Abstract
In this paper, topological quantum phase transition was reported in the magnetic topological insulator MnBi2Te4 under pressure strain. Electronic and topological properties of the bulk anti-ferromagnetic MnBi2Te4 were investigated by first-principles calculations. We found that the band structure of MnBi2Te4 changes with the strain, resulting in a phase transition between metal and insulator. From the variation of charge-density distribution with strain, it was found that hydrostatic tensile strain is beneficial for increasing the interlayer spacing, thereby reducing the anti-ferromagnetic interaction between layers. On the contrary, the compressive strain promotes the strengthening of the bonding between the Te and Bi atomic layers. It was worth noting that the phase transition occurs at 2.12% strain when the band crossing is observed at Γ point, suggesting that the band gap has just closed. In addition, through the calculation of surface states, it is observed that, after the action of 2.12% strain, the bulk band gap of the system closes with the surface band gap reopens, achieving an intrinsic mechanism of strain modulation of the MnBi2Te4 antiferromagnetic bulk structure to undergoes a topological quantum phase transition. Our results provide feasible guidance not only for pressure-strain engineering of MnBi2Te4 experimentally but also for developing a meaningful strain-control mechanism for the electronic structures of other potential intrinsic magnetic insulators.
Export citation and abstract BibTeX RIS

Original content from this work may be used under the terms of the Creative Commons Attribution 4.0 licence. Any further distribution of this work must maintain attribution to the author(s) and the title of the work, journal citation and DOI.
1. Introduction
The newly reported MnBi2Te4 has attracted wide-ranging research interest for its special properties of intrinsic magnetic topological [1–4]. Moreover, it has a long-term explored the axion insulator phase, which is confirmed by experiments [5]. Ordinary topological insulator [6, 7] doped magnetism is widely used owing to its novel physical properties, such as quantized anomalous Hall effect (QAHE) [8–10], topological magnetoelectric effect [11], and Majorana fermions [12] with topological superconductivity. The layered van der Waals antiferromagnetic topological insulator MnBi2Te4 was discovered by a combination of authoritative theoretical calculations and significant experimental results and was further confirmed to have a non-trivial topological property [13]. A-type antiferromagnetism is the magnetic ground state of MnBi2Te4, with a topological non-trivial adjustable band gap of approximately 0.2 eV [1]. Therefore, the band gap of the MnBi2Te4 antiferromagnetic bulk structure can be controlled by applying strain. Experimenters have continuously synthesized single-crystal MnBi2Te4 through different methods [14–21]. Inevitably, various physical properties of MnBi2Te4 have been fully studied in the field of condensed matter physics (Dirac cone, Chen number, phase transition and so on) [14, 22–39].
Most interestingly, both theoretical and experimental studies have shown that MnBi2Te4 is very sensitive to the changes of the lattice constant [1], and it is reasonable and feasible to carry out strain studies on the material. Strain control is a very common method for exploring the properties of material, and it has been widely used in various types of research [40–51]. Typically, methods of strain control are relatively widely used in the topological insulator Bi–Te(Se) family [40, 52–54]. According to the latest research, MnBi2Te4 undergoes a phase transition between semiconductor and metal under the influence of external pressure, and the presence of a pressure-induced amorphous state is observed [55]. Chen et al also found that through the application of stress (hydrostatic pressure), the lattice constant, resistivity, and carrier concentration of MnBi2Te4 will change with the different amounts of strains. At the same time, that study also described the evolution curve of volume with pressure by the Birch–Murnaghan equation and conducted magnetic phase transition studies [47]. Unlike ordinary topological insulators protected by time-reversal symmetry, MnBi2Te4 undergoes time-reversal breaking due to the introduction of magnetism, but it also has topologically non-trivial surface states at specific surfaces such as the (110) terminal [1, 3]. The (110) terminal is the surface that possesses the symmetry represented by the τ1/2 half translation operator along the crystal axis connected by two counter-spin Mn atomic layers in figure 1(a), and forms a combined symmetry with the broken time-reversal symmetry operator Θ, expressed as S = Θτ1/2 [1, 23]. The surface states of MnBi2Te4 with different magnetic configurations are investigated in a combination of theory and experiment, and it is found that the surface Dirac cone appears to be insensitive to antiferromagnetic ordering due to weak magnetic coupling [23]. The recent study on surface state change under different special strain modulation for MnBi2Te4-derived materials MnSb2Te4, MnBi2Se4, and MnSb2Se4 have been also reported [56].
