Abstract
Low-dimensional III–V semiconductor arrays have been widely investigated for photonic, electronic and optoelectronic applications. Bottom-up epitaxy of nanostructures with metastable phase is an effective approach to modify their optical and electronic structures. However, in addition to their optical properties, their thermal properties are also important and can affect device performance. Here, we use wurtzite InP nanosheet arrays as an example and perform detailed optical and Raman scattering studies. It is shown that Al2O3 provides good protection for nanosheets up to 873 K without any observable deterioration in optical properties or phase transition. The A1(TO) and E2h modes show opposite trends in polarization-dependent Raman scattering. The first-order temperature coefficient and thermal expansion coefficient of wurtzite and zincblende InP are obtained from temperature-dependent Raman experiments and are quite close to each other. Furthermore, by applying density functional theory and density functional perturbation theory, the Grüneisen parameter and the thermal expansion of wurtzite InP lattice are calculated, showing good agreement with the experimental Raman data. There is little thermodynamic property difference between wurtzite and zincblende InP phase. The revealed thermal properties of the InP nanosheets provide valuable information for those devices that operate at high temperatures.
Export citation and abstract BibTeX RIS
1. Introduction
Bottom-up epitaxy of low-dimensional III–V semiconductors has been extensively investigated in the last decade due to their potential applications in photonics, electronics and optoelectronics, as well as for further fundamental knowledge of their properties [1–6]. One advantage of bottom-up epitaxy over the top-down process is the ability to form metastable phase [7], which is another approach to modify the optical and electronic properties of semiconductors. For instance, the metastable hexagonal wurtzite (WZ) phase of GaP and Ge has a direct band gap, while their stable bulk phase has an indirect band gap, showing great potential applications in light-emitting devices as well as efficient photodetectors [8, 9]. Thus, fully understanding the optical and material properties of metastable phase is scientifically important. For instance, the thermal expansion coefficient is an important basic thermodynamic parameter of semiconductors, especially for those materials operating at elevated temperatures [10–12]. Raman spectroscopy is a versatile and non-destructive technique to study the phonon vibration properties in semiconductors, providing valuable information, such as crystal symmetry, composition, strain, lattice dynamics and electronic band structure [4]. Temperature-dependent Raman scattering can determine the properties of phonons in a variety of materials [13, 14]. Consequently, Raman scattering has been widely applied to understand the phonon scattering properties in both 2D materials [15] as well as III–V semiconductors [16]. Metastable WZ InP nanowire and nanosheet arrays synthesized via the selective area epitaxy (SAE) method have been reported [17, 18] for solar cells [19], light-emission diodes [20], laser [21], as well as bioscience [22] applications. In contrast to numerous reports on their optical properties, their thermodynamic properties as well as their stability at elevated temperatures have been less investigated. Previously, Raman scattering has been mostly focussed on 1D III–V nanowires. For instance, Mukhopadhyay et al investigated the phonon scattering properties of WZ InP nanowires via both experiments and theoretical calculations [23]. However, no Raman investigation of the WZ InP nanosheets has been reported yet. WZ InP nanosheet has potential applications in lasing. Lasing can increase the temperature of InP nanosheet due to insufficient heat dissipation, while InP is known to be easily decomposed at high temperatures. In addition, the metastable WZ phase may transform into a stable zincblende (ZB) phase at higher temperatures. Therefore, its performance at high temperature is important. The effect of surface passivation is unknown at high temperatures. Moreover, for thermodynamic calculation via the calculation of phase diagrams (CALPHAD) approach to guide the III–V nanowire growth [24, 25], the thermodynamic properties of the metastable phase are necessary to build a comprehensive thermodynamic database. Therefore, it is of great significance to study the thermodynamic properties and surface passivation of metastable WZ InP nanosheets at high temperature.
