Abstract
In this paper, the theory of collisional and turbulent transport of impurities in tokamak plasmas is reviewed. The results are presented with the aim of providing at the same time a historical reconstruction of the scientific progress and a complete description of the present theoretical knowledge, with a hopefully sufficiently complete reference to the works which have been published in the field in the last decades. After a general introduction on the physics challenges offered by the problem of impurity transport and their relevance for practical nuclear fusion energy, the theory of collisional transport is presented. Here a specific section is also dedicated to the transport parallel to the magnetic field lines. A complete review of the transport mechanisms produced by turbulence follows. The corresponding comparisons between theoretical predictions and experimental observations are also presented, highlighting the influence that the validation activities had in motivating further theoretical investigations. The paper is completed by a section on the direct interactions between collisional and turbulent transport and by a final specific review dedicated to the progress in the theory–based modelling activities. In the writing of this review paper, the main goal has been to combine readability with completeness and scientific rigour, providing a comprehensive list of references for deeper documentation on specific aspects.
Export citation and abstract BibTeX RIS

Original content from this work may be used under the terms of the Creative Commons Attribution 4.0 license. Any further distribution of this work must maintain attribution to the author(s) and the title of the work, journal citation and DOI.
1. Introduction
Impurities are an unavoidable and likely necessary component of a fusion reactor plasma, with strong impact on both performance and operational possibilities. The capability of understanding and predicting their behavior in tokamaks is therefore of paramount importance for the achievement of practical fusion energy. At the same time the study of the transport of impurities is a research area of particular interest from the physics standpoint. A too high concentration of impurities in the plasma can have very negative effects, even leading to plasma disruptions (e.g. [1]). At the same time, if their presence is kept under control, they can have positive effects, as the seeding of some impurities is also observed to improve the confinement [2–5], and will certainly be required in a reactor to increase peripheral radiation and reduce the heat loads which reach the divertor plates [6–10].
The amount of impurities present in the different regions of the plasma, from the core to the edge, is the result of a combination of effects connected with the transport and the strength of their sources at the periphery, as well as at the centre for He ash in a burning plasma. With respect to the main plasma components, which are usually dominated by turbulent transport with limited role of the collisional transport, impurities have the additional physically interesting but more complex property that their transport is concomitantly governed by both collisional and turbulent mechanisms, whose relative role depends not only on the plasma conditions, but also on the impurity charge and mass. The large variations in mass and charge that impurities can have also provide additional dimensions in the investigation of the transport properties with respect to the main plasma species. Not only can impurities have dominant components of neoclassical transport in addition to the turbulent transport, but also high mass and charge open the possibility of impurity density distributions which are not poloidally homogeneous on the magnetic flux surfaces, providing additional complexity to the problem. These aspects increase the number of physical parameters whose impact has to be theoretically understood but also offer additional ways which can be experimentally applied to modify their radial profiles.
The fact that collisional transport can produce density profiles of highly charged impurities with an extreme central peaking was realized since the early times of the research on magnetized plasmas, for both classical [11] and neoclassical [12, 13] transport. Since those times, these first results have been source of motivation for experimental and theoretical research on impurity transport.
Finally, differently from heat transport [14, 15], but in close analogy to particle transport of the main plasma species [16] and to some of the properties of momentum transport [17, 18], the transport of impurities requires the investigation of both diagonal and off-diagonal components [19], that is, the investigation of the properties of both diffusion and convection, where, in particular for highly charged impurities, the role of neoclassical convection can become dominant.
The need of metallic walls for fusion reactors (e.g. [20–24]) and in particular the use of highly charged elements like molybdenum and tungsten in present day devices [25–27] has renewed the interest on impurity transport studies in recent years, leading to important progress in the theoretical, the modelling and the experimental research. This paper aims at providing a review of this progress, particularly from the theoretical and modelling standpoints, but also including the results obtained in the comparison with the experiments, the physical understanding of the observations and the validation of the theoretical predictions. This paper is dedicated to thermal particles only. Both the neoclassical and the turbulent transport are reviewed, as well as very recent investigations dedicated to the direct interaction between the two transport processes. Section 2 is dedicated to the collisional neoclassical transport, where more emphasis is given to recent developments, particularly in connection with the impact of poloidal inhomogeneities of the impurity density distribution and their related experimental relevance. For conventional neoclassical transport theory, the well–known review paper of Hirshman and Sigmar [28] will be considered as general reference. Consequently, section 2.1 is specifically dedicated to the parallel transport, which allows the calculation of the distribution of impurities on the magnetic flux surfaces and thereby their poloidal asymmetries, while section 2.2 reviews the radial collisional transport, which can be strongly modified in case of inhomogeneous poloidal distributions of the impurity density. Section 3 is dedicated to the turbulent transport. Here a complete description of all of the mechanisms producing impurity transport will be presented, since, on this side, it can be considered that a comprehensive review is still missing. A complete identification of turbulent diffusion and turbulent convection processes within a local and δf description is presented, with specific subsections which allow the dependencies on density, temperature and rotation gradients to be identified, and dependencies on impurity charge and mass to be compared to those of neoclassical transport. Corresponding comparisons with experimental observations are also described, as these have often motivated the theoretical progress. Section 4 describes the present knowledge on the effects which arise from the direct interaction between neoclassical and turbulent transport, by which the total transport cannot be simply considered to be provided by the sum of the two transport components, as separately computed. This largely is a new field of investigation. Finally, section 5 is dedicated to the modelling of impurity transport and impurity density profiles. The emphasis is given to studies which have been performed more recently, when more comprehensive theoretical models became available and have been applied. Although some of the results presented in the previous sections are unavoidably repeated in section 5, a somewhat self–contained review of the modelling activities is considered to be an important and useful element of this paper. This section also includes a brief description of additional effects, particularly connected with the presence of magneto-hydrodynamic (MHD) modes, which have a clear impact on the impurity density profiles. Although this review is mainly dedicated to actual transport processes in the physical microscopic sense, like turbulence and collisions, the impact of MHD modes is important and deserves to be briefly mentioned. In section 6 conclusions are briefly drawn, recalling the main current open issues towards a complete predictive capability for future devices.
2. Collisional transport of impurities
Since the frequency of Coulomb collisions is proportional to the square of the charge of each colliding particle, collisional transport of impurities can be more regularly important as compared to the collisional particle and heat transport of the main ion species, and of course of the electrons. In particular, for highly charged impurities, the neoclassical transport is hardly negligible, and can be dominant in the central part of the plasma over the turbulent component. Conventional ordering of neoclassical transport is organized through the assumption that at the lowest order in an expansion in the normalized poloidal Larmor radius, the electrostatic potential, the densities and the temperatures of the plasma components are homogeneous over the magnetic flux surfaces [28–30]. This ordering, based on the parameter , leads to the conventional local treatment of neoclassical transport theory (here
is the main ion poloidal Larmor radius and
is a characteristic length of variation of the temperature and density radial profiles, with
the main ion thermal velocity and
the cyclotron frequency with the poloidal magnetic field). It is well known that this ordering can be inappropriate for the description of plasma regions with large poloidal orbits, in particular close to the magnetic axis, and where the gradients of the kinetic profiles become large, like in the edge pedestal region. Moreover, this ordering can also be not satisfied by highly charged impurities, which, due to the high collisional frequency, can produce more significant friction in the parallel direction preventing the density to be constant over the magnetic flux surfaces [31], as regular ordering would in constrast expect. The additional parameter
can become of order unity if Z is sufficiently large (here νii
is the ion–ion collision frequency, q and R the safety factor and major radius, and Z is the impurity charge). With Δ≈ 1, even though
, parallel friction can be large enough to lead to the development of poloidal asymmetries of the impurity density which can strongly affect the radial impurity neoclassical transport [31–36]. Additional effects can strongly modify the poloidal distribution of the impurity density, in particular the plasma rotation [32, 36–41] as well as the presence of particle species featuring significant temperature anisotropies, like resonant particle species in the presence of ion cyclotron resonance heating (ICRH), which also develop non homogeneous poloidal distributions [42–47]. Even the significantly more limited temperature anisotropies developed by fast ions produced by neutral beam injection (NBI) can have a non–negligible impact on the poloidal distribution of heavy impurities [45, 48]. In general, any small poloidal asymmetry of the electrostatic potential has a very strong effect on the distribution of the high-Z impurities as a consequence of the strong parallel electric force. Poloidal asymmetries can increase or reduce the neoclassical impurity transport, particularly for heavy and highly charged impurities, up to levels which can largely exceed the turbulent transport, making neoclassical impurity convection the dominant term, or down to levels which can be even lower than the corresponding collisional classical transport [31, 36]. Therefore, an accurate calculation of the radial collisional transport also requires the consistent calculation of the poloidal asymmetry of the impurity density, particularly for heavy and highly charged impurities, where the large mass makes that impurities are affected by centrifugal forces and the high charge by the electric field and by the friction with the main ions. Within a conventional neoclassical ordering, where both
and
[29, 37], sources of poloidal asymmetry only come from external effects like rotation or additional particle species with poloidal asymmetries affecting the electrostatic potential. The outboard localization of heavy impurities produced by the toroidal centrifugal force strongly increases the neoclassical transport [32, 38, 39]. Several recent works dedicated to the modelling of tungsten (W) impurity transport, have included this effect leading to a clear experimental realization of their essential importance in the modelling [49–52] and in the understanding of the experimental observations and the causes of the observed W accumulation, particularly in conditions of NBI only heating. This has been possible also thanks to the application of the drift-kinetic solver NEO [53–55], which is built on the conventional ordering
and
[29, 37], but which also includes the impact of toroidal rotation effects [54] as well as the effects due to the presence of an ICRH minority with much larger perpendicular than parallel temperatures. This latter feature was applied in [50] for the first time in the modelling of the radial transport of tungsten. Of course, in the presence of these particle species with temperature anisotropies, a realistic model has to be applied for the calculation of the density distribution of this particle species [42–46]. These poloidal density distributions have to be considered in the calculation of the poloidal asymmetry of the electrostatic potential which can be obtained by imposing quasi-neutrality and which as such also affects the distribution of the impurity density. All of these elements can be consistently included by a complete treatment of the parallel impurity transport, which thereby becomes an essential first element to be considered before the problem of the perpendicular (radial) transport is tackled.
2.1. Parallel impurity transport
The description of parallel impurity transport can be developed starting from the zero and first order moments of the drift-kinetic equation, that is, the continuity and momentum balance equations [28, 29],


