Abstract
Recent progress in superconducting metal-induced surface reconstructions on silicon is reviewed, mainly focusing on the results of the author's group. After a brief introduction of an ultrahigh-vacuum (UHV)–low-temperature (LT)-compatible electron transport measurement system, direct observation of the zero resistance state for the Si(111)–()-In surface is described, which demonstrates the existence of a superconducting transition in this class of two-dimensional (2D) materials. The measurement and analysis of the temperature dependence of the critical current density indicate that a surface atomic step works as a Josephson junction. This identification is further confirmed by LT-scanning tunneling microscopy (STM) observation of Josephson vortices trapped at atomic steps on the Si(111)–(
)-In surface. These experiments reveal unique features of metal-induced surface reconstructions on silicon that may be utilized to explore novel superconductivity.
Export citation and abstract BibTeX RIS
1. Introduction
The term "superconductivity" might remind many of us of bulk materials. This is presumably because superconductivity is a macroscopic phenomenon that requires a huge number of electrons and its representative applications such as a superconducting magnet are based on bulk materials. Studies on thin films and micro- and nanoscale superconductors, however, are also important considering the recent progress in superconducting digital integrated circuits and highly sensitive magnetic sensors.1,2) A particularly fascinating possibility is the integration of superconducting building blocks into an organized complex system with emergent functionalities based on the concept of nanoarchitectonics.3) What happens if the size of a superconductor is reduced toward the atomic-scale limit? The possibility of a two-dimensional (2D) superconductor with a truly atomic-scale thickness is especially intriguing in terms of the development of novel 2D materials such as graphene.4) Theoretically, it has long been established that an ideal 2D system can become superconducting through the Kosterliz–Thouless–Berezinskii (KTB) transition.5,6) Nevertheless, the existence of 2D superconductivity in the atomic-scale limit has not been widely recognized until very recently. This is partly ascribed to the experimental observations of the superconducting-insulating transitions in this regime, which are driven by inevitable structural and chemical disorders in 2D materials occurring at surfaces and interfaces.7,8)
In this review paper, we introduce the superconductivity of metal atomic layers epitaxially grown on silicon surfaces, which are technically called metal-induced silicon surface reconstructions.9) They feature ultimately small thicknesses, unique atomic and electronic structures distinct from bulk counterparts, and simple chemical compositions. Superconductivity in this class of 2D materials was first discovered by scanning tunneling microscopy (STM) measurements based on the spectroscopic evidence of the energy gap at low temperatures.10,11) This finding was followed by direct observations of the superconducting transition through electron transport measurement, clarifying the emergence of the zero resistance state.12–14) These experimental efforts firmly established the existence of 2D atomic-layer superconductors. We stress that this new direction of research has been made possible owing to the development of combined ultrahigh-vacuum (UHV) and low-temperature (LT) technology; because the handling of metal atomic layers on silicon demands the UHV technology while the emergence of superconductivity requires an LT environment, this combination and compatibility are essential. It is also noteworthy to mention that, almost concurrently, a variety of new 2D superconductors have been discovered recently and are now under rapid progress: electric-field-induced 2D gas at interfaces, atomic sheets of transition-metal dichalcogenide, FeSe monolayers on SrTiO3, etc.15–17) The surface metal atomic layers on silicon treated here are considered to be one of these 2D superconductor families. Many advanced techniques for sample fabrication and instrumentation developed in the field of surface science and nanotechnology are applicable to this system, including STM, angle-resolved photoemission spectroscopy measurements (ARPES), and molecular self-assembly. This opens new possibilities for the superconductivity research, which has conventionally concentrated on bulk materials. In the following, we will describe recent developments in surface atomic-layer superconductors on silicon, mostly focusing on our results.
2. Direct observation of superconducting phase transition by electron transport measurement
Figure 1(a) shows the UHV–LT electron transport measurement system that has been developed in our laboratory.18) This apparatus allows standard preparations and characterizations of samples used in the field of surface science, together with in situ electrode patterning by a shadow mask technique. Figure 1(b) shows the schematic drawing of the electron transport measurement unit accommodated within the cryostat, where electrical contact to the sample is secured by pressing the spring probes onto it. After being loaded into the unit, the sample can be cooled to the lowest temperature of 1.5 K by flowing and pumping liquid helium. The sample stage is surrounded by double radiation shields that are cooled by return of the helium flow and by liquid nitrogen.19)
Download figure:
Standard image High-resolution imageFig. 1. (a) Overview photograph of the UHV–LT electron transport measurement system. (b) Schematic drawing of the electron transport measurement unit. Adapted from Refs. 13 and 19.
Download figure:
Standard image High-resolution imageThe Si(111)–()-In surface [referred to as (
)-In] was chosen for the study, and consists of indium atomic layers on a Si(111) surface with a commensurate relation of the
periodicity.20–22) According to a recent atomic structural model proposed by ab-inito calculations, the surface indium adatoms are made of a double atomic layer.23,24) The sample was prepared by high-temperature flashing of the silicon substrate up to 1250 °C in the UHV environment, followed by indium deposition and post-annealing at around 300–400 °C. The sample surface was confirmed to have a highly crystalline (
)-In reconstruction with large domains through STM observations and low-energy electron diffraction (LEED) measurements [see Figs. 2(a) and 2(b)]. It was further patterned by etching the unnecessary surface areas using an Ar+ ion beam and a shadow mask, which defined the electrode and the current path for electron transport measurement. Finally, the sample was inserted into the cryostat within the instrument without breaking the UHV environment and the transport properties were measured by the four-terminal method.
