat distribution is not changing significantly pulse to pulse. Also, the dependency of cryogenic cooling system heat removal capacity on pump power and the dependencies of both the physical and laser properties of the active elements on temperature and concentration are accounted. In this paper, the system of equations is extended to account the non-uniform distribution of active ions:

where κ is the dopant ion concentration, cm−3; T is the active element temperature, K; x and y are transversal coordinates aligned to working surface of the disk, cm; z is the longitudinal coordinate orthogonal to XY plane, cm; t is time, s. In all equations the direction along z also corresponds to the axis of the pump radiation.

Λ, ρ and С is the thermal conductivity coefficient, W cm−1 K−1, density, kg cm−3, and the thermal capacity of the medium, J K−1 kg−1; and heat source term Q is defined as

where η is the part of the energy of the absorbed pump radiation that is turned into heat; Iр is the pump radiation intensity, W cm−2.
The boundary condition at the surface in contact with heatsink is

where T(P) reflects the experimentally measured parabolic temperature dependence of the heatsink on the average diode pump power P; mi are the approximation coefficients; l is the thickness of the disk. All other surfaces are considered perfectly isolated.
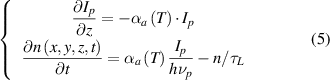
where n is the population density of the laser level, cm3; νp is the central frequency of the pump radiation, s−1; h is the Planck's constant, J s−1; τL is the luminescence lifetime, s. The absorption coefficient αa , cm−1; and the gain coefficient gL, cm−1, are, in turn, defined as


where nt is the concentration of activating ions, сm−3; σа is the absorption cross-section at the wavelength of pumping radiation, cm2; σL is the luminescence cross-section at the wavelength of radiation being amplified, cm2.
The dependency of absorption cross-section on temperature is described by expression obtained by fitting of published data [21–23]:

The luminescence cross-section is, in turn, described by the next formula also obtained by fitting of experimental data taken from [24]:

Occupation factors fji are calculated using the next formula:

where ΔEji are corresponding energies for the ith level of upper manifold and the jth level of lower manifold, J, and k is Boltzmann's constant, J К−1.
The analysis of published data [16, 25, 26] provided us with the dependence of the thermal conductivity coefficient on the temperature and the concentration of optical centers in YAG:Yb crystal:

where A, B, D, E, F, K are constant approximation coefficient. The mathematical model represented by equation (9) describes the coefficient of thermal conductivity with error not exceeding 5% compared to experimental data in the temperature range 70–330 K.
The system of balance equations is solved in combination with the heat equation, which allows taking into account both the dependences of the absorption cross-section of Yb ions at the pump radiation wavelength (936 nm) and the population of laser levels on temperature. The three-dimensional heat equation is solved by the method of spatial splitting on an uneven spatial grid and involves calculating temperature distributions in the active element and in the components of the cryogenic cooling system.
A series of experiments were carried out for the approbation of the model, however, for active elements with uniform distribution of dopant ions [8, 17]. The results are in good agreement with experimental data.
3. Modeling
The following calculations use the experimentally measured spatial and temporal distribution profiles of pump radiation, as well as the experimentally determined parameters of the closed-loop cryogenic cooling system [8].
Model distribution profiles of active ions included the following:
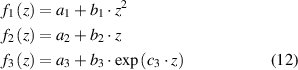
where ai, bi, ci are constant coefficients determined by the condition constant value of the absorption coefficient of pump radiation:
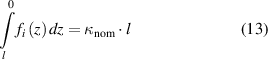
where κnom is the value of uniform concentration of activating ions to compare with. In this study κnom is equal to 5 at.%.
Equation (13) is not sufficient to determine in a unique way the values of the coefficients ai, bi, and ci, thus additional considerations required: distribution profile fi(z) must lie in 0–15 at.% range and it should grow along z. The former is required to allow one to grow such crystal with laser quality and also to keep validity of model dependencies. The latter is dictated by simple assumption that heat should be deposited as close as possible to cooled surface to decrease temperature. It should be noted that this assumption was verified by means of modeling.
For the exponential profile all conditions mentioned above defines the least possible initial value to be 2.2 at.%. While linear and parabolic profiles could start from 0 at.%, the initial value 2 at.% were used for clarity. Figure 1 features the concentration profiles used for modeling:
Figure 1. The dependency of dopant ions concentration on coordinate for different profiles.
Download figure:
Standard image High-resolution imageThe following parameters were calculated for the profiles represented in figure 1: temperature distribution along the pump radiation axis in the center of the active element (figure 2), the population density of the laser level (figure 3), gain in case of a small signal (figure 4). The simulation was carried out for the following parameters of pump radiation: peak power of 200 W, diameter of 1.5 mm at a level of 1/e2 mm, pulse duration of 500 μs, and repetition rate of 1 kHz.
Figure 2. Temperature distribution in the center of the active element along the pump radiation axis.
Download figure:
Standard image High-resolution imageFigure 3. Dependence of the population density of the upper laser level on the type of concentration profile of optical centers in YAG: Yb.
Download figure:
Standard image High-resolution imageFigure 4. Dependence of the small signal gain on the type of concentration profile of optical centers in YAG: Yb.
Download figure:
Standard image High-resolution imageAs can be seen from figure 2, both parabolic and exponential concentration profiles demonstrate a 15% decrease in the maximum temperature of active laser element as compared to the thermal distribution in YAG:Yb crystals with a constant concentration profile. Also, a region with gradually changing value is formed over ∼40% of the length of the active element, leading to a decrease of the longitudinal gradient. In this case, there is no significant difference between the exponential and the parabolic profiles.
It should be noted that the dependence of the population density n of the excited level for constant distribution profile is qualitatively different from that for other concentration profiles, providing efficient energy storing. Such energy deposition increases total small signal gain, as can be seen in figure 4.
The maximum small signal gain gL in YAG:Yb crystals exceeds the corresponding value for the constant concentration profile of optical centers Yb3+ (figure 4) by more than 2.5 times for the exponential concentration profile, and by 2 times for the parabolic profile. The single-pass overall gain for constant doping profile is 1.75, for linear 2.01, for parabolic 2.23, and for the exponential 2.33.
4. Growth experiments
A number of growth experiments aimed at obtaining gradient activated YAG crystals were carried out to confirm that it is possible to obtain such a single-crystal material with non-uniform distribution of optical (impurity) centers along the growth axis that would demonstrate the ability to remove thermo-optic effects in a similar way as segmented laser elements made of YAG:Nd optical ceramics do [27].
Previously, a new class of optical materials with functional non-uniform distribution of optical dopant were investigated [28]. It was shown that it is possible to increase optical pumping efficiency and to smooth the temperature profile in the active medium by means of non-uniform distribution of optical centers along the growth axis.
The method of growing gradient-activated crystals has been developed [29]. The technology of obtaining single crystals with controllable non-linear distribution of impurity centers is based on the Czochralski method with additional charging of the melt and involving the process of mono-crystal being pulled from the aperture in the crucible-reactor placed coaxially inside the main crucible. Strictly determined compositions of the melts Ndn :Y3–n Al5O12,Ndm :Y3–m Al5O12 (n ≠ m) are specified both for the crucible-reactor and the main crucible, and during the pulling of the crystal the crucible-reactor is being moved in relation to the main crucible with pre-calculated speed using special software.
During the growth experiments, a single crystal with the following composition was obtained:

where z is the coordinate along the direction axis of the formation of the crystal concentration profile; a, b, c, k are some constants (a = 3 × 10−6 mol.% mm−3; b = 2 × 10−4 mol.% mm−2; c = 4 × 10−4 mol.% mm−1; k = 0.7558 mol.%).
The actual concentration profile of the crystal was studied by methods of optical spectroscopy and laser emission analysis [30, 31]. The inset of figure 5 features a typical absorption spectrum within the range from 750 to 850 nm. The spectra were measured in arbitrarily chosen longitudinal coordinates of the YAG:Nd crystal.
Figure 5. Experimental confirmation of the implementation of the concentration profile of Nd3+ ions in a gradient-activated YAG:Nd crystal by optical spectroscopy.
Download figure:
Standard image High-resolution imageFigure 6 shows the value of the integrated energy absorbed by the optical centers, calculated by the formula (15), in arbitrary longitudinal coordinates of the crystal plate:
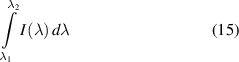
Figure 6. Changes in the concentration profile of Nd3+ ions in gradient-activated YAG:Nd crystal (crystal plate photo (a)) and the refraction index dynamics in the terahertz range.
Download figure:
Standard image High-resolution imagewhere I(λ) is the absorption spectrum; dλ—sampling interval.
Changes in the crystal composition along the growth axis may affect the dynamics of its refractive index within the terahertz range [32, 33]. The research was carried out using TeraK15 spectrometer, and absorption spectra and refractive indices were obtained for gradient-activated YAG:Nd crystal in the range from 0.1 to 3.5 THz. The markers in figure 6 show the values of the refractive index of gradient-activated YAG:Nd crystal, obtained in arbitrary longitudinal coordinates of the crystal plate. These results show the correlation between the dynamics of refractive index changes and the function of the optical centers concentration profile changes in gradient-activated crystal YAG:Nd.
Inset (a) of figure 6 features a photo of a crystal plate obtained from the boule of the gradient-activated YAG:Nd crystal with the direction of the growth axis of the mono-crystal c indicated.
Studies of the actual concentration profile by optical spectroscopy and laser emission analysis give a fairly high degree (>85%) of the reliability of the theoretically established concentration profile of Nd3+ ions in YAG crystals. Previously, a technology of growing Yb:YAG crystals with high dopant concentration gradients has been developed [34]. It essentially means that it is possible to grow an YAG-matrix element with desired concentration profile.
5. Conclusion
Based on the numerical model, the influence of a number of optical centers concentration profiles on thermal fields under high-power end-face diode pumping is analyzed. It is demonstrated that in case of using gradient-activated YAG: Yb3+ crystals with a Yb3+ concentration profile varying from 2 to 14 at.% according to the exponential or parabolic law, the maximum temperature decreases by ∼15%, and a region with a temperature gradient of ∼10% is formed along the first half of the active element.
Within the framework of the current research the dependencies of the population of the upper level and the gain in case of the small signal on the coordinate in the active element are obtained, which in non-standard cases have a qualitatively different form and are characterized by higher gain values.
The possibility of creating concentration profiles of optical centers in gradient-activated crystals with YAG matrix with a specified distribution of the optical centers concentration along the growth axis of the crystal is demonstrated. A technology of growing gradient-activated single crystal involving active mirror as active element is proposed.
Acknowledgments
Authors declare no conflict of interest. This work was funded by the Presidium of the Russian Academy of Sciences (Extreme Light Fields and Their Interaction with Matter Program, Grants Nos. AAAA-A18-118040290036-4, АААА-А18-118040290040-1) and in part by the Russian Foundation for Basic Research (RFBR) (Project No. 20-02-00529-a), RFBR and Novosibirsk oblast government (Grant No. 19-42-543007) and the RF Ministry of Science and Higher Education (Grant Nos. AAAA-A17-117030310296-7, FSUN-2020-0007, FZEN-2020-0022/20/65t-2).