Here, nZ
and are the impurity density and pressure, with
the ion temperature,
the fluid velocity, πZ
the viscous tensor, and φ the electrostatic potential. The friction force
includes the friction of the impurity with all the other species. The magnetic field is given by the usual general expression
, where ψ is the poloidal flux divided by 2π, ϕ is the toroidal angle, and the function I(ψ) is related to the poloidal current inside a magnetic surface. The perpendicular projection of the momentum balance, keeping only the dominant terms provided by pressure gradient and electric force, in combination with the divergence free condition, provides the usual expression of the first order velocity, which relies upon the assumption that the flow velocity is mostly within the flux surface (or, more precisely, that the divergence of the radial flux is much smaller than that of the flux on the magnetic surface)

where

More generally, ωZ can also include the contributions from the inertial terms from equation (2).
The parallel projection of the momentum balance gives
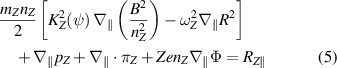
where from left to right we identify the inertia terms connected with poloidal and toroidal centrifugal forces, the pressure gradient term, where the temperature can be considered homogeneous on magnetic surfaces, the viscous term and finally the electric and the friction parallel forces. This is a complete expression, which describes all the collisionality regimes and includes both poloidal and toroidal inertial (centrifugal) terms as well as friction. Starting from this expression, depending on the collisional regime, the adopted ordering and the physical approach, different terms have been included or neglected in various models. In particular, theoretical approaches have been developed with different assumptions on the collisionality regime of the main ions (banana, banana–plateau or Pfirsch–Schlüter), on the collisionality regime and the charge of the impurities (thereby neglecting the viscosity tensor term for impurities in the high collisionality limit), considering impurities only in the trace limit (), neglecting inertial terms and thereby centrifugal forces or neglecting the friction force at the lowest order. A comprehensive and more detailed recent review of the different theoretical approaches to parallel impurity transport can be found in [56], section 2.2 and, correspondingly, comparisons of the theoretical predictions of the poloidal variation of a high-Z impurity density have been recently performed on molybdenum measurements in C-Mod [57]. More recent works which are not included in that review are the following [35, 36, 58]. In [58] the role of the Coriolis force is also considered, and a model is developed in which the combined effects of the centrifugal force, the Coriolis force, the poloidal electric field and the ion-impurity friction force on the in–out impurity density asymmetry are investigated. Maget et al [35, 36] extend the work of [32] with the inclusion of the diamagnetic velocity of the impurities in the calculation of the impurity–main ions parallel friction, removing the assumption
and also allowing for the possibility of poloidal inhomogeneities of the main ion density.
The calculation of the friction force plays a critical role, since, as it will be also presented in the next subsection, it is directly connected with both the classical and neoclassical components of the collisional radial flux. In fact, from the momentum balance equation, equation (2), one can see that the perpendicular (radial) particle flux is produced by both perpendicular and parallel friction [29], where the perpendicular friction causes classical collisional transport and parallel friction the neoclassical collisional transport. One of the first examples of the realization of its importance and its direct calculation in the Pfirsch–Schlüter regime can be found in [59]. The friction force between the impurity with charge Z and the (main) ion species 'i' is defined as the first order velocity moment of the collision operator,

where the second equality expresses the property of momentum conservation of the Coulomb collisions. Of course, knowledge of the main ion distribution function, for instance by means of a numerical solver, allows the calculation of the friction force by integration in the velocity space. A very common approach considers an expansion of the perturbed distribution function in generalized Laguerre polynomials [28], where the coefficients of the polynomials are directly connected to macroscopic moments of the distribution function, with the first two providing the parallel particle and heat flows. The corresponding expansion of the friction force allows one to express this force as a linear combination of these moments of the distribution function, where the coefficients are the friction coefficients [28]. Analytical expressions for the parallel friction have been derived in various limits, mainly connected with different collisionality regimes of the main ions, in the banana regime [31, 32], in the Pfirsch–Schlüter regime [33] and more recently the plateau regime [34]. The parallel particle and heat flows can be expressed as linear combinations of radial density and temperature gradients as well as the corresponding poloidal flow functions KZ (ψ). Assuming isothermal flux surfaces, the parallel momentum balance equation becomes an equation for the poloidal distribution of the impurity density,
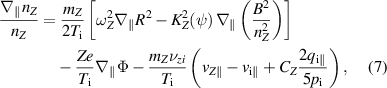
where on the right hand side we can again identify the toroidal and poloidal centrifugal terms, the parallel electric force, and the parallel friction term. In equation (7) we have neglected the viscous tensor term, which becomes small for highly charged impurities, typically in a high collisionality regime [31]. According to the general approach that we have just described, the parallel friction has been expressed as

with CZ
an appropriate coefficient and the parallel ion heat flow, which can be expressed as proportional to the ion temperature radial gradient. In general, in the limit of
, the diamagnetic term can be neglected in the expression of the parallel impurity flow, and the expression for the parallel friction becomes independent of the radial gradients of the impurity. We notice that this does not rigorously correspond to a trace impurity limit
, but the trace limit still has the similarity of removing the dependence of the parallel friction on the impurity density. If in contrast the dependence of the parallel friction on the impurity density and on its radial gradient is kept through the inclusion of the diamagnetic velocity

then the equation for the parallel distribution of the impurity density also depends on the radial gradients of the impurity density profile through the friction term [35, 36]. In this more complete treatment the poloidal asymmetry of the impurity depends on the radial profile shape. Concomitantly, the radial transport depends on the poloidal asymmetry of the impurity, with the consequence that parallel and perpendicular dynamics are completely connected, and the solution leads to a 'natural' impurity poloidal asymmetry [35, 36] which is consistent with the radial profiles solution of the radial transport equation. In general, the parallel friction force can be expressed through a linear combination of radial gradients of the main ion density and ion temperature, through its dependence on the parallel particle and heat flows. The coefficients of this linear combination, including their poloidal dependence, vary with the collisionality regime. However, the impact of the friction term can be characterized by considering the following dimensionless parameter, first introduced in [31], which is largely equivalent, and more precisely quantifies, the impact of the ordering parameter Δ,

Here = r/R is the local inverse aspect ratio, and τiZ
is the ion–impurity collision time

while the corresponding ion–impurity collision frequency is given by . The characteristic perpendicular length
is provided by a linear combination of ion density and temperature logarithmic gradients (
and
with main ions in the banana [32] and the Pfirsch–Schlüter [33] regimes respectively). It is easy to observe that this parameter can be rewritten in the equivalent form

We also observe that friction becomes important when radial logarithmic gradients are large enough in combination with a sufficiently high ion collisionality, and in particular that it is proportional to the impurity charge squared. Therefore it is much stronger for highly charged impurities, whereas it is in general negligible for light impurities, even in the presence of large gradients in the pedestal region. It can also be expected that, at the low collisionalities of a reactor burning plasma, effects coming from friction are very small and only become important close to the separatrix in the peripheral part of the pedestal. In contrast, they can be significant over large portions of the minor radius in present devices for an impurity like W in high density L–mode plasmas, and certainly in the pedestal of present H–mode plasmas, even for light impurities.
The centrifugal term connected with the poloidal rotation is usually negligible and it is regularly neglected in almost the totality of the theoretical models. It has been recently considered in the analysis of ASDEX Upgrade light impurity (boron and nitrogen) density profiles which were concomitantly measured at the low and high field side [60], and has been found to become non–negligible very close to the separatrix, in the external region of the pedestal, while friction plays the dominant role over the entire pedestal.
The centrifugal term connected with the toroidal rotation is usually the dominant term for heavy impurities in the core plasma in the presence of toroidal rotation. It always has to be considered in consistent combination with the electrostatic potential term. The first complete treatment of this term computing the impact on the impurity density and its transport is in [38], starting from the rigorous approach developed in [37]. It has been followed by several other works [32, 36, 39–41]. The essential feature here is that while the centrifugal force pushes impurities on the outboard side, the electrostatic force connected with the different distribution of electrons and ions produced by the same rotation develops a parallel electric field which pushes the impurities on the high field side and slightly opposes the dominant effect of the centrifugal force. Other effects can contribute to the development of a parallel electric field. The most noticeable among these is connected with the presence of an ion species with a temperature anisotropy, as generated by ICRH [42–46] and also by NBI, although usually with weaker effects due to a lower level of anisotropy, but larger density of fast particles [45, 48].
The inhomogeneity of the poloidal distribution of the impurity density strongly affects the neoclassical transport. This can be easily understood, since the net radial flux averaged over the flux surface results from almost equal contributions of opposite sign from the outboard and the inboard sides. Thereby, any modification of the size of the inward or outward components, as produced by an increase of the particle density on the low or high field side, can directly lead to much larger radial fluxes [31–33, 35, 36, 38, 40, 41].
At present there are models and corresponding codes which allow these effects to be computed consistently, although with different levels of assumptions. No complete model is available which takes into account all of the possible sources of poloidal inhomogeneity and consistently computes the consequent radial transport while solving the drift-kinetic equation with a sufficiently complete expression of the collision operator. The drift–kinetic solver NEO [53–55] has been used in many recent applications for impurity transport calculation requiring the inclusion of centrifugal [49–52] and temperature anisotropy [50, 52] effects. This code is based on the Hinton ordering [37] and thereby includes centrifugal effects at the lowest order, but does not include friction effects at the lowest order, thereby it cannot reproduce effects which are instead included in the Helander approach [31], which allows friction effects to be considered at the lowest order. For the comparisons with experimental measurements, complete validation tests could be performed by simultaneous measurements of all of the components that concomitantly impact the parallel transport and determine the poloidal distribution of the impurities. Simultaneous measurements of the toroidal and poloidal flows and of the poloidal distribution of the impurities would provide a more stringent and complete test for the theoretical predictions [57].
2.2. Perpendicular collisional transport
As anticipated, and as well known [28, 29], classical and neoclassical perpendicular transport are connected with the perpendicular and the parallel components of the friction force respectively.
The usual approach is to consider the toroidal projection of the momentum balance equation, equation (2). It is of practical use to report here analytical expressions for the classical and neoclassical transport. From equation (2), and considering a rigid toroidal rotation only, with angular velocity Ω, we have

where we have also kept the contribution of the viscous tensor, responsible for the banana-plateau transport.
By taking the scalar product with R2∇ϕ, the toroidal component of this equation allows us to express the radial fluxes in the form

The second term, proportional to the perpendicular component of the friction, is the classical transport. The first term, proportional to the parallel component of the friction is the neoclassical transport. In the conventional situation of a poloidally homogeneous impurity density, if the viscous term is neglected, then, since , one has that
. In the case of poloidally inhomogeneous density, this solubility condition becomes
[31, 32], with the consequence that the neoclassical transport can be split into the two contributions
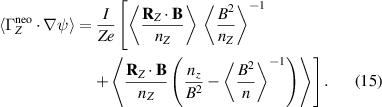
Here, the first term on the right hand side is the banana-plateau component, which is substantial only when the viscous term πZ
is important, while the second is the Pfirsch–Schlüter component, which is present independently of the strength of the viscous term. In the limit of highly charged impurities, , which thereby are in a high collisionality regime, the viscous term becomes small compared to the other terms [31], and the dominant contribution is provided by the Pfirsch–Schlüter component.
An analytical expression of the radial flux of Pfirsch–Schlüter impurity transport can then be obtained without making any assumption on the specific poloidal inhomogeneity of the impurity density distribution. Assuming main ions are in the banana limit and with a poloidally homogeneous main ion density, and neglecting the impurity diamagnetic velocity in the friction term, consistent with the limit , this reads [32, 40, 50, 51],