Figure 1. (a) MnBi2Te4 structure and its (b) top view. (c) Brillouin zones of bulk MnBi2Te4.
Download figure:
Standard image High-resolution imageHowever, the current pressure-strain research on MnBi2Te4 is not systematic, especially in terms of the strain regulation of its electronic structure. The influence of strain on the electronic structure of MnBi2Te4 remains an important topic of investigation. Therefore, it is urgent to further explore the inherent special properties of MnBi2Te4 via its electronic structure characteristics. In addition, although surface state studies of MnBi2Te4, including the series of materials from which it is derived as a parent, have been relatively prevalent in recent years, studies of the specific effects of strain on the topological properties of MnBi2Te4 have always been so incomplete. Thus, in this paper, the first-principles calculations of the hydrostatic strain applied to the anti-ferromagnetic structure of MnBi2Te4 are carried out, focusing on changes in the band structure and charge density. One of the most meaningful results obtained is the determination of the strain value at which the conduction and valence bands just cross at the Γ point. Base on the calculation of the surface states, it is interesting that the system under the action of 2.12% strain shows a topological quantum phase transition. Our results both provide a meaningful guideline to the strain modulation of the electronic structure of MnBi2Te4, which can be instructive for experimental preparation and potential applications.
2. Methods
First-principles calculations based on density functional theory (DFT) using pseudopotential plane-wave method with projected augmented wave [57] were implemented by Vienna ab initio simulation package [58, 59]. The energy cutoff of the plane wave is set to 350 eV. Perdew–Burke–Ernzerhof type generalized gradient approximation exchange-correlation function [60] and spin–orbit coupling are used. Due to the strong correlation between the 3d orbit of Mn atoms, the U value of 4 eV was considered [3]. Figure 1(a) shows the unit cell structure of MnBi2Te4, whose traditional unit cell belongs to the R
m space group (No. 166). The lattice constant is a = b = 4.36 Å, c = 40.6 Å [3]. The anti-ferromagnetic unit cell we calculated is marked by a black dotted pink filled box, and the upper and lower spins are indicated by arrows. The first Brillouin zone containing all high symmetry points is given by figure 1(c). The 5 μB initial magnetic moments per manganese atom is assigned to the MnBi2Te4 system according to the A-type antiferromagnetic configuration [3]. The anti-ferromagnetic configuration is composed of two ferromagnetic sevenfold layers (Mn–Te–Bi–Te–Te–Bi–Te), as shown in figure 1(a). A force criterion of 0.02 eV Å−1 was applied during structure optimizations. A Monkhorst–Pack k-point mesh is used for sampling the Brillouin zone for the relaxation and self-consistency of calculations [3], while 15 × 15 × 5 for surface states and Wannier charge center (WCC) calculations via using WannierTools packages [61]. The high-symmetry points of the inverted lattice space taken by the band calculation are Γ(0, 0, 0), Z(π, π, π), F(π, π, 0), L(π, 0, 0).
Hydrostatic strain is achieved by changing the lattice constant of the initial traditional unit cell. The specific expression is as follows:
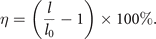
η represents the applied strain value while l and l0 represent the directions of the three lattice constants of the strained and initial structures, respectively.
The specific values of the lattice constants after applying hydrostatic strain to the system are given in table 1.
Table 1. Variation of the lattice constant of the system under hydrostatic strain.