In this work, we investigate the optical properties of WZ InP nanosheets by polarization-dependent Raman scattering. The A1(TO) and E2h
phonon vibration modes of WZ InP nanosheets show opposite trends under four typical polarization configurations. Moreover, the thermodynamic property is obtained by temperature-dependent Raman scattering in the x(zy) configuration. It is shown that the deposition of an Al2O3 layer around the nanosheets can protect their optical properties up to a temperature of 873 K, without any phase transition. The first-order temperature coefficient (χ) and linear thermal expansion coefficient (αL
) of WZ InP are close to those of ZB InP. These parameters are further confirmed by first-principles calculations using density functional theory (DFT) and density functional perturbation theory (DFPT). These results demonstrate that the thermodynamic properties of metastable WZ InP phase are similar to stable ZB phase.
2. Methods
SAE of WZ InP nanosheet arrays was performed via metal-organic chemical vapor deposition (Aixtron 200/4) with two growth directions of [11] and [1
0]. The pattern preparation process, together with the growth details, has been reported in our previous work [17]. In short, the nanosheet arrays were grown at 750 °C with a V/III ratio of 297. The precursor flow of trimethylindium was 4.2 × 10−6 mol min−1. After growth, the morphology of the nanosheets was examined by both scanning electron microscopy (SEM, FEI Verios 460) and atomic force microscopy (AFM, NanoMan VS). Optical characterizations, including time-resolved photoluminescence (TRPL), were carried out on single InP nanosheets. The InP nanosheet was transferred to a marked Si/SiO2 substrate by scratching the nanosheet array with a paper tip under an optical microscope. Polarization-dependent Raman scattering studies were performed on a single InP nanosheet via a Renishaw system. An Ar ion laser (532 nm) was used for optical excitation through a 100× objective lens, and the Raman signal was collected in a backscattering geometry. The laser power was 1 mW to avoid possible heating of the sample. Room-temperature polarization results were obtained by rotating the nanosheet sample in 10° steps. Temperature-dependent Raman spectra were collected in the temperature range of 123–873 K using a temperature-controlled probe stage (HFS600E-PB4). For this purpose, a 50× objective lens was used. To avoid decomposition, the transferred InP nanosheet on Si/SiO2 substrate was coated with a thin Al2O3 film by magnetron sputtering (SKY Technology Development Co. Ltd). The deposition was performed at radio frequency (RF) power of 50 W and pressure of 0.5 Pa. The Al2O3 target surface was pre-sputtered for 10 min to remove impurities and ensure deposition stability. Then, Al2O3 film with thickness of around 10 nm (measured using a stylus profiling system) was deposited by turning on the sample baffle. All the Raman spectra were obtained under the same experimental conditions. The peak positions and intensities were obtained by fitting a Lorentzian function to the spectrum.
The lattice constant, elastic constant, phonon dispersion, Grüneisen parameter and thermal expansion of the lattice were calculated using DFT and DFPT, which were implemented in the Quantum ESPRESSO package [26, 27]. The local density approximation [28] to the exchange-correlation functional was used for all the calculations. The effect of core electrons is replaced by projector augmented-wave pseudopotentials [29, 30]. The In-5s5p4d and P-3s3p electrons were treated as valence electrons. Plane-wave basis sets were used to expand the electronic wave function and charge density. The cutoff energy for the wave functions and charge density were set to 100 and 600 Ry, respectively. A Γ-centered uniform 6 × 6 × 4 k-point mesh was used to sample the first Brillouin zone. The frequencies of the phonon modes were calculated on a Γ-centered uniform 6 × 6 × 4 q-point mesh.