Here the numerical coefficient c0 is equal to 0.33 in the large aspect ratio limit with an ion–ion collision operator for the main ion distribution function given by pitch-angle scattering combined to a momentum restoring term [32]. The two geometrical coefficients PA and PB encapsulate the dependence on the poloidal distribution of the impurity density,
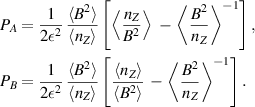
If the impurity density is poloidally homogeneous, in the large aspect ratio limit, these coefficients are PA
= 1 and PB
= 0. In present devices with large NBI heating PA
and PB
can be as large as 100 [61]. The result presented here closely follows the derivation in [32]. Analogous derivations, also in the limit , have been obtained with main ions in other collisionality regimes, in particular banana-plateau [34] and Pfirsch–Schlüter [33]. The treatment presented in [32] has been recently generalized to consistently include the diamagnetic velocity of the impurities in the friction term and the poloidal inhomogeneity of the main ions with sources of poloidal asymmetry caused by friction only [35], as well as produced by toroidal rotation or temperature anisotropies of ICRH resonant species [36]. A complete analytical treatment within the drift-kinetic approach valid for general geometry and arbitrary impurity charge has been also obtained in the limit in which both the main ions and the impurities are in the Pfirsch–Schlüter regime [41]. The combined impact of poloidal asymmetries and pressure anisotropies of the impurities on the neoclassical impurity transport has been recently presented in [62] with an analytical derivation, also in relation with the effects that can be produced by turbulence in directly affecting the collisional transport. The aspect of the direct interaction between turbulent and collisional transport will be reviewed in section 5. The analytical model presented in [40] and described by equation (16) has been compared with the predictions of the code NEO [53–55] in [63] for applications to JET plasmas. The NEO code contains a complete treatment of the linearized collision operator, valid in all collisionality regimes, but only assumes in input a poloidal inhomogeneity produced by the centrifugal force from toroidal rotation [38] or a specific form of temperature anisotropy of an ICRH resonant species, following [45], as applied e.g. in [50]. The analytical formula has been found to reproduce reasonably well the NEO numerical results, even when impurities move out of the Pfirsch–Schlüter limit, but requires ions in the banana limit. The strength of the temperature screening terms critically depends on the assumptions made for the calculation of the main ion parallel flow, which is based on an approximated collision operator and can become inappropriate in general geometry. The more general treatment presented in [35, 36], which directly includes friction in the self–consistent determination of the parallel distribution of the main ion and impurity densities, following [32] but without dropping the contribution from the impurity diamagnetic velocity in the friction force, requires main ions in the banana limit. It could be extended to other collisional regimes profiting of the results in [33, 34].
The possibility of developing a sufficiently accurate and fast model for routine integrated modelling applications should be considered a high priority in the direction of obtaining a complete pulse simulator which can be applied for the modelling of an entire plasma discharge with limited computational time. Fluid codes like NCLASS [64] and NEOART [65] based on the Hirshman–Sigmar approach [28] can be used for the calculation of the neoclassical transport of light impurities, but are limited to the most general assumptions of conventional neoclassical ordering and do not include any effect related to the poloidal asymmetry of heavy highly charged impurities. Therefore, in general these codes cannot be applied for realistic computations of the transport of heavy impurities in the presence of any poloidal asymmetry (while of course they are highly reliable for the calculation of heat conductivities of the main species and of the bootstrap current, as well as of the transport of impurities which do not feature any poloidal asymmetry). A set of practical analytical formulae, again in the limit of conventional neoclassical theory ordering, for both the Pfirsch–Schlüter and the banana-plateau contributions can be found here [66], where a useful summary (and the correction of some typos) of the formulae obtained in [28] is provided.
We recall that the entire treatment presented in this section always assumes that , that is, still follows the local neoclassical ordering. However, following [31] and related works [32–34] as well as more recently [35, 36], it can allow for a non-negligible friction force at the lowest order, that is
. The code NEO [53–55] follows the conventional neoclassical ordering which assumes both
and
, however, as already mentioned, it allows for poloidal inhomogeneities of the density distribution as produced by rotation [37, 38] and temperature anisotropies of ICRH resonant species. Considering that friction effects are usually small to negligible for high temperature (low collisionality) plasmas in high confinement regimes, the code NEO is a very practical and reliable tool for accurate applications dedicated to the calculation of the impact of toroidal rotation on heavy and highly charged impurities like W. It however requires considerable computational time for integrated modelling applications in a transport code.
Finally, as we already mentioned and it is well known, local neoclassical theory orders small the normalized poloidal Larmor radius δ. This ordering can become not appropriate to describe the plasma close to the magnetic axis due to the large orbits and in the edge pedestal region, also due to the strong radial gradients. This leads to the need of developing models and corresponding codes which are not based on the local ordering. Codes with radially global properties, like XGCO [67] and XGCa [68], KEATS [69], PERFECT [70], and IMPGYRO [71] can be applied to these purposes, particularly for the plasma periphery, however they do not include rotational effects. Also global gyrokinetic codes which are usually applied for turbulence simulations but which also have sufficiently sophisticated multi–species collision operators [72–74] can be applied for global neoclassical calculations. Examples of these codes that have recently also addressed the problem of neoclassical transport are GYSELA [75] and GT5D [76]. These codes can also address the problem of the concomitant calculation of neoclassical and turbulent transport, and the direct interaction between the two transport mechanisms. These effects will be reviewed in section 4. Recent studies on global effects [77, 78] have demonstrated a collisional convection which is provided by the combination of an inward pinch connected with the main ion density gradient and a temperature screening term, consistent with the local theory. The strength of the temperature screening has been recently subject of investigations, and it is found in agreement with local theory when global simulations also reproduce the parallel ion heat flux [62].
3. Turbulent transport
The theory of turbulent transport of impurities can be developed in a way which is completely analogous to that of the main ion species [16, 79]. There is however an essential difference between impurities and main ion species. Excluding extreme and usually transient conditions in which impurities are present in very large concentration and/or exhibit very strong gradients, the impurity particle species are not the cause of the micro-instabilities which are at the source of the turbulent transport. This implies that in several situations the transport of the impurities can be well approximated by the trace limit, in which the impurities have negligible role in the quasi-neutrality equation, that is in the dispersion relation of the micro–instability. We observe that while in collisional transport the negligible impact of collisions with an impurity species is described by the parameter , where the impurity charge squared reflects the dependence of the collision frequency, in turbulent transport the impurity species becomes negligible in the micro–instability dispersion relation, that is in the quasi–neutrality condition, when
, which is more easily satisfied. However, micro–instabilities and turbulence which are more sensitive to the impact of collisions, like trapped electron modes (TEMs), can be modified by an increase of collisionality, which can be produced by the presence of impurities and which is proportional to
. We have to emphasize that most (if not the totality) of the codes that allow the calculation of impurity transport are not based on the trace limit, and thereby allow the computation of the transport considering the actual concentrations of the impurities, and thereby also the impact that their presence has on the micro–instabilities and turbulence. However, from the standpoint of providing a description of turbulent impurity transport, the trace limit has the practical property of having impurity particle fluxes which are linear in the impurity gradients. A specific study dedicated to the assessment of the applicability of the trace limit as compared to the inclusion of actual impurity concentrations shows that the trace limit is better fulfilled with ion temperature gradient (ITG) modes, whereas relatively lower charge concentrations of the impurities are required with TEM instabilities [80]. In general, if the impurity charge concentration and the impurity density gradients are small enough, which prevents the destabilization of impurity driven modes, the inclusion of the impurity charge densities in the quasi-neutrality condition (non-trace limit) only leads to small deviations from the linear dependence. In the codes (which regularly include all of the kinetic species in the quasi–neutrality equation), these can be taken into account by computing the transport coefficients around the logarithmic gradients of interest. This corresponds to a local linearization of the flux–gradient relation, which is usually largely applicable in the context of impurity transport even outside the trace limit. Beyond the trace limit, that is, in finite concentrations, impurities can also impact the microinstabilities and the turbulence. Usually the main effect is that of a stabilization through dilution, since impurities replace part of the main ions which in contrast contribute to the destabilization of the micro–instability, particularly in the case of the ITG (e.g. [81, 82]). However, depending on the shape of the impurity density the effect can change and become destabilizing for radially hollow impurity density profiles [83], also through the destabilization of an impurity mode at sufficiently high impurity concentrations and gradients. Impurities can also affect the stability through an increase of the collisionality, particularly stabilizing the TEM, and thereby in general the quantitative prediction of the impact of impurities on the microinstabilities and the turbulence depends on various parameters and requires specific calculations [84]. The topic of the impact of impurities on the micro–instabilities and turbulent transport of the main plasma however is not considered to be part of the present review and the reader who is interested on these aspects is suggested to consider the introduction sections of these recent publications [85, 86].
First studies of impurity turbulent transport have been performed by means of a fluid quasi–linear description [82], within the framework of the Weiland transport model [87]. Interest in turbulent impurity transport from the theoretical perspective has then remained very limited from more than one decade, while several experimental studies started to clearly document that at least light impurities were not exhibiting transport levels consistent with the neoclassical expectations, as reviewed in [88]. Then a series of works have started to shed light on the theoretically predicted properties of turbulent impurity transport [89–94], mainly following the approach and the already ongoing interest that was present in turbulent particle transport [95, 96]. A complete decomposition of the turbulent impurity flux in all of its main transport mechanisms required the additional inclusion, and the physical understanding, of the effect coming from the presence of a toroidal rotation gradient and a toroidal rotation [97]. This was ignored in those previous works and its identification was largely connected with the growing interest at that time of the properties of toroidal momentum transport and the existence of a toroidal momentum pinch [17, 98]. The problem of the impact of radio–frequency fields was first considered investigating the the effect of a radio–frequency ponderomotive force [99]. A quasi–linear analytical model has been also derived with a gyrokinetic approach in the electrostatic limit [100], and the properties of impurity turbulent transport have been studied in the presence of ITG [100] and TEM [101] instabilities. The dependence on the main ion mass has been analysed in [102] in relation with the transport properties of the main plasma, as well as the impact of a poloidal asymmetry of the electrostatic potential produced by a species with temperature anisotropy, like a resonant species in the presence of ICRH, a problem which was already examined in [103] and in [104]. Concomitantly, the role of poloidal asymmetries of the impurity density has been investigated analytically [105] and the role of centrifugal effects completely characterized with both analytical and numerical calculations [106], making use of the gyrokinetic code GKW [107] in which rotational effects were in the meanwhile included [108]. Finally, electromagnetic effects were investigated in [109, 110], where both works have demonstrated a reduction of the impurity turbulent diffusion with increasing normalized plasma pressure β. This brief historical survey of the first investigations on turbulent impurity transport (although partly selective, a more complete review will be provided below) allows us to underline the clear scientific connections that are present among the research on turbulent impurity transport and the research on the particle transport of the main species and the turbulent toroidal momentum transport. These are all characterized by a very strong role of the off–diagonal transport components [19].
A quasi–linear treatment can be performed from both the fluid and the kinetic approaches and allows us to identify all of the main components of the turbulent impurity flux. A complete treatment from the fluid standpoint, which extends previous works in [82, 90, 91] and also includes the impact of a toroidal rotation gradient, can be found in [97]. Within a kinetic description, a complete decomposition of the impurity turbulent flux can be found in [106] where also centrifugal effects are included, and thereby also the consequent possibility of a poloidally inhomogeneous impurity density distribution. Specific derivations leading to expressions which have more direct analytical applicability, but in which the effect of roto-diffusion is neglected, can be found in [94, 100]. For heavy and highly charged impurities like W, these centrifugal effects can have a non–negligible impact on the turbulent impurity transport at the largest Mach numbers achieved in present tokamaks. In contrast their effect is usually negligible for light impurities (e.g. Ar or lighter). In the present review we have opted for a treatment where centrifugal effects are omitted. This is justified by considering the otherwise significant complication in the mathematical treatment in combination with the fact that experimental conditions where these effects are important for the turbulent transport are also characterized by a strong increase of the neoclassical transport (also by centrifugal outboard localization of the impurities). Then, in these conditions, neoclassical transport usually provides the dominant convective component, reducing the relative importance of the centrifugal modification of the turbulent convection.
3.1. Analytical derivation of diagonal and non-diagonal components of turbulent transport
A general expression of the turbulent impurity particle flux can be formally derived from the gyrokinetic equation, where we also consider the presence of the radial gradient of the toroidal rotation, while still considering the limit in which the impurity toroidal rotation is small compared to its thermal velocity (that is, we neglect centrifugal effects). As already mentioned, a more complete treatment which also includes the impact of the centrifugal effects can be found in [106], where the formulation of the gyrokinetic equation in the plasma co-rotating frame [111] has been adopted.
Here below we derive gyrokinetic expressions of the particle and heat fluxes of a species σ, where we single out the dependence on the particle charge Z. We consider the linearized gyrokinetic equation for the perturbed distribution function
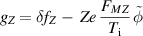
of a species with charge Z and mass A, and a Maxwellian equilibrium distribution function FM
Z
with temperature . Here
is the perturbed electrostatic potential. We consider a single toroidal mode number n = ky
r/q in Fourier space, with r the local minor radius, q the local safety factor and ky
the wave number binormal to the magnetic field, in simple s − α geometry. For simplicity, in the analytic model we consider the electrostatic collisionless limit, neglect centrifugal effects, and focus on the elements of the gyrokinetic equation which are essential to identify the main properties of the diffusive and convective terms. The gyrokinetic equation for the amplitude of the Fourier mode
, with
, reads
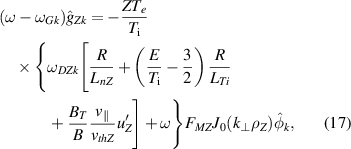
where the generalized gyro–center frequency contains both parallel and drift gyro-center motion