η (%) | −5 | −4 | −3 | −2 | −1 | 0 | 1 | 2 | 3 | 4 | 5 |
---|---|---|---|---|---|---|---|---|---|---|---|
a = b (Å) | 4.142 | 4.1856 | 4.2292 | 4.2728 | 4.3164 | 4.3600 | 4.4036 | 4.4472 | 4.4908 | 4.5344 | 4.578 |
c (Å) | 38.57 | 38.976 | 39.382 | 39.788 | 40.194 | 40.600 | 41.006 | 41.412 | 41.818 | 42.224 | 42.63 |
3. Results and discussion
Figure 2 shows the evolution of the band structure for MnBi2Te4 with hydrostatic pressure strain. A direct band gap of approximately 0.2 eV appears at the Z high-symmetry point for the unstrained pristine system. Figures 2(a)–(f) represent the applied compressive strains, while figures 2(h)–(l) display the effect of tensile strains. It is obvious from both figures, that the application of strain has a significant influence on the band structure near the Fermi level. It is also clearly visible that in the strain range with an absolute value of 5%, the band gap changes from direct to indirect, regardless of whether the compressive or tensile strain is applied. However, it is easier under compressive strain to present an indirect band gap and the valence-band maximum (VBM) is kept at the Z high-symmetry point. For tensile strain, the indirect band gap only appears when the strain is greater than 4%. In particular, after the compressive strain exceeds 7%, it naturally appears as a metallic state with the conduction and valence bands very close to each other. In addition, a direct band gap is presented at Γ, which is the result of excessive compression. What is interesting is that, except for the direct band gap strain results at Z, the conduction-band minimum (CBM) of the remaining energy bands are all at the Γ high-symmetry point. The most notable phenomenon is that the band gap is very small when 2% hydrostatic pressure tensile strain is applied, which is shown in the direct band gap at Γ.
Figure 2. The band structures of MnBi2Te4 under (a)–(f) hydrostatic compression, (g) no strain, and (h)–(l) hydrostatic tension. The specific strain values are correspondingly marked in the figure, the red and blue balls represent CBM and VBM, respectively. The light blue dash-dot represents the Fermi level.
Download figure:
Standard image High-resolution imageTo further explore the distribution of the electron cloud at the CBM and VBM of the band structure, the local charge densities at the CBM and VBM of the band structure were calculated under different hydrostatic pressure strains, which is shown in the upper and lower half-panels of figure 3, respectively. The high-symmetry points at the CBM or VBM of the electronic structure after each pressure strain are marked under the local charge-density diagram (Z+ indicates the place near the Z high-symmetry point in the band structure). It is easy to find that most of the electrons in the CBM and VBM are contributed by Te and Bi atoms. It can also be seen that strain is an important factor leading to changes in the CBM and VBM. After the strain is applied, the CBM tends to shift to Γ, while the VBM does not change under the action of compressive strain. Most obviously, under the premise of controlling the isosurface value to be consistent, the charge densities of the CBM and VBM of the 3% strain system are almost 0, indicating that the interaction between the atoms at the CBM and VBM of the structure under this strain is weak. This may be the reason that the band gap reopened. When the VBM is at Z or Z+, most of the electrons are contributed by Te atoms, while at the Γ high-symmetry point, the contribution is more from Bi atoms. Most of the CBM of the band structure is at the Γ high-symmetry point, and the electron-cloud distribution of the corresponding atoms between each sevenfold layer of the other system, except 4% or 5%, is consistent (the sevenfold layer is Te–Bi–Te–Mn–Te–Bi–Te). At the CBM, the Te atom in the inner layer of the sevenfold layer (Te4 in figure 4) and the Bi atom near it accumulate more electrons as the degree of compression increases, and the overlap of the charge density gradually increases, indicating the interaction enhanced between nearby Te and Bi atoms. Therefore, the hydrostatic strain affects the charge-density distribution at the CBM and VBM, which leads to changes in the electronic structure band gap and other characteristics.