The Grüneisen parameters of the λth phonon mode at the q-point with respect to the lattice constant a and c were calculated by,
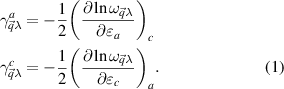
The central difference method around the equilibrium structure was used to compute these derivatives numerically. The strains along the a-axis and the c-axis (
a
and
c
) were varied by a step of 0.004. The thermal expansion of the a-axis and c-axis was calculated by solving the following equation [31]:
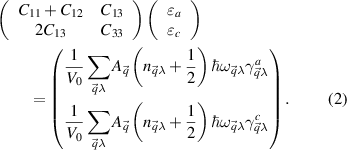
In the above equation, the elastic constants C11, C12, C13 and C33 were calculated from the strain–energy relationship. The factor Aq is the weight of the corresponding q-point. The occupation number of a phonon mode is,

3. Results and discussion
The morphology of as-grown WZ InP nanosheet arrays is shown in figure 1(a). These nanosheets grow uniformly in the (111) direction with a height of 4.5 μm. The crystallography directions and related coordinates during Raman measurements are indicated in figure 1(a), where X, Y and Z are the laboratory coordinates, which are parallel to the [11], [1
0] and (111) directions of the nanosheet, respectively. Figure 1(b) shows the corresponding AFM image of a transferred InP nanosheet. The nanosheet thickness is quite large (278 nm), with the result that the quantum effect is negligible. Therefore, the thermodynamic properties of the nanosheet are mainly determined by its structure. Neglecting several large bulges, most likely due to dust contamination during the transfer process, the nanosheet exhibits a root-mean-square roughness of 0.38 nm, indicating that the as-grown {1
00} facet is almost atomically flat. This further confirms the advantages of SAE in producing nanostructures with a smooth surface [32]. Figure 1(c) compares the Raman spectrum of an InP nanosheet and InP nanowire transferred onto Si/SiO2. Characteristic transverse optical (TO) (304.5 cm−1) and longitudinal optical (LO) (341.6 cm−1) peaks of WZ InP can be observed from both the nanowire and nanosheet [33]. One significant difference is the peak intensity, where the TO and LO peak intensity of the nanosheet is five and two times larger than that of the nanowire, respectively. In addition, extra peaks at 610.8, 648.5 and 685.7 cm−1 are observed and ascribed to the second-order Raman peaks of 2TO, TO + LO and 2LO, respectively [34]. The stronger Raman scattering intensity of the InP nanosheet could be related to larger light–matter interaction volume. The optical properties of the WZ InP nanosheet were further investigated by room-temperature micro-photoluminescence (µ-PL, Qontor Renishaw) and TRPL, as shown in figure 1(d). A strong peak at 870.7 nm (1.42 eV) is observed. WZ InP nanosheet array can be formed along both the [11
] and [1
0] directions with mainly side facets of {1
00} and {11
0}, respectively [17]. Carrier decay of WZ InP nanosheets with {1
00} and {11
0} side facets is nearly the same but slower than their nanowire counterparts. The extracted carrier lifetime for nanosheets and nanowires is 0.51 and 0.25 ns, respectively. From our previous work, the crystal structure of nanowires and nanosheets grown under the same conditions results in the same crystal phase [17]. Therefore, the lifetime difference between nanowire and nanosheet could result from the different surface recombination velocities of different facets [35]. Again, strong PL emission and long carrier lifetime demonstrate the high optical quality of the WZ InP nanosheets.
Figure 1. (a) SEM image of the WZ InP nanosheet arrays showing the relationship between the spatial coordinates and crystal orientations. Inset image is the top view of a nanosheet. (b) AFM mapping of a transferred InP nanosheet. (c) Raman spectra of the WZ InP nanosheet and the nanowire grown under the same conditions. (d) PL spectrum of the WZ InP nanosheet. Inset shows the carrier decay of an InP nanosheet and nanowire.