and where the parallel wave number is considered to be a number (and not an operator) in this formal derivation. Here
is the energy of the gyro-center, with
the magnetic moment, the fluctuating electrostatic potential is normalized as
and the normalized toroidal rotation gradient is
, with Ω the plasma toroidal angular velocity. For simplicity, collisions have been neglected in this analytical description since they do not alter the decomposition of the turbulent particle flux that follows. The gyro–center motion is given by
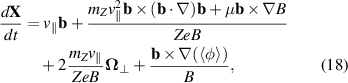
where in the order we can recognize the parallel motion, the curvature and grad B drifts, the Coriolis drift due to the plasma angular velocity , and the E × B drift (non–linear term). Second order terms in the background plasma rotation arising from the centrifugal drift have been omitted (again, general derivation in [106]). In this geometry the expression of the drift frequency is
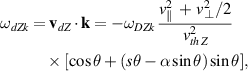
where we have introduced a generic (fluid) drift frequency defined as and where here
. By formally solving the gyrokinetic equation and adopting usual definitions of fluxes produced by the fluctuating E × B drift, we find formal analytical expressions of the impurity particle flux

As shown in [109], the impurity transport produced by magnetic flutter is usually negligible, due to the small thermal velocity of heavy impurities, and it will not be specifically discussed here. In contrast, electromagnetic effects can affect the E × B transport, in particular reducing the impurity turbulent diffusion in ion temperature gradient (ITG) turbulence [109, 110].
From the above expression we obtain the usual decomposition of the impurity particle flux,

where the coefficients DnZ
, DTZ
, DuZ
and VpZ
represent impurity turbulent diffusion, thermo-diffusion, roto-diffusion and pure convection respectively. We recall that equation (20) expresses a linear relation in the case of trace impurities, also in nonlinear simulations, however it requires a local description to be rigorously satisfied [112]. It is practical to also define normalized coefficients defined as ,
, and
for thermo–diffusion, roto-diffusion and pure convection respectively. These can be more directly computed in quasi-linear models in particular. The different contributions to the flux will be reviewed in the following subsections.
3.2. Impurity turbulent diffusion
In order to investigate the nature of the turbulent impurity diffusion, it is instructive to compare its kinetic expression with the corresponding expression of the heat conductivity [113]

and
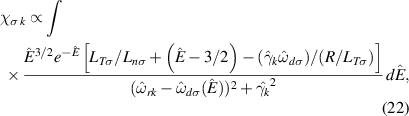
where the species index σ refers to both electrons e and main ions i. In equations (21) and (22) we have neglected parallel ion motion and we have introduced the energy only dependent drift frequency , where
. Equation (22) provides the expression of the heat conductivity for both main species electrons and ions, with
. Following [113], we observe that the condition which maximizes the heat conduction of the main species (electrons and main ions) does not correspond to the condition that maximizes the impurity diffusion. Indeed not only the denominators are different (
and 1 for the main species), but also the conduction and diffusion correspond to different energy moments, and thereby the different powers of the energy in the numerators weigh in a different way the impact of the energy dependent resonance provided by the denominator. The role of the relevant resonance produced by the type of turbulence, and thereby the value of the real frequency of the unstable mode resulting from the solution of the dispersion relation is of fundamental importance in turbulent transport. This aspect has been also pointed out in connection with the different size of ion and electron particle diffusion and convection in different turbulence regimes, leading in particular to much larger values of the diffusion and the convection of the ions than those of the electrons in ITG turbulence [114, 115]. Analogously, when considering the ion heat conductivity in the presence of ITG turbulence, we observe that this is maximized in the presence of a large and positive (ion drift direction) real frequency of the unstable mode, as it results from the solution of the dispersion relation for parameters delivering a strong ITG mode. In contrast, at the same ion charge (Z = 1), the particle diffusivity is maximized by smaller values of the real frequency, due to the weaker energy dependence of the numerator. If we consider a highly charged impurity (Z
1), then the drift frequency at the numerator is practically removed by the large value of Z, and the resonant condition is simply obtained when the mode frequency is close to zero. An analogous argument can be developed for trapped electron mode turbulence, where a large and positive mode frequency produces a large heat transport, but again a small impurity particle transport. The maximum reachable value of
increases with increasing charge at low charge values of the impurity (
) and already reaches the
asymptotic value for
. In conclusion, it is evident that conditions for which the impurity diffusion coefficient is large correspond to conditions where the mode real frequency is neither strongly positive or strongly negative, but in fact where the mode frequency approaches zero. These conditions are obtained when the ion and electron heat fluxes are of comparable magnitude, what suggests that the ratio of the impurity diffusion to the main plasma effective heat conductivity
is a non–monotonic function of the electron to ion heat flux ratio. This result is indeed confirmed by a set of non–linear gyrokinetic simulations [113] which find that the impurity diffusivity to heat conductivity ratio can vary by more than an order of magnitude and is maximum when
. This theoretical result can provide at least qualitative explanation of early observations of a strong increase of the impurity diffusivity in response to the addition of central electron cyclotron resonance heating (ECRH) [116]. It has also found at least qualitative confirmation in a very recent experimental study [117], although the experimentally estimated values of the impurity diffusivity featured variations which are even larger than those which are theoretically predicted. In particular, significantly lower values of the minimum of
than those predicted have been obtained, whereas maximum values were found to be more consistent with the theoretical predictions and as large as
. A clear demonstration of a drop of the
ratio at values of
has not been obtained so far, but would provide an additional important piece in the theory validation.
Given the common nature of the properties of both the denominator and the numerator in the expressions of the impurity diffusion and convection coefficients and the main species heat conductivities, the property of being maximized when the turbulent electron and ion heat fluxes are of comparable size is also shared by the convective terms [118]. We start now by examining the thermo-diffusion term. The directions of the convective components are given considering usual monotonically increasing safety factor profiles. If the magnetic shear is negative enough or the α parameter large enough to change the sign of the curvature and grad B drift, all of the terms connected with the compression of this drift reverse their direction.
3.3. Turbulent thermo-diffusion
The turbulent thermo-diffusion is an interesting convective term which is analogous to the corresponding term produced by the logarithmic temperature gradient for electron particle transport [79]. The dominant component of this term stems from the toroidal resonance [90, 94], which, analogously to the electron thermo-diffusive convective part, is characterized by having its radial direction connected to the direction of propagation of the turbulence. A detailed physical explanation at the basis of this thermo-diffusion mechanism can be found in [19] and will not be repeated here. As kinetic integral it is given by
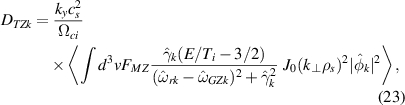
from which one can directly realize that in the absence on an energy dependent denominator it would be zero, because . Thereby, the existence of this convective term is directly connected with the energy dependence present at the denominator, which is included in the energy dependence of the gyro-center drift. We consider the curvature and grad B drift resonance first. Because the curvature and grad B drift is proportional to 1/Z and thereby reverses direction from electrons to ions, the direction of the turbulent thermodiffusion is also opposite for electrons and ions. Another connected property is that it reverses direction with the type of turbulence, if turbulence is propagating in the ion or the electron drift direction, ITG and TEM respectively for instance. This is produced by the sign reversal of the kinetic dependence
, which can lead to an overall positive or negative net thermo-diffusive flux depending on whether the resonant real frequency enhances the contribution at low (inward) or high (outward) energies [19]. In the presence of ITG turbulence, the thermo-diffusion of ions and impurities is directed outward, whereas it is directed inward for the electrons. Thereby, in ITG turbulence thermodiffusion leads to a flattening of the impurity density profile with increasing strength of the ion temperature gradient. With increasing impurity charge, the energy dependence of the denominator is removed (or, in other words, the resonant conditions moves at energies which are very far from the
), and thereby the kinetic integral increasingly approaches the unperturbed situation of
. Indeed, with increasing impurity charge the thermo-diffusion decreases as 1/Z and, compared to the other convective terms, becomes practically negligible for
, whereas it is independent of the impurity mass. These dependencies can be also obtained with a fluid description [82, 90, 91]. Moving now to the slab resonance provided by the parallel motion term
at the denominator, this is charge independent and does not imply a change of sign along the energy axis and thereby provides a contribution which has the same inward direction for both ions and electrons and in both electron and ion propagating turbulence [79]. It is inversely proportional to the impurity mass.
3.4. Turbulent roto-diffusion
The roto-diffusion term is caused by the presence of a radial gradient of the toroidal rotation. Its existence has been first pointed out within a fluid treatment and confirmed with gyrokinetic simulations [97]. The analysis of its kinetic integral reveals that it is directly connected with the same mechanisms leading to parallel momentum transport