The Bader-charge difference in figure 4, which refers to the difference between the Bader charge of each atom and the initial charge, allows the gains and losses of each atom to be observed more accurately. The initial charge is Mn:7 |e|, Bi:5 |e|, Te:6 |e|, there are a total of 82 charges. Figure 4(e) shows the anti-ferromagnetic structure of MnBi2Te4 composed of two ferromagnetic sevenfold layers, and the corresponding positions of 14 atoms are marked by numbers. It can be seen that, except for the Te atoms (i.e. Te2, Te7, Te3, and Te6) at the boundary between the sevenfold layers, the Bader charges of the other atoms of the same species have a highly consistent trend with strain. It is conceivable that strain mainly affects the Bader charge of Te atoms at the junction of the sevenfold layer, which makes the charge distribution between Te atoms at the boundary of different sevenfold layers different, in particular, the compressive-strain effect is more obvious. In addition, the numerical value shows that Te atoms gain electrons while Bi atoms and Mn atoms lose electrons. Under the action of hydrostatic pressure strain, the deviation trend in each atom's Bader charge from its initial charge roughly increases with the increasing lattice constant. What is more noteworthy is that when the 1% tensile strain is applied, the electron charges of the Mn atomic layer and nearby Te atomic layer are the most obvious, which is the inherent reason the direct band gap changes from the Z high-symmetry point to the Γ high-symmetry point. From the current perspective, the hydrostatic strain can affect the band structure, charge distribution, and atomic distance of materials.
Figure 3. Local charges of intrinsic magnetic anti-ferromagnetic MnBi2Te4 at CBM (top panel) and VBM (bottom panel) under different strains. (The isosurface values are all 0.0007 eV/bohr3, yellow represents charge accumulation, and blue represents charge reduction.)
Download figure:
Standard image High-resolution imageFigure 4. (a)–(d) The Δ charge of each atom of MnBi2Te4 under different strains, which represent the Bader charge subtract the initial valence charge. (e) The position of each atom, including 4 atomic intervals. (The initial valence charge is Mn:7 |e|, Bi:5 |e|, Te:6 |e|.)
Download figure:
Standard image High-resolution imageTherefore, the various effects of strain on the system are summarized, and the results are shown in figure 5. As shown in figures 5(a) and (b), the variation of the CBM, VBM, and band gap values with strain can be observed. The most noteworthy is that, after gradually refining the calculation, the strain value at which the band gap is exactly 0, is finally determined, namely 2.12%. Figure 5(c) shows evolution curves of energy with volume and dependence of lattice constants a and c under hydrostatic pressure (where V0 is the volume of the initial structure). The value range of the volume variable is −7% to 5%, and it is found that the energy change after tensile strain is stable and generally lower than that of compressive strain. In particular, the structural energy near 1% is the lowest, which means that the system is the most stable. This result is also consistent with the strongest interaction between the Mn atomic layer and nearby Te atomic layer under the action of 1% strain.
Figure 5. (a) The CBM, VBM, and band gap evolution curves of MnBi2Te4 with different strains and (b) locally enlarged view of the shaded box. (c) Evolution curves of energy with volume and dependence of lattice constants a and c under hydrostatic pressure. (d) Variation trend of atomic distance with strains. Different interatomic distances di (i = 1–4) are given in figure 4(e).
Download figure:
Standard image High-resolution imageCombined with figure 5(d), it is easy to see the relationship between inter-atomic distance and strain. Except for the slope of the d4 curve at tensile strain is greater than that at compressive strain, the strain curves of the other di (i = 1–3) values are almost linear, and the slopes increase sequentially (the details of the interlayer spacing di (i = 1–4) are marked in figure 4(e)). It is reasonable to draw a conclusion that the closer the layer spacing of the middle Mn layer is, the less likely it is to be affected by external strain, which is the difference between the strengths of the ionic bonds. The large change in d4 is because the Te atomic layer between the sevenfold layer is affected by the van der Waals force and is very susceptible to strain, especially tensile strain. Therefore, it can be said that MnBi2Te4 is very sensitive to the lattice constant because it has a layered structure that is easy to change. Notably, reducing the interlayer coupling effect of anti-ferromagnetism so that a stable ferromagnetic state can be generated under a low external magnetic field is undoubtedly a key goal of the experimenters in exploring the QAHE. Our results are an important reference for the study of QAHE on experiments.