Download figure:
Standard image High-resolution imageWe further performed polarization-dependent Raman scattering on the same WZ InP nanosheet. The schematic diagram of the measurement set-up is shown in figure 2(a). Based on the cracked edges of the nanosheet, its crystal orientation and the Raman polarization configurations can be determined. Figure 2(b) compares the measured Raman spectra of the nanosheet under four polarization configurations: x(yy), x(zz)
, x(yz)
and x(zy)
. For all configurations, three characteristic peaks at 300.6, 304.5 and 341.6 cm−1 are observed with variation in intensity. These peaks are ascribed to the A1(TO)/E1(TO), E2h
and LO vibration modes of WZ InP, respectively. Since the vibration frequencies of A1(TO) and E1(TO) are the same, they are indistinguishable in the spectrum. Therefore, in the subsequent polarization analysis, the peak at 300.68 cm−1 is assigned to the A1(TO) mode and is observed under all the polarization configurations. In comparison, the E2h
vibration mode is only expected to occur under x(yy)
polarization configurations according to scattering selection rules. However, the E2h
mode peak also occurs with a lower intensity under x(yz)
and x(zy)
polarization configurations. E2h
is a unique phonon vibration mode of WZ phase, and since it is a hexagonal lattice with anisotropy, the polarization configuration perpendicular to the growth direction of the nanosheet will be affected [16]. As a result, Raman signals in the E2h
phonon vibration mode can also be observed under the x(yy)
, x(yz)
and x(zy)
polarization configurations.
Figure 2. (a) Schematic of Raman scattering set-up. Insets are SEM images of the transferred WZ InP nanosheet under four typical polarization configurations: x(yy), x(zz)
, x(yz)
and x(zy)
. (b) Corresponding room- temperature Raman spectra under thepolarization configurations indicated in (a). (c) Polarization- dependent Raman peak intensity of the A1(TO) and E2h
modes.
Download figure:
Standard image High-resolution imageThe Raman spectra in figure 2(b) show an additional weak peak at 341.6 cm−1. Considering our backscattering geometry configuration and the crystallographic axes of a typical WZ nanosheet grown along the (111) direction, only the A1(LO) mode can be experimentally observed, while the E1(LO) mode is forbidden in backscattering configuration [36]. Therefore, we can conclude that the Raman peak appearing at 341.6 cm−1 is from the A1(LO) mode. The presence of this mode could be related to a resonance effect [37].
The extracted Raman scattering intensity of the A1(TO) and E2h
vibration modes at different rotational angles is shown in figure 2(c). The A1(TO) and E2h
peaks have opposite polarization dependence. The A1(TO) vibration mode shows the strongest intensity under x(zz) configuration, while the E2h
vibration mode is strongest under x(yy)
configuration. The Raman scattering results of the WZ InP nanosheet and WZ InP nanowire from the literature [37] are summarized in table 1. The Table shows that the polarization-dependent Raman scattering of WZ InP nanosheets and nanowires has the same trend. Thus, it can be concluded that the Raman scattering property is independent of the nanostructure geometry.
Table 1. Optical phonon modes and polarization configurations in backscattering geometry for WZ InP nanosheets.
Phonon modes | Polarization configurations [16] | Raman exp. ω (cm−1) | Rigid [37] ω (cm−1) | DFPT ω (cm−1) |
---|---|---|---|---|
A1(TO) |
x(yy)![]() ![]() | 300.68 | 305.3 | 301.7 |
302.1 [37] | 304.6 [23] | |||
E1(TO) |
x(yz)![]() ![]() | 300.68 | 306.3 | 305.6 |
302.4 [37] | 309.2 [23] | |||
E2h |
x(yy)![]() | 304.5 | 313.0 | 306.9 |
306.4 [37] | 307.9 [23] | |||
A1(LO) | 341.6 | 346.4 | 346.5 | |
341.9 [37] | 340.2 [23] | |||
E1(LO) | Not observed | 347.3 | 334.4 | |
339.1 [23] |
The vibrational frequency is influenced by both intra- and intermolecular changes, which can be reflected by temperature-dependent Raman scattering [14, 38, 39]. Moreover, it can reveal the possible heat-induced phase transition in metastable WZ phase. Thus, temperature-dependent Raman studies over a large range (from 123–873 K) were performed. A thin Al2O3 passivation layer was deposited by RF sputtering to avoid possible decomposition of the InP nanostructure during heating. Moreover, the polarization configuration of x(zy) was used to better reveal the change in the A1(TO) and E2h