It is equal to zero in the absence of a background toroidal rotation (Coriolis drift) or of another symmetry breaking mechanism leading to a finite (which can be also directly provided by a finite rotation gradient
). In contrast, when these terms are present, they produce a direct coupling between parallel velocity fluctuations and density fluctuations, leading to radial particle transport. The mechanisms producing roto-diffusion have complete analogy to those which lead to Coriolis momentum pinch [98] and to the intrinsic rotation. In general, the dominant component comes from the presence of a background toroidal rotation in combination with the toroidal (curvature and grad B) resonance, and it is proportional to A/Z. Thereby, it can remain substantial also for heavy and highly charged impurities, differently from the thermo-diffusion term. Its direction also depends on the direction of propagation of the turbulence, and it is directed outward in the case of the ITG turbulence, in the same direction as the thermo-diffusion term. Plasmas characterized by strong NBI heating and developing strong rotation and ion temperature gradients produce thermo-diffusive and roto-diffusive transport contributions which are both directed outward and which concomitantly lead to a flattening of the impurity profile. This predicted dependence has received some qualitative and in some cases even quantitative experimental validation [119–122]. In the limit of the slab resonance only, roto-diffusion is directed outward and is charge and mass independent. It is also modified by neoclassical modifications of the equilibrium distribution function [123], as it will be more specifically discussed in section 4. Finally, also the problem of the impurity transport produced by the parallel velocity gradient instability in slab geometry with weak magnetic shear has been considered with an analytical quasi-linear model [124], where the properties of both diffusion and convection have been separately studied.
3.5. Turbulent pure convection
We call pure convection a contribution to the particle flux which is not proportional to any gradient of any kinetic profile. It can be identified in the expression for the particle flux equation (19),
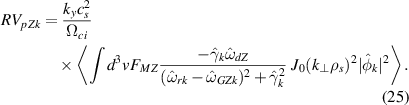
The main mechanism of turbulent convection is connected with the inhomogeneity of the magnetic field, leading to the compressibility of the E × B flow. Thereby it is charge and mass independent, and gives a contribution which is directed inward and in simplified geometry in the strong ballooning limit simply equal to Cp
=−2 (where the −2 stems from the sum of the curvature and grad B drift components, each one providing a contribution of −1/R, and we recall that the dimensionless coefficient Cp
is given by the ratio of the pure convection to the diffusivity , equation (20)). This mechanism is completely analogous to that present for the main plasma [87, 96] and in the past was also interpreted in terms of turbulent equipartition [125]. This E × B compression term is already present in the impurity transport formulation presented in [82], in combination with the thermodiffusive term, and has been also identified in gyrokinetic simulations of He transport [89]. As already anticipated, with reversed magnetic shear this impurity convection reverses sign from inward to outward [126]. An additional contribution to the convection comes from the slab resonance and the parallel dynamics [90, 94]. This component is interestingly reversing direction depending on the direction of propagation of the turbulence, being directed inward in the case of ITG turbulence, and outward in the case of TEM turbulence. It is proportional to the charge to mass ratio Z/A of the impurity and thereby can remain finite also for highly ionized heavy impurities. Analogously to the other terms connected with the slab resonance, it is proportional to the square of the parallel wave number and therefore in general it also increases with decreasing value of the local safety factor. An indication of the impact of this effect on the peaking of the impurity density profile in the central region has been obtained in specific gyrokinetic modelling of ASDEX Upgrade plasmas with central electron heating [127]. Its role has also been confirmed in nonlinear gyrokinetic simulations of He transport, where the He pure convection has been found to move from inward to outward with increasing electron to ion heat flux ratio [112].
Finally, we recall that also in the case of turbulent transport, modifications of the impurity fluxes are produced in the presence of poloidal density asymmetries of the impurities, although these are less important with respect to neoclassical transport. With increasing impurity toroidal rotation, centrifugal effects mainly provide a large positive (outward) addition to the thermodiffusion which is offset by a corresponding large but negative (inward) addition to the roto-diffusion and a small outward component to the pure convection [106]. While the final result can depend on the actual size of these different components, depending on the local parameters, it usually provides a small inward additional net contribution to the convection, leading to a slightly increased logarithmic gradient of the impurity density profile at the low field side. All of these effects can be largely described by means of a simplified model in which the fluxes are computed taking into account these density asymmetries, while the asymmetries are neglected in the actual solution of the dispersion relation [106]. This simplified approach has been more recently included in the QuaLiKiz transport model, where the turbulent transport of impurities has been generalized to the situation of poloidally asymmetric conditions also including the impact of temperature anistropies [128] (specifically in appendix C). The problem of the impact of the presence of a species with temperature anisotropy, such as a resonant minority species with ICRH, on the turbulent impurity transport has been examined in [102–104]. This effect has been also included in the gyrokinetic code GKW [50], where it is applied following the model for impurity poloidal asymmetry proposed in [45].
3.6. Additional effects which impact turbulent impurity transport and specific comparisons with experiments
As anticipated in the previous subsection, electromagnetic effects can also impact the turbulent transport of impurities. Magnetic flutter usually provides a small to negligible contribution to the total flux, due to the low thermal velocity of the impurities, while the modifications that finite β effects have on the growth rate and frequency of the unstable modes can modify the E × B transport [109, 110]. The behaviour of the impurities when entering a regime with dominant kinetic ballooning modes (KBMs) has been specifically investigated in [110], and found to result in small values of turbulent convection, mainly leading to flat predicted impurity density profiles. Indications that KBM are the dominant instability close to the magnetic axis in H–mode plasmas have been recently obtained by means of gyrokinetic simulations [129], a result that could motivate renewed consideration on the properties of impurity transport in the presence of this type of turbulence. Comparisons among the results of nonlinear gyrokinetic simulations and the predictions of gyrokinetic and/or fluid quasi-linear results have been studied as a function of magnetic shear [93], as well as in TEM and ITG turbulence driven by temperature gradients [130] and in TEM turbulence driven by the density gradient of the main plasma [131]. Consistency between the quasi–linear results and the results from the nonlinear simulations has been found in general although gyro-kinetic quasi-linear results exhibit a slightly stronger dependence on the impurity charge as compared to the nonlinear results. Consistently, in both nonlinear and quasi-linear results, the increase of impurity charge implies an increase and a decrease of the predicted impurity peaking factor in ITG turbulence and TEM turbulence, respectively. This difference can be understood through the opposite sign (outward in ITG and inward in TEM) of the thermodiffusion component, which decreases with increasing charge as presented in section 3.2. Density gradient driven TEM turbulence exhibits impurity density gradients which are weaker than those of the bulk plasma. Consistently with the general properties of impurity turbulent transport, also in these studies [130, 131], the impurity peaking predicted by turbulent transport only remains significantly smaller than the peaking provided by neoclassical transport alone. Further comparisons in the verification of a quasi–linear fluid turbulent transport model against nonlinear gyrokinetic simulations have been performed in [132] where both the electron and the impurity density gradients were consistently obtained from the condition of zero particle fluxes. The low collisionality, turbulence generated, density peaking of the bulk plasma [95] was regularly found to be stronger than that featured by the impurities, confirming the favorable behaviour for bulk plasma and impurity density profiles of low collisionality reactor–like conditions, which are dominated by turbulence. An analytical model for quasi-linear impurity transport has been developed in [100]. This approach appears promising for extensions towards a more complete description for fast applications in transport modelling. Companion studies have been devoted to impurity transport in the presence of TEM [101]. At the transition between ITG and TEM turbulence, more sophisticated effects can occur, which lead to nonlinear results in quantitative disagreement with quasi–linear results that are based on the dominant linear mode only [118]. This property can be understood as a result of the effect of subdominant linear modes, which can determine the dominant properties of the impurity turbulent convection in the case the dominant linear mode has a turbulent convective component which is very small. In these peculiar but experimentally still relevant conditions, quasi-linear models based on the computation of transport combining the effects of both dominant and subdominant linear modes provide results which are closer to the nonlinear results.
The impact of the inclusion of an accurate description of the plasma shape through a numerical magnetic equilibrium solution in comparison with simplified analytical descriptions has been investigated in [133]. Regarding the electromagnetic effects connected with fluctuations of the magnetic field and produced by an increase of the normalized plasma pressure β, with usual ITG modes at sufficiently large mode frequency, the reduction of the growth rate, which is obtained when the threshold for dominant kinetic ballooning modes (KBMs) is approached, leads to a significant decrease of the impurity diffusion coefficient. This reduction is larger than that of the corresponding main ion heat conductivity [109, 110, 134]. This result is also consistent with [113], where the reduction of impurity diffusivity by finite β has been also confirmed in nonlinear gyrokinetic simulations. The effect is practically absent in the case of TEM instabilities, since these are weakly modified by finite β. The impact on the convection is usually weaker, and determined by a more complex combination of multiple effects in the various convective components. In general, however, in the presence of ITG modes at sufficiently large mode frequency, a reduction of the growth rate leads to a stronger relative reduction of the pure convection term with respect to thermo-diffusion and roto-diffusion, which in contrast have a relatively increased effect. Since in these conditions the pure convection is directed inward, whereas both thermo-diffusion and roto-diffusion are directed outward, the reduction of the growth rate can lead to an overall less inward or more outward total convection of the impurity. This effect on the impurity transport has been specifically analyzed also in combination with the stabilization produced by the inclusion of a population of fast ions, like that produced by neutral beam injection heating [134]. As already mentioned, effects connected with the presence of fast ions with temperature anisotropy have been also studied in relation with the consequent development of poloidal asymmetries of the electrostatic potential and the impurity density [102–104]. An overall outward effect in the convection is predicted by these works, which are more specific to the role of ICRH resonant minority ions. The combination of these effects in connection with the presence of beam ions has not been investigated yet to our knowledge. The impact of the consequent poloidal asymmetry of the electrostatic potential on the poloidal distribution is however an exponential function of the impurity charge [45], and therefore becomes negligible for light impurities, for which turbulent transport is usually dominant.
Comparisons of impurity turbulent transport predictions with experimental observations are better performed on light impurities, which have lower levels of collisional transport. First attempts were mainly limited by relatively incomplete theoretical descriptions, in particular still ignoring the presence of roto-diffusion (e.g. [127, 135, 136]), and/or affected by the neglect of the impact that poloidal asymmetries can have on the neoclassical transport component of heavy impurities (e.g. [137]). In one of the first comparisons between dedicated experiments and theoretical predictions, an increase of the central diffusivity of nickel was observed in Tore Supra [138] with increasing electron temperature logarithmic gradient in the central region of the plasma, well beyond the neoclassical levels and consistent with theoretical quasi–linear calculations of impurity diffusivity, as produced by the turbulence destabilization. Additional important steps towards the validation of turbulent transport predictions of impurity transport with experimental observations have been performed in [119, 139] with complementary approaches. The former study compares quasi–linear gyrokinetic predictions with a set of measured density profiles of intrinsic boron, demonstrating the non-negligible importance of the inclusion of roto-diffusion [97] in recovering experimental trends produced by a variation of the electron heating fraction in the presence of background NBI heating, although not reaching a full quantitative agreement. The latter compares, for the first time, global non–linear gyrokinetic simulations with the gyrokinetic code GYRO [140], matching the heat fluxes, with the separate measurements of the diffusion and convection coefficients of a Ca impurity injected in dedicated laser ablation experiments [141]. The comparison provides an unprecedented demonstration that, within the experimental uncertainties, the gyrokinetic predictions were able to reproduce the experimentally estimated values of the impurity transport coefficients. A careful study of the experimental uncertainties was also performed in [121], where the impact of varying plasma toroidal rotation on the measured intrinsic boron density profile was compared to the results of both linear and non–linear gyrokinetic simulations. Within uncertainties a quantitative consistency was possible, although evidences of disagreement at the margin of the uncertainties were observed in the presence of strong NBI heating and strong plasma rotation, with observed hollow boron density profiles being predicted with lower level of profile hollowness. Similar results were also obtained in analogous validation works at JET, consistently documenting the incapability of the gyrokinetic modelling to reproduce the observed hollowness of the intrinsic carbon density profiles [133, 142]. The consistency between predictions from a fluid model and the gyrokinetic results were also verified in these works, and the predicted dependence of the density peaking of injected impurities around mid-radius has been found to be in qualitative agreement with the experimental observations, with an increase of the peaking factor with increasing impurity charge for Z