Given that the conduction and valence bands are crossed at the Γ high-symmetry point under the condition of 2.12% strain, its band structure, density of states, and charge density are compared with those of the unstrained system. Figures 6 and 7 are the band structure and density of states combination diagrams of the unstrained system and the 2.12%-tensile-strain system, respectively. It can be seen from figures 6(b) and 7(b) that the band structure near the Fermi level is mainly composed of Bi and Te atoms. In addition, the two kinds of atoms show a tendency to balance each other. Furthermore, in their projected band structures, as shown in figures 6(c) and 7(c), and the corresponding partial state density (figures 6(d) and 7(d)), it is obvious that there is a band inversion in the p orbitals of Bi and Te atoms. However, the phenomenon of band inversion after 2.12% strain is weakened. The reason for this result is that there are fewer orbital-occupied states at the intersection of band structures, so the inversion phenomenon shown in the thumbnail in figure 7(c) is not obvious. In fact, the inversion phenomenon of band structure still exists from the density of states, as shown in figure 7(d).
Figure 6. Strain-free system of MnBi2Te4: (a) band structure of the strain-free system. (b) The total density of states (red dot) and partial density of states (PDOS). (c) Band structure projection of the p orbitals of the Bi and Te atoms (partially enlarged view of the embedded dotted frame). (d) The PDOS of p orbitals of Bi and Te atoms. The gray dotted line indicates Fermi level.
Download figure:
Standard image High-resolution imageFigure 7. The system of MnBi2Te4 under 2.12% hydrostatic strain: (a) band structure. (b)The total density of states (red dot) and PDOS. (c) Band structure projection of the p orbitals of the Bi and Te atoms (partially enlarged view of the embedded dotted frame). (d) The PDOS of p orbitals of Bi and Te atoms. The gray dotted line indicates Fermi level.
Download figure:
Standard image High-resolution imageTo essentially compare the potential topological properties of the unstrained and 2.12% strained systems, we calculated their surface state and WCC through the maximally-localized Wannier functions, as shown in figure 8. Figures 8(a) and (c) show a comparison between the band structure of MnBi2Te4 obtained from first-principles and the WannierTools calculation, both of the Wannier functions interpolation is indeed in excellent agreement with the first-principles results. Comparing their surface states of the (110) termination in figures 8(e) and (g), it is found that the surface states of the unstrained system are closed and exhibit strong topological properties, and conversely, the system under 2.12% strain exhibits trivial topological properties with open surface states. At the (110) surface protected by S-symmetry, the closing surface helical state emergy at the strain absence, but a surface band gap of 0.01 eV opens along with the appearance of the gapless bulk state because of the introduction of 2.12% hydrostatic strain, however, the double degeneracy is still maintained in the bulk band structure. The appearance of a gapped surface state transforms the system from a topological insulator to an ordinary insulator. Our surface state result of the unstrained system is in full consistent with the recently reported surface state topological properties of the MnBi2Te4 family of materials [1, 3, 23, 62, 63]. Correspondingly, the WCC calculations in figures 8(b) and (d) also show that the 2.12% strain changes the topological invariance Z2 of the system from 1 to 0, corresponding to the topological property change from non-trivial to trivial. To confirm that a topological quantum phase transition occurs in the system under 2.12% strain, we have also calculated the surface states at 1% and 3%, as shown in figures 8(f) and (h). From figures 8(e)–(h), we can see that at 1% the system remains a closed surface state, while the surface state opens up when the strain is higher than 2.12% and the surface state opens up a larger band gap at 3%, indicating that the system does undergo a topological phase transition.
Figure 8. The comparison of the band structures of (a) the no-strain system and (c) the 2.12% hydrostatic strain system. The results of the WCC calculations in the kz = 0 plane of (b) the no-strain system and (d) the 2.12% hydrostatic strain system. (e)–(h) are the surface states in the (110) plane of the no-strain, the +1% strain, the +2.12% strain, and the +3% strain systems, respectively. A local enlargement of the surface state near the Fermi surface is included in (e)–(h).