vibration modes with temperature. Figure 3(a) shows the temperature-dependent Raman spectra of a WZ InP nanosheet. Both the A1(TO) and E2h
modes follow a systematic red-shift and the total peak intensity drops with an increase in temperature. Even though the scattering intensity of the A1(TO) and E2h
peaks is strong up to 873 K, the LO mode becomes indistinguishable when the temperature exceeds 513 K (see figure S1 (available online at stacks.iop.org/JPD/54/505112/mmedia)). The variation in peak positions with temperature is predominantly due to anharmonicity [40]. The increase in temperature changes the atomic positions and the interatomic potential energy due to phonon–phonon interaction and thermal expansion [41–45]. Raman scattering signal of undoped ZB InP wafer was collected and is shown in figure 3(b) as a reference. Only the TO mode of the ZB InP substrate is observed during the temperature-dependent measurements. For both WZ and ZB InP, all modes decrease monotonously in wavenumber with increasing temperature without any anomaly, which suggests the absence of any structural-phase transition within the investigated temperature range. The extracted peak position shifts with temperature for both samples are compared in figure 3(c). The first-order temperature coefficient (χ) can be obtained via a linear equation [46]:

Figure 3. Temperature-dependent Raman spectra of (a) WZ InP nanosheet and (b) ZB InP substrate from 123–873 K. Extracted (c) Raman peak position and (d) full width at half-maximum (FWHM) for different vibration modes for the two samples.
Download figure:
Standard image High-resolution imagewhere ω0 is the frequency of the vibration mode at 0 K. The extracted ω0 for the A1(TO) and E2h modes is 304.97 and 309.18 cm−1, respectively, with χ values for the A1(TO) and E2h modes being −0.01536 ± 4.6 × 10−4 and −0.01673 ± 4.6 × 10−4 cm−1 K−1, respectively. The temperature coefficient of the E2h mode is slightly larger than that of the A1(TO) mode, indicating a slight difference in the sensitivity of the modes to temperature [47]. When the temperature is above 700 K, the peak shift is not obvious, most likely because the scattering process of phonons reduces the sensitivity of phonon frequencies to temperature [48]. Moreover, the extracted ω0 and χ values of the ZB InP substrate are 307.21 cm−1 and −0.01568 ± 4.4 × 10−4 cm−1 K−1, respectively, which are in good agreement with the literature [49]. After cooling down, Raman and PL spectra of the nanosheet were collected again, as shown in figure S2. The Raman scattering and PL emission intensities are nearly the same as those before heating, suggesting that heat treatment does not cause any structural change or deteriorate its optical performance.
The FWHM of the Raman peaks as a function of temperature is plotted in figure 3(d). The FWHM of both the E2h and A1(TO) modes increases slightly with temperature for the WZ InP nanosheet, in comparison to the much larger increase in the TO mode of the ZB InP substrate. The narrow linewidth as well as its slow increase with temperature indicates a high crystal quality of the nanosheet and the protective effect of the Al2O3 layer. In comparison, the peak width of the ZB InP substrate increases significantly during heating up, showing clear degradation in the quality of the InP crystal. Anharmonicity in the crystal allows the decay of Brillouin zone-center optical phonons into one acoustic and one optical phonon. Increasing temperature causes a rise in phonon–phonon interactions, resulting in peak broadening. The selection the of A1(TO) and E2h phonons in the WZ InP phonon density of states contributes to the change in FWHM. For this peak broadening mechanism, the temperature-dependent FWHM can be expressed as [50],

where Г(0) is the FWHM of a vibrational mode at absolute zero, ℏ and k are Planck's constant and Boltzmann constant, respectively, and ω0 is the value fitted using equation (1). The extracted FWHM of different vibration modes can be well fitted via equation (2), which indicates the validity of the peak broadening mechanism proposed above. Thus, it is suggested that the temperature-induced peak broadening in WZ and ZB InP is caused by phonon–phonon interactions [38, 51].