20 and a saturation to constant values for larger values of Z, much below the neoclassical predictions, consistent with a charge independent scaling of impurity transport at large charge, as explained in the previous section.
The inconsistency with experimental observations of hollow intrinsic impurity density profiles was also found in more recent studies at JET C–Wall [143] and JET–ILW [144], where the gyrokinetic simulations were found to predict weakly peaked impurity density profiles in conditions where the experimental observation had flat or hollow profiles. The increasing evidence of a disagreement between predictions and observations in these conditions has concomitantly motivated further experimental investigations not only on intrinsic impurities profiles [145] but also on injected impurities with transient transport experiments, separately determining diffusion and convection [122, 146], with comparisons with both quasi-linear gyrofluid and quasi-linear and nonlinear gyrokinetic simulations respectively. In [146], dedicated to high confinement regimes at DIII–D without ELMs, cases of agreement between theoretical predictions and experimental observations where mainly identified in the convective term, whereas evidences of disagreement were reported on the diffusivities. In [122], an ECRH and NBI heated H-mode plasma at ASDEX Upgrade was found to have boron diffusion and convection coefficients quantitatively consistent with the gyrokinetic predictions within experimental uncertainties.
Systematic comparisons over databases of observations in ASDEX Upgrade [145] and JET C-Wall [143] H-mode plasmas provided important informations in the identification of parameter domains in which the disagreement between theory and experiment was particularly large. In JET [143] the disagreement was found to be particularly large at high collisionality. At ASDEX Ugprade a new framework for the evaluation of impurity densities based on measurements from charge exchange recombination spectroscopy was recently developed [147]. In this device the disagreement was documented to be particularly large at high ion temperature logarithmic gradient and rotation gradients [145], correlating with large NBI heating power. The ASDEX Upgrade cases with higher ion temperature and rotation gradients were close to the JET parameter space investigated in [143] and confirmed the overprediction of impurity density peaking found in that previous work.
These evidences of disagreement motivated in particular the investigation of the direct impact of a neoclassical equilibrium on the turbulent impurity transport [123] and, more recently, the impact of beam ions [134]. While the former study has identified only minor effects, which become negligible for light impurities at core plasma conditions, the latter study has clearly identified an important additional ingredient which was missing in the modelling activity performed so far and which leads to a strong reduction or even reversal of the convection at high values of beam ion density and gradients, due to a combination of effects involving both turbulent and neoclassical transport. Further comparisons between theoretical predictions including the impact of beam ions and experimental observations are currently being performed in order to conclusively assess whether the inclusion of the impact of beam ions is the only critical ingredient that was still missing in order to bring theoretical predictions and experimental measurements to agree within uncertainties. The difficulty in quantitatively predicting the convection of the impurity transport has been confirmed by a recent study in C-Mod [148], where theoretical predictions have been compared with calcium impurity density profiles as well as separate diffusive and convective transport coefficients experimentally inferred by means of a fully Bayesian approach. Clear observations of outward impurity convection and hollow density profiles have been obtained which cannot be reproduced by quasi–linear and non–linear gyrokinetic simulations [149]. These results suggest that while beam ions certainly are an important ingredient, they could not be the only element which is still missing in the theoretical description to reliably reproduce the occurrence of hollow density profiles of light impurities. Very recently, theoretical studies with a linear gyrokinetic model solving for multiple eigenmodes have been also dedicated to the possibility that impurity modes [82, 83, 150] produced by hollow impurity density profiles can sustain a turbulent screening effect [151]. If impurity modes are destabilized, what requires sufficient impurity concentration in combination with a sufficiently large reversed impurity density gradient, these modes could produce the dominant turbulent impurity flux, even if the impurity modes are linearly subdominant with respect to the temperature gradient driven instabilities. In these conditions, impurity modes can sustain hollow impurity density profiles in the presence of sufficiently large electron and ion temperature gradients [151]. Transient destabilization of an impurity mode has also been obtained in the transport modelling of impurity laser ablation experiments with a gyrofluid transport model [152], in this case producing a large impurity flux directed inward.
The impact of a change of main ions, such as a hydrogen isotope, on the impurity transport is a topic which has received limited consideration so far in the comparison between theoretical predictions and experimental observations. From the experimental standpoint it is challenging to isolate pure isotopic effects, that is only deriving from a change of mass of the main ion, on the impurity behaviour from effects which are connected with the consequent changes in plasma parameters. These are also produced by a change of the main ions and could be dominant. Direct isotopic effects can be isolated in theoretical studies. An analytical quasi–linear transport model has been applied to investigate the differences in impurity transport produced by a change of main ions, considering hydrogen, deuterium and tritium, in the presence of ITG modes in a simplified sheared slab geometry [153]. The results show that the change of hydrogen isotope has limited effects on the impurity diffusion and some effects on the impurity convection, in particular with a different behaviour for light, low-Z and heavy, high-Z impurities. An increase of main ion mass implies a reduction of outward impurity convection for light impurities, and an increase of the convection for heavy impurities. More studies on this topic could certainly be of interest, where nonlinear effects can also be important, in particular considering initial operation in H and possibly in He in ITER.
Finally, a specific paragraph has to be dedicated to the important topic of the density profiles and the transport of He as an impurity. Past observations of He density profiles as impurity in deuterium plasmas revealed that He density profiles are very similar to the profiles of the main plasma electron density and with He diffusivities which are comparable to the effective heat conductivity [154]. More recently, applying a specifically developed kinetic forward model for the He plume emission [155], the properties of the He density profiles have been characterized in a dedicated study at ASDEX Upgrade [145], which largely confirmed past results, and documented that the shape of the He density profiles follows that of the electron density, but becomes slightly less peaked than the electron density in conditions of large NBI heating fraction. All of the experimental investigations robustly confirm the absence of He accumulation in the plasma center in all the explored confinement regimes, qualitatively consistent with the general concept that transport of low charge impurities is dominated by turbulence and turbulence is theoretically predicted to not produce central accumulation. Recently, from the modelling standpoint, the transport of He has been specifically considered in [89, 112]. The former work focused on the mechanisms leading to the formation of inward convection, with the support of gyrokinetic simulations. The latter work studies the properties of He transport in combination with those of α particles particularly around the operational point provided by the ITER baseline scenario. The He diffusivity is found to be about
in these conditions, which have an electron to ion heat flux ratio around 1. Diffusion coefficients of He which are equal or larger than the effective heat conductivity have been also found in ITG [142] and TEM turbulence simulations [130], with the TEM turbulence providing the larger values. Anomalous values of diffusion and convection of He have been measured in dedicated experiments in MAST [156] L–mode plasmas, with the observed He peaking factor in good agreement with quasi–linear gyrokinetic predictions in conditions of dominant TEM turbulence. More in general, the ratio
follows the dependence on the electron to ion heat flux ratio which has been pointed out in [113] and reviewed in section 3.1, which also reflects the impact of varying the dominant turbulence conditions from ITG to TEM [114]. Moving from dominant ITG turbulence to conditions of mixed ITG and TEM turbulence is predicted to increase the He diffusivity. Large values of He turbulent diffusion in collisionless TEM turbulence have been recently reported in a dedicated theoretical study, by means of an analytical model [157], with a particularly favorable role of the electron temperature gradient for the removal of He ash. In strong TEM turbulence however the He diffusivity becomes smaller than that of the electrons [114]. Given the central role that the He diffusivity and its ratio to the heat conductivity play in determining the ratio of the He confinement time to the energy confinement time and thereby also the requirements in the He exhaust system in a burning plasma [158, 159], a more complete validation of the theoretical predictions for this critical parameter against accurate experimental measurements should certainly be considered a present priority. From the side of the convective terms of He turbulent transport, in a database of He density profiles at ASDEX Upgrade a systematic underprediction of the observed He density peaking is regularly found [134, 145], when the impact of NBI fast ions is also included. This underprediction of the peaking is also found in a comparison with 3He density profiles at JET [144]. The reasons behind this disagreement, as obtained from a theoretical model which at the same time provides correct quantitative predictions of other light impurity density profiles, are presently still unclear.
4. Direct interaction between turbulent and neoclassical effects
Neoclassical and turbulent transport of impurities are usually computed independently and added. This customary approach assumes that there is no direct interaction between the two transport processes, an assumption which has been regularly adopted in the impurity transport modelling [160]. This assumption is mainly based on the different characteristic time and spatial scales which are involved in the collisional and the turbulent transport processes. Such an assumption should only be considered as a sort of general approximation, because direct effects which couple collisional and turbulent transport are in fact always present, although they can have small to negligible impact on the impurity transport properties in the majority of usual plasma conditions. The most direct mechanism by which collisional transport can impact turbulent transport is through the consideration that in the presence of collisions, the distribution functions of the particle species which determine the plasma local equilibrium, are not given by unperturbed Maxwellian distributions, but also include neoclassical modifications leading to the neoclassical local equilibrium. This is in particular characterized by the presence of lowest order neoclassical flows, which directly impact the turbulent momentum transport [123, 161–165]. The concomitant impact of the neoclassical equilibrium distribution function on the impurity turbulent transport has been directly investigated in [123] with both a fluid analytical approach and a numerical approach through the coupling [165] between the drift-kinetic solver and neoclassical transport code NEO [53–55] and the gyrokinetic turbulent transport code GKW [107]. Analogously to their impact on the momentum transport, the presence of the neoclassical equilibrium flows impact the impurity transport mechanism connected to roto-diffusion. One effect is directly produced by the parallel symmetry breaking introduced by the neoclassical flows, in complete analogy with the turbulent momentum transport mechanism [161]. An additional effect, more subtle but dominant for turbulent impurity transport, is connected with the velocity integrals which involve the parallel velocity times the distribution function of the impurities, and which produce finite contributions due to the presence of the neoclassical perturbation. These terms directly lead to roto-diffusion, even in the absence of a direct coupling between density fluctuations and parallel velocity fluctuations. Conditions of high collisionality and heavy impurities are required for these effects to become non–negligible and experimentally measurable. Interestingly, this roto-diffusive radial transport, produced by the neoclassical equilibrium, can reverse direction with the signs of the plasma current and of the magnetic field.