Download figure:
Standard image High-resolution imageTo compare the difference in charge distribution between the zero-bulk-band-gap system and the initial system, their charge densities were calculated separately and a charge density difference diagram was made. As is well know, charge density difference helps one visually see the general direction of charge transfer. Figure 9 shows the charge-density diagrams of the two systems of strain-free and 2.12% strain, where figures 9(a) and (b) are the three-dimensional (3D) and two-dimensional (2D) diagrams, respectively, of the charge density difference of their 2 × 2 × 2 supercells. Figures 9(c) and (d) are the charge-density maps of the unstrained and 2.12% strain systems of the unit cell [(001) planes]. Their charge density differences are shown in figure 9(e). The charge density difference is obtained by subtracting the charge density of the two systems, as in the expression Δρ = ρperfect − ρstrain. Based on figures 9(a) and (b), it can be seen that, compared with the 2.12%-strained system, the unstrained system accumulates charges near Mn and Te atoms but loses electrons near Bi. This shows that the hydrostatic strain of 2.12% for strengthening the bonding between the Te atomic layer of the system and the Bi atomic layer in the sevenfold layer. It can be seen more intuitively from figure 9(e) that the system without strain has less electronic localization near the Bi atom than the 2.12%-strain system. This means that the 2.12% strain is beneficial to attracting more electrons near the Bi atom, which verifies the fact of the enhanced interaction between Bi and Te atoms. In contrast, it weakens the coupling between the sevenfold layer, which makes the structure easier to peel off, decreases the effect of anti-ferromagnetism, and reduces the degree of band inversion at the same time. In fact, this is the essential reason why MnBi2Te4 can undergo topological phase transition after 2.12% strain action.
Figure 9. (a) Three-dimensional and (b) two-dimensional of the charge density difference of MnBi2Te4 between the unstrained system and 2.12% hydrostatic strain system in the (100) plane. The two-dimensional charge density of (c) unstrained and (d) 2.12% hydrostatic strain systems(e) and their charge density difference in the (001) plane. (The isosurface value of the three-dimensional graph is 0.008 eV/bohr3 and the saturation values of the two-dimensional graphs are marked with scales in the corresponding graphs.)
Download figure:
Standard image High-resolution image4. Conclusions
Hydrostatic strain control of the intrinsic anti-ferromagnetic topological insulator MnBi2Te4 has been investigated using first-principles calculations based on DFT. Through the modulation of band structure under pressure strain, the insulator-metal phase transition and topological quantum phase transition were found. In addition, pressure strain has an influence on the positions of the CBM and VBM and also the charge-density distribution, from which the mutual transformation between the direct and indirect band gaps can be achieved. In particular, the conduction and valence bands cross at Γ point when the strain is 2.12%. The compressive strain will cause a difference in the charge distribution of the originally equivalent sevenfold boundary Te atoms. The atomic distance shows that the distance between sevenfold layers is most affected. Compressive strain can enhance the bonding between the Te atomic layers at the sevenfold layer boundary. On the contrary, tensile strain increases the sevenfold layer spacing, which means the anti-ferromagnetic coupling strength decreases accordingly. Finally, through the calculation of topological characteristics and surface state, we find that MnBi2Te4 undergoes a topological quantum phase transition with the occurrence of pressure strain. Our result provides theoretical guidance for both experimental research and the potential application of the intrinsic magnetic insulator.
Conflict of interest
The authors declare no conflict of interest.
Author contributions
J-M Zhang conceived and supervised this work. W-T Guo and L Huang performed the calculations. W-T Guo and J-M Zhang wrote the manuscript. Y Yang and Z Huang analyzed the datas and provided valuable and constructive suggestions.
Acknowledgments
We acknowledge the financial support by the National Natural Science Foundation of China (Nos. 11874113 and 61804030) and the Natural Science Foundation of Fujian Province of China (No. 2020J02018). The work was carried out at National Supercomputer Center in Tianjin, and the calculations were performed on TianHe-1(A).
Data availability statement
The data that support the findings of this study are available upon reasonable request from the authors