The red-shift of the optical phonon with increasing temperature is generally associated with a combinatorial effect of volume (thermal expansion) and temperature contributions, resulting from anharmonicity in the crystal [52]. The phonon frequency ω can be expressed as a function of volume and temperature as follows [53]:
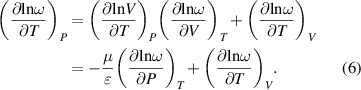
Here, μ= (∂ ln V/∂T)P
and = −(∂ ln V/∂P)T
are the volumetric thermal coefficient and volumetric isothermal compressibility, representing the volumetric contribution at constant temperature, and the temperature contribution at constant volume, respectively. Using equation (3) and the thermodynamic parameters (µ,
), we can obtain the anharmonic (pure-temperature) contribution. The most important anharmonic quantity is the thermal expansion coefficient (αV
), which is given by [54],

In addition to its importance, the study of anharmonic properties is closely related to the study of the equation of state. Moreover, Grüneisen parameter (γ), which is another important parameter, is widely used in describing the thermodynamic properties of crystals. For the metastable WZ phase, Grüneisen parameter can hardly be measured due to difficulties in sample preparation. Thus, this parameter is estimated to be the same as the stable phase during the previous publication, as illustrated in GaN [55]. Indeed, this parameter can be theoretically calculated within the DFT approach, as applied in this work. We first calculated the frequencies of the A1(TO) (301.7 cm−1) and E2h (306.9 cm−1) modes. The calculated values agree with the extracted values from experiments, confirming the validity of our calculations and experiments. The calculated dispersion of phonons along a high-symmetry path in the first Brillouin zone is shown in figure 4. There is a large energy gap between the low-lying six acoustic bands and the other six optical bands.
Figure 4. Theoretical phonon dispersion and Grüneisen parameter of each phonon mode of WZ InP. Pseudo-color represents the calculated Grüneisen parameter for each phonon mode with respect to (a) the lattice constant a and (b) the lattice constant c.
Download figure:
Standard image High-resolution imageThe Grüneisen parameter describes the modulation of vibration frequencies by changing the crystal volume. The WZ phase has a lower symmetry than the cubic ZB phase. Thus, the vibrational frequency as a function of crystal volume cannot be obtained directly. Instead, we calculate the vibrational frequencies as a function of lattice constants a and c, as shown in figure 4. The obtained A1(TO) and E2h Grüneisen parameters of WZ InP are 1.39 and 1.36. In the whole high-frequency region, the Grüneisen parameter of WZ InP is 1.375. Using the same method, the calculated Grüneisen parameter for the ZB phase is 1.38, which is slightly smaller than the measured value (1.44) in the literature [56] and similar to the calculated value for the WZ phase. The Grüneisen parameters of high-frequency (>100 cm−1) phonon modes are positive. These high-frequency modes are occupied at high temperatures. They cause positive thermal expansion at high temperatures. For a large part of the phonon modes with their frequencies lower than 100 cm−1, the Grüneisen parameters are negative, especially for the two lowest TA modes. The thermal excitation of these modes with negative Grüneisen parameters is expected to cause negative thermal expansion below 20 K.
Based on the theoretically calculated γ, the linear coefficient of thermal expansion (αL ) is approximately one-third of αV , and αL is correlated to the γ by the following equation [55]:

The obtained αL of A1(TO), E2h modes of WZ InP nanosheet and TO mode of ZB InP substrate are quite close to each other, namely 4.03 × 10−6, 4.42 × 10−6 and 4.11 × 10−6 K−1, respectively. The extracted linear thermal expansion coefficient for InP substrate is close to that found in the literature (4.35 × 10−6 K−1) [57]. In addition,the thermal expansion can be theoretically calculated using the weighted average of Grüneisen parameters. We calculated the lattice constants (a and c) at a series of temperatures from 200–800 K. The calculated volumetric thermal expansion coefficient is 15 × 10−6 K−1. The equivalent linear thermal expansion coefficient is 5.01 × 10−6 K−1, which is close to the experimental data above. We also calculated the frequencies of the A1(TO) and E2h modes at different values of a and c. Using the calculated lattice constants as a function of temperature, we can derive the frequencies of the A1(TO) and E2h modes at different temperatures. The calculated temperature coefficients of these two modes are in fairly good agreement with experimental data (table 2).