The direct impact that turbulence can have on neoclassical transport has been recently investigated in the framework of global gyrokinetic simulations [166–168]. These investigations have been mainly performed making use of the global gyrokinetic code GYSELA [75], where a multi-species collision operator has been recently implemented [73, 74] which allows the simultaneous computation of neoclassical and turbulent particle fluxes, in particular opening the possibility to the investigation of the direct interactions between the two transport mechanisms. In general, it can be expected that whenever turbulence modifies the plasma state over scales which go beyond the microscopic scales, and on time scales which are not those of transport, a direct interaction between turbulence and neoclassical transport becomes possible. Then this direct interaction cannot be accounted by the calculations of the two transport processes separately. In other words, modifications of plasma profiles which are produced by turbulence on a transport time scale have an indirect impact on neoclassical transport, which can be computed independently from possible direct interactions between the two transport mechanisms. However, when turbulence produces modifications of the macroscopic properties of the plasma on time scales which are shorter than those of the transport time scales, for instance with the development and sustainment of meso-scale structures [169–171] and of large scale poloidal asymmetries [166, 167], direct interactions between turbulent and collisional transport become possible, so that the total transport cannot be considered to be provided by the sum of the two effects computed separately. An additional cause of direct interaction can arise when required orderings for local transport of both collisional and turbulent transport are not satisfied [172]. In the framework of impurity transport, deviations from the neoclassical transport as computed independently from the turbulent transport have been found in simulations of heavy impurities like tungsten [62, 166]. The main cause of this direct interaction has been identified to be provided by turbulence driven poloidal asymmetries [62] and thereby presents a similarity with effects of poloidal asymmetries on neoclassical transport which are produced by macroscopic external means, as already described in section 2. Since this direct effect of turbulence on neoclassical transport is connected with the turbulent generation of poloidal asymmetries of the electrostatic potential, it is particularly important for high-Z impurities like W, which are more strongly affected by the consequent parallel electric field. The turbulent generation of poloidal asymmetries is directly connected with that of poloidal convective cells [173]. Two main mechanisms have been identified that generate poloidal convective cells [167]. One is connected with the flow compressibility, that results as a side-band effect in the pumping of axisymmetric (toroidal mode number n = 0) modes with poloidal mode numbers m =±1 by n = 0 and m = 0 zonal flows, by means of the flux surface averaged effect of the Reynolds stress. The second mechanism is related to the ballooning nature of the turbulence, which in turn produces a ballooning structure of the Reynolds stress. The latter mechanism prevails at very low frequencies, mainly producing in–out asymmetries, while the former mainly produces up–down asymmetries. Conditions where convective cells play an important role are likely connected to those where also zonal flows are strong. The experimental identification of these mechanisms as well as the validation of the predicted effects on high-Z impurity transport however remain difficult to obtain, since within uncertainties they can be hidden by other effects connected to macroscopically and externally generated poloidal asymmetries.
An additional mechanism of interaction between turbulence and neoclassical transport has been recently identified in simulations of ITG turbulence with the global full-f gyrokinetic code GT5D, including kinetic electrons, bulk ions, and low to medium charge tracer impurities [174]. Enhanced neoclassical particle transport is obtained due to a new synergy effect, connected with the transient development of non–ambipolar turbulent fluxes of electrons and bulk ions, generated by the bursty excitation of the ITG turbulence. This non-ambipolarity of the turbulent particle fluxes leads to a fast growth of the radial electric field following the ambipolar condition, which, in turn, leads to up–down asymmetric density perturbations by compression of the E × B flow and increased transport due to the magnetic drift. The enhancement of the neoclassical particle transport increases with increasing ion mass and only acts on the particle transport.
Global investigations of turbulent impurity transport have been also performed with the TERESA model and related code [175], a reduced 4D model for trapped electron and trapped ion modes. The properties of the impurity diffusion within this reduced trapped particle turbulence model have been investigated in [176]. Consistently with quasi-linear results and with previous publications based on a local model [90], the diffusivity of the impurities is found to increase with increasing charge in TEM turbulence and to decrease with increasing charge in trapped ion mode (TIM) turbulence, analogously to the behaviour in ITG turbulence. The properties of the convective terms produced by a temperature gradient, namely thermodiffusion, and by pure convection as produced by trapped particle turbulence within this model have been investigated in [177], where non–linear simulations are shown to be consistent with quasi–linear expectations and reflect the general properties described in sections 3.2 and 3.4 [90, 91], in particular the reversal of the thermodiffusion from outward to inward when moving from TIM to TEM turbulence. A recent work has also assessed the validity of the trace impurity limit in the framework of the TERESA model for trapped particle mode turbulence [178], showing a reduction of the turbulent transport for concentrations of W40 + above 2 × 10−4, and a complete quench for concentrations above 10−3, with the electric potential fluctuations becoming in phase with the impurity density fluctuations.
5. Review of theory-based modelling of impurity transport and impact of MHD activity
Various studies dedicated to the impurity transport modelling and to the comparison with experiments have been already considered in the previous sections, since usually these results play a central role in further motivating the theoretical developments in the overall validation process. At the same time, this review paper would be incomplete without a specific section dedicated to the modelling and validation, which is becoming an increasingly large part of the present research activities. The theoretical background presented in the previous sections shows that modelling requirements for accurate predictions are not the same for light and heavy impurities. Neoclassical transport is usually non-negligible for light impurities only close to the magnetic axis and in the H–mode pedestal, and it is not strongly affected by the small poloidal asymmetries that light impurities can develop. In contrast, neoclassical transport can play a dominant role in the correct prediction of the transport of heavy and highly charged impurities over the entire plasma profile, particularly in conditions of strong plasma rotation and high localization of the heavy impurities on the low field side. In general, moving towards the low collisionality of a reactor and in the absence of strong poloidal asymmetries of the electrostatic potential, it can be expected that turbulent transport becomes dominant also for heavy impurities, while neoclassical transport never becomes completely negligible. This brief introduction explains why in present experiments the validation of the turbulent transport properties can be more appropriately performed on observations on light impurities, whereas neoclassical transport effects, particularly in connection with poloidal asymmetries, have to be studied on heavy impurities.
The dominance of neoclassical transport for heavy impurities has been recognized since early works, and the properties of neoclassical transport in determining the observed central accumulation have been documented in many experiments, particularly in conditions of good energy confinement, in the core [116, 160, 179–187] and in the pedestal of H–modes [188–190], where turbulent transport is reduced.
A hierarchy of models can be applied for the theoretical predictions of the experiments. High fidelity models are characterized by more comprehensive physical descriptions, typically drift-kinetic and gyro-kinetic codes for neoclassical and turbulent transport respectively, but also imply heavier computational requirements. They are usually only applicable to the modelling of single time slices. In contrast, models which can be used in transport modelling workflows are usually a combination of analytical formulae or fast numerical codes for the neoclassical transport and multi–species quasi–linear turbulent transport models like TGLF [191–195] and QuaLiKiz [94, 128, 196]. The drift-kinetic code NEO [53–55] is fast enough to be also applicable in transport modelling [61], although it implies very long computational times.
One general aspect to be considered in the modelling of heavy impurities like W is that they are not fully ionized and thereby are present with multiple ionization stages. The fractional abundances of these stages can be computed with impurity transport codes like STRAHL [197–199] or SANCO [200], which make use of appropriate ionization and recombination data. However for the purposes of predictive transport modelling including collisional and turbulent transport it is regularly considered that to independently compute the transport of all the ionization stages would be computationally too demanding and largely unnecessary, while the turbulent transport coefficients are a very weak function of the impurity charge and the collisional transport does not depend more strongly than linearly on the impurity charge. Since for a given electron temperature the ionization stages which are significantly populated are usually on a relatively small interval of impurity charges (see e.g. [201] for W), it is practical and certainly sufficiently accurate to consider heavy impurities with one single representative charge number with the impurity charge as given by the local average over the fractional abundances of all the ionization stages.
Transport modelling of the dynamical evolution of heavy impurity accumulation was already performed in early works, efficiently combining simplified models for neoclassical transport in different collisional regimes [183]. In the pedestal of H-mode plasmas the transport of impurities is found to be mainly dominated by collisions [190]. Modelling of neoclassical transport of heavy impurities in the pedestal has revealed that convection is directed outward in conditions of much stronger ion temperature gradients than density gradients, as expected in a reactor [202]. Integrated modelling studies in which transport codes have been coupled to impurity transport codes have been more recently applied in the prediction of the behaviour of W [203] and also of additional seeded impurities like N [204], however with the inclusion of simplified models for turbulent transport, The experimental realization that light impurity transport could not be completely explained by neoclassical transport only [116, 138, 205–208] motivated the validation of the theoretical predictions of turbulent transport for light impurities [119, 121, 127, 133, 136, 139, 142] adopting both fluid and gyro-kinetic models and comparing predictions with experimental time slices. The non-negligible impact of roto-diffusion was identified [97, 119, 121] but the predictions of light impurities like boron and carbon were often too peaked with respect to the flat or even hollow profile measurements. More recent comparisons [143–145] confirmed this type of disagreement between theoretical predictions and measurements. Comparisons were also performed on separated transport coefficients experimentally obtained by transient transport experiments [117, 122, 139, 146]. The identification of the source of this disagreement has required a few years of research, as instances of quantitative agreement were also obtained, starting from the pioneering work with flux-matching global gyrokinetic turbulence simulations [139]. Most recent results suggest that this problem is now understood and it is the consequence of the neglect in those previous studies which also had NBI heating of the impact of the NBI fast ions [134]. Presently a larger validation effort is undertaken in order to consolidate this result and demonstrate that it can reconciliate the theoretical predictions with the experimental measurements over a broad parameter range. Moreover, while the fast particle effect on the impurity transport is already well reproduced in the quasi–linear gyrokinetic calculations, as compared to the non-linear results, no systematic demonstration is yet available on the capabilities of quasi-linear transport models to quantitatively predict this effect. Additionally, while many aspects of light impurity transport appear now to be consistently understood in the general framework of turbulent transport, He transport still appears to present some challenges. The general feature that the shapes of the He density profiles follow those of the main plasma electron density profiles as originally shown in DIII–D [154] can be considered to be qualitatively largely reproduced by the theoretical predictions, however recent quantitative comparisons on the logarithmic density gradients in the confinement region at AUG show that the He density peaking is regularly underpredicted by the theoretical calculations by a ΔR/Ln approximately equal to 1 [134, 145]. The reasons behind this disagreement are currently unknown and progress would benefit from similar investigations in different devices. An additional aspect which still deserves robust validation is the prediction of the size of the He diffusion coefficient, and, correspondingly, its ratio to the heat conductivities, since this parameter plays a critical role on the impact of the central He source in a burning plasma and the He exhaust requirements [112].