Table 2. Thermodynamic coefficients of different phonon vibration modes of InP nanomaterials with different crystal phases. The numbers in parentheses are theoretical results. The theoretical linear thermal expansion coefficient is deduced from the calculated volumetric thermal expansion coefficient.
Crystal | Phonon mode | χ (cm−1 K−1) | γ | αL (×10−6 K−1) | β (meV GPa−1) |
---|---|---|---|---|---|
WZ InP nanosheet | A1(TO) | −0.01536 ± 4.6 × 10−4 | 1.39 a | 4.03 | 88.2 ± 0.5 [60] |
−0.01211 a | 5.01 a | ||||
E2h | −0.01673 ± 4.6 × 10−4 | 1.36 a | 4.42 | ||
−0.01080 a | 5.01 a | ||||
ZB InP substrate | TO | −0.01568 ± 4.4 × 10−4 | 1.38 a | 4.11 | 76 [60] |
−0.0168 [61] | 1.44 [57] | 4.35 [57] |
aRepresents data obtained by theoretic calculations in this work.
In addition, Chaix-Pluchery et al [54] obtained the first-order linear pressure coefficient (β) of WZ InP nanowires (88.2 meV GPa−1), which is slightly larger than the ZB counterparts (76 meV GPa−1) [26, 58]. The obtained thermodynamic coefficients of WZ and ZB InP via both experiments and theoretical calculations are summarized in table 2. The obtained thermodynamic parameters for ZB and WZ InP phase, such as temperature coefficient, Grüneisen parameter, thermal expansion, linear pressure coefficient, as well as heat capacity [59] are quite close to each other, which indicates that the crystal-phase change in InP does not cause a huge change in thermodynamic properties compared to the impact on electronic and optical properties. These results suggest that the thermodynamic properties of the metastable phase in III–V semiconductor may be estimated using the value of its stable counterpart. Moreover, these properties are necessary to establish a thermodynamic database to guide the III–V nanostructure growth via the semi-empirical CALPHAD method [24, 25].
4. Conclusion
We performed detailed Raman and PL studies of metastable WZ InP nanosheets. The side facets of SAE nanosheets are almost atomically flat and Al2O3 can protect them from decomposition up to 873 K. Moreover, no phase transition is observed during the temperature range from 123–873 K. The A1(TO) and E2h modes of the nanosheets show opposite polarization-dependent trends and these trends are independent of the nanostructure morphology. Furthermore, thermodynamic parameters, such as first-order temperature coefficient and thermal expansion coefficient were extracted from temperature-dependent Raman experiment. The determined χ and αL for WZ and ZB InP are quite close to each other, suggesting that the thermodynamic properties of metastable WZ InP nanosheets are quite similar to their bulk stable ZB phase. Finally, DFT and DFPT have been used to calculate the phonon dispersion of WZ InP. The calculated phonon frequencies, first-order temperature coefficients and linear thermal expansion coefficients are close to the experimental data, confirming the practical operation and feasibility of our experimental scheme. Our results show that there is very little difference in the thermodynamic properties of WZ and ZB InP crystals. These basic parameters of WZ InP nanosheets provide valuable information for their application in devices, especially for those working at elevated temperatures. Understanding the properties at higher temperature as well as surface passivation of InP provides valuable information for both fundamental scientific research as well as devices working at high temperatures.
Acknowledgments
The Australian Research Council (ARC), National Natural Science Foundation of China (Grant Nos. 61974166 and 12004439) and the Postgraduate Innovative Project of Central South University (Grant No. 1053320192152) are acknowledged for their financial support. The Australian National Fabrication Facility, ACT Node and the Australian Microscopy and Microanalysis Research Facility are acknowledged for access to facilities used in this work.
Data availability statement
The data that support the findings of this study are available from the corresponding author upon reasonable request.
Conflict of interest
The authors declare that they have no known competing financial interests or personal relationships that could have appeared to influence the work reported in this paper.