On the side of heavy impurity transport, the importance of including the effect of poloidal asymmetries particularly in the neoclassical calculations has been now robustly recognized [49–52] and demonstrated to also impact the magnitude of the temperature screening term. This effect is required to be properly included in the modelling since in some conditions the reduction of the temperature screening coefficient due to centrifugal low field side localization of the heavy impurities can lead to central impurity accumulation, consistent with experimental observations [50, 51]. Absence of strong rotation allows conditions with reduced neoclassical transport and a stronger role of the turbulent transport, with consequent beneficial effects on the impurity transport behaviour, as shown in both C–Mod [209] and more recently at WEST [210].
The large and successful validation effort performed by combining gyrokinetic and drift-kinetic codes on impurity density profiles from single time slices as well as on transport coefficients from dedicated transient experiments of impurity transport have renewed the interest in the integrated transport modelling, where the recently increased theoretical knowledge can also be taken into account [61, 63, 209, 211, 212]. This has allowed in particular integrated modelling simulations with the JINTRAC plasma simulator code of the W behaviour in JET hybrid scenarios [61] including the dynamical simulation of the accumulation process of W with the concomitant predicted evolution of eight transport channels. This integrated modelling workflow applies the quasi–linear gyrokinetic transport model QuaLiKiz and the drift-kinetic code NEO for the turbulent and neoclassical transport components respectively, both including the impact of the poloidal asymmetries, which are also self-consistently predicted in the simulation. This achievement in the integrated modelling capabilities can be considered the current frontier in this research activity.
A reliable prediction of the development of central accumulation and/or its avoidance remains one of the most important objectives. Here a critical aspect is played by the transport in a very small region very close to the magnetic axis, a region over which a relatively limited amount of investigations has been performed so far and in which the competition between collisional and turbulent transport becomes critical. An additional ingredient which often plays a dominant role in this region is the MHD activity, which is known since long to also impact the impurity behaviour. Although studies dedicated to the impact of MHD instabilities on the impurity behaviour are not considered to be part of this review paper, given their importance, a short mention is certainly in order. Close to the magnetic axis the effect of sawtooth profile relaxations in mitigating or even avoiding impurity accumulation has received large consideration [198, 213–218]. More recently, also more complicated effects have been identified and investigated, in which the presence of long–living, saturated internal kink modes at the q = 1 surface can produce helical deformations of the magnetic equilibrium which can affect the impurity transport, particularly through an interplay with central ECRH, leading to centrally hollow impurity density profiles [219, 220]. These central impurity holes cannot be explained by the usual combination of neoclassical and turbulent transport effects in the presence of an axisymmetric magnetic equilibrium [52, 220] and still await a physical explanation, likely connected with 3D effects of this helical magnetic core on the transport and/or the additional contribution to the radial electric field produced by the presence of fast electrons trapped in the perturbed magnetic configuration [221].
The MHD code XTOR–2F has been extended with the inclusion of impurity transport equations with increasing level of sophistication [222]. Nonlinear simulations of the impurity behaviour during sawtooth crash events have been performed [222–224], demonstrating expulsion of impurity ions in the case of a peaked impurity density profile before crash, and penetration of impurity ions in the case of an initially hollow impurity density profile. The results are also important for the prediction of He ash by sawtooth events and are shown to be largely consistent with the predictions obtained with a Kadomtsev reconnection model. Nevertheless, the condition of initially hollow impurity density profile is more challenging for a quantitative description with the simplified model, in comparison to the nonlinear MHD simulations. Other approaches to compute the impurity transport in the presence of MHD modes are based on the possibilities offered by 3D codes in which the perturbed magnetic equilibrium is obtained by dedicated calculations with a 3D equilibrium code which mimics the presence of the MHD perturbation. An example of this application has been obtained with the guiding–center orbit–following code VENUS–LEVIS on a 3D equilibrium obtained with VMEC [225] in simulations of JET hybrid scenario conditions with a helical core deformation of the equilibrium at the center. Interestingly the result in these conditions show that in the presence of a helical core the inward flux of impurity ions is strongly enhanced by the increased poloidal velocity of the background ions near the magnetic axis, also strongly increasing the impurity peaking with respect to the condition of axisymmetric equilibrium. Thereby, this simulated effect does not appear to be able to explain the impurity hole observed in AUG when in the presence of a helical core also ECRH is applied in the center [220]. These results motivate further studies specific to these conditions, characterized by reactor relevant central electron heating, since a reliable theoretical explanation of these observations is still missing and would be important to acquire validated predictive capability also regarding this type of observations. This is also a domain of research in which direct collaboration between tokamak and stellarator communities could be particularly beneficial. Analogous studies have been intensively carried out in order to explain the so–called 'impurity hole' phenomenon, particularly observed at LHD [226]. These 3D studies have been performed with both gyrokinetic turbulent simulations [227, 228] and neoclassical calculations [229–233]. Both traditional neoclassical theory and gyrokinetic quasi-linear and nonlinear simulations predict an inward convection of the carbon impurity, which cannot explain the hollow carbon density profiles observed in LHD NBI heated plasmas with high ion temperatures. Extensions of the neoclassical model in order to also include the effect of the electrostatic potential variation on a flux surface have been attempted [229–232] in the simulations, also under the consideration that poloidal asymmetries can have strong impact on the impurity neoclassical transport in tokamaks, as described in the present review paper. However, the inclusion of these effects has been found to lead to a further increase of inward convection of carbon. The problem is still under investigation. Very recently, radially global neoclassical transport simulations [233] predict an opposite sign of the ambipolar radial electric field, with a consequent carbon flux directed outward, and the anisotropic potential is found to enhance the removal of impurities from the core. For additional information, progress in neoclassical impurity transport in stellarators has been very recently reviewed [234]. Finally, impact on the impurity behaviour has also been observed to be produced by NTMs [49, 235]. Also in this case, solid theoretical explanations and related theory–based modelling are still pending.
6. Conclusions
This paper has offered a review of the progress in the theoretical description and in the modelling of impurity transport in tokamak plasmas. Recent progress in neoclassical transport, reviewed in section 2, has been obtained by means of the development of more complete models, also with analytical approaches and with the inclusion of the poloidal asymmetry effects in the numerical tools which are applied for a quantitative description of the heavy impurity transport. This has given renewed interest to the study and the validation of theoretical predictions of parallel transport. The aspects of parallel transport have been reviewed in section 2.1, while the collisional radial transport has been presented in section 2.2. From the standpoint of turbulent transport, recent progress has allowed us to obtain an increasingly comprehensive identification of all of the elements that contribute to the turbulent impurity particle fluxes. All of the known elements have been reviewed in section 3, with specific subsections dedicated to the diffusion and to the different convective mechanisms. The results in the validation of the theoretical predictions have been presented following the evolution in time over the last two decades, highlighting the connection with the progress in the theoretical description. Section 4 has reviewed recent studies dedicated to the calculation and the understanding of direct interactions between collisional and turbulent transport processes, showing that important progress has been made in recent years also on this theoretically fundamental aspect. Finally section 5 has provided an at least partly stand–alone review of the progress in the modelling of impurity transport and impurity density profiles and concluded by briefly mentioning the impact of the presence of MHD modes on the impurity behaviour.
The main conclusion that can be drawn from this review is that the present knowledge and understanding of the transport of impurities in a tokamak plasma has reached a level which is comparable to those that are present in the understanding of particle and heat transport. The causes of central impurity accumulation have been clearly identified, the reasons by which certain plasma conditions are more prone to impurity accumulation have been recognized, and, consequently, also the transport mechanisms which allow the accumulation to be avoided in the tokamak operation have been understood from the physics standpoint. The general result that has to be underlined is that while collisional transport can lead to extreme central impurity accumulation, the properties of tokamak plasma turbulence are such that no turbulent transport mechanism can lead to a strong central accumulation of the impurities. Some aspects of the turbulent transport have been only identified or understood very recently, like for instance the impact of fast ions, and thereby these effects still require more complete validation by means of more extensive comparisons with the experimental observations. In particular, the conditions which lead to hollow impurity density profiles and the possibility of reliably and quantitatively predicting this transport property with theoretical models still require a complete assessment. While qualititatively several parametric dependences are well reproduced by theory, quantitative predictions of He density profile shapes appear to still fall outside our current theoretical capabilities, what can be considered surprising, when in similar conditions light impurities like boron or carbon are usually well described by theory. This problem requires further studies from both the theoretical and the experimental standpoints, also aiming at converging on a solid validation of the theoretical predictions for the He diffusivity. First examples of complete integrated modelling workflows which include a comprehensive description of both the collisional and the turbulent transport of the impurities have been performed and can be expected to become routinely performed in the short future, increasing the set of plasma conditions over which integrated modelling approaches are validated. The impact of MHD modes on impurity transport and impurity density profiles has been characterized experimentally and is now motivating interest from the theoretical standpoint, a complex problem which requires 3D effects to be taken into account.
Looking forward, with the aim of reaching a complete predictive capability not only of the impurity transport coefficients and of the shapes of the impurity density profiles in the confined plasma, but also of the actual impurity concentration in the plasma, the connection between the transport in the region with closed field lines, described in the present review, and the transport in the region with open field lines, and thereby also the related prediction of the amount and impact of the impurity sources coming from the walls remain the critical steps. Here multiple effects, such as the 3D geometry of the walls, the specific characteristics of each device in the distribution of the impurity sources, the type of heating systems, the properties of the divertor, in particular in the retention of impurities, and its operational conditions, the additional seeding of impurities, can concomitantly determine the level of concentration of intrinsic and seeded impurities in the confined plasma (e.g. [236] and references therein). Additional effects connected with the type of confinement regime, in particular the presence of edge localized modes (ELMs), and/or with the plasma operation, such as gas puff levels or the application of ELM mitigation or suppression techniques, can also have a strong impact.
Acknowledgments
The Author would like to warmly thank all of the colleagues who, over the last 15 years of his research, have contributed to his understanding and to his research on impurity transport by means of close collaborations or just by very fruitful discussions. In particular, E A Belli, R Bilato, C Bourdelle, F J Casson, Y Camenen, E Fable, X Garbet, P Helander, P Maget, P Manas, A G Peeters, M L Reinke. The Author is also profoundly grateful to the colleagues with whom he had the opportunity of closely collaborating in the performance of dedicated experiments or more generally for the comparisons with the experimental observations on impurity transport, in particular R Dux, A Kappatou, P Mantica, R M McDermott, T Odstrcil, T Pütterich, M Sertoli, M Valisa.
This works has been carried out within the framework of the EUROfusion Consortium and has received funding from the EURATOM research and training programme 2014–2018 and 2019–2020 under Grant Agreement No. 633053. The views and opinions expressed herein do not necessarily reflect those of the European Commission.
Data availability statement
All data that support the findings of this study are included within the article (and any supplementary files).