Fig. 2. (a) STM image of the ()-In surface (bias voltage: Vs = −0.0015 V). (b) LEED pattern taken on the (
)-In surface (beam current: E = 86 eV). (c) Resistance of the (
)-In surface measured as a function of temperature. The resistance was defined as the ratio of the measured voltage to the bias current. The inset shows the data over a larger temperature range. Adapted from Ref. 12.
Download figure:
Standard image High-resolution imageFigure 2(c) shows a representative temperature dependence of the sample resistance, which was measured by the van der Pauw-type four-terminal method with configurations I and II.12) The resistance R defined as the ratio of the measured voltage to the bias current (= 10 µA) was found to sharply decrease to nearly zero at Tc = 2.8 K. The fact that the zero resistance was detected simultaneously for the two orthogonal directions demonstrated the manifestation of a superconducting transition at Tc. The gradually accelerating decrease in resistance observed for T > Tc is a precursor of the superconducting transition. This is unique to low-dimensional systems and is caused by thermal fluctuations of Cooper pairs, which are temporarily created and annihilated.25) Our quantitative analysis showed that this effect could be expressed by including both the Aslamazov–Larkin term and the Maki–Thompson term in the microscopic theory of superconductivity.13)
Correspondingly, the current–voltage (I–V) characteristics signified the emergence of superconductivity at Tc [see Fig. 3(a)]. While only a linear I–V curve was observed for T > Tc, the zero resistance (superconducting) regime appeared at around I = 0 at Tc and extended as the temperature was decreased further. The transition from the superconducting to the normal state was extremely sharp. The critical current Ic defined as this threshold amounted to 520 µA at 1.8 K, which corresponded to a very high 2D critical current density of J2D,c = 2.0 A/m. Interestingly, the observed I–V characteristics were asymmetric with respect to the zero bias point; i.e., the threshold current for the transition from the normal to the superconducting state, Ir, was smaller than the critical current Ic. This phenomenon was independent of the current sweeping direction [indicated by the arrow in Fig. 3(a)], leading to a hysteresis in the I–V characteristics [inset of Fig. 3(a)]. Its origin can be attributed to a delay in the transition to the superconducting state caused by Joule heating in the normal state and a consequent temperature rise of the sample.
Fig. 3. (a) Temperature dependence of I–V characteristics (1.77 < T < 3.11 K). The inset shows a hysteretic behavior of the I–V characteristics taken at 1.8 K. (b) Temperature dependences of the critical current Ic and the 2D critical current density J2D,c). Fitting results based on two theoretical models are also indicated by the blue solid curve (Josephson junction model) and by the red dotted curve (Cooper pair breaking model). (c) Schematic illustration of a metal-induced surface reconstruction including an atomic step. The arrows indicate a supercurrent flow corresponding to a Josephson vortex. Adapted from Ref. 12.
Download figure:
Standard image High-resolution imageThe temperature dependence of the critical current [see Fig. 3(b)] gives one an insight into the place where the superconducting current is regulated. Two possibilities exist: i) within flat terrace areas on the sample surface and ii) at atomic steps that separate the terraces [see Fig. 3(c)]. In the former case, the critical current is determined by the point where the Cooper pairs are broken by an excessive supercurrent, leading to the following form of the temperature dependence of J2D,c:26)

In the latter case, the atomic step works as a Josephson junction, the critical current of which determines that of the sample. Assuming that the junction is of the tunneling type, J2D,c is given by the Ambegaokar–Baratoff equation:27)

where Δ(T) is the superconducting energy gap and ρstep is the resistance per length of the atomic step. It was found that the fitting analysis based on the latter model could reproduce the experimental result better than the former, suggesting the validity of the Josephson junction picture [see Fig. 3(b)]. Here, we chose Δ(T) = 0.57 meV from the literature11) and assumed the temperature dependence of Δ(T) given by the Bardeen–Cooper–Schrieffer (BCS) theory. The value of ρstep = 2.4 × 10−4 Ω m obtained from the fitting was approximately equal to ρstep = 3.3 × 10−4 Ω m, which was deduced under the assumption that the normal sheet resistance of the sample was attributed to the electron scattering at atomic steps.
The significance of the above experiment lies in the fact that it has demonstrated not only the emergence of superconductivity itself but also the existence of macroscopic supercurrents (i.e., macroscopic superconducting coherence) in the metal-induced surface reconstruction. This is surprising since numerous atomic steps present on the surface could sever the current path but nevertheless, supercurrents can run for a long distance of the order of millimeters. Our experiment also allowed us to obtain important information on the critical supercurrent in this system for the first time. We note that, for the samples exhibiting clear superconducting transitions, their sheet resistances ρ2D are typically less than 400 Ω, which is much smaller than the quantum resistance for Cooper pairs, h/4e2 (= 6.45 × 103 Ω). It has been established that a transition from the superconducting to the insulating states takes place for ρ2D > h/4e2 as a consequence of the destruction of macroscopic coherence.7,8) Hence, our result is compatible with the criteria for retaining superconductivity. The presence of such a low sheet resistance despite the atomic-scale thickness of the sample is attributed to a high crystallinity of the surface reconstruction, implying a significant difference compared with the case of conventional thin metal films. Subsequent electron transport measurements on the Si(111)–SIC–Pb surface, monolayers of Pb and In on a GaAs(110) surface, and Si(111)–()-TlPb also confirmed the existence of supercurrents in this class of 2D materials.14,28,29)
3. Observation of Josephson vortices with STM
A sufficiently thin 2D superconductor behaves as a type-II superconductor regardless of its bulk properties. This means that the out-of-plane magnetic field penetrates into the sample plane easily and induces circulating supercurrents with a quantized vorticity in the layer, called superconducting vortices.25) At the center of a vortex, superconductivity is destroyed by excessive supercurrents and is replaced by a normal-like state extending for a distance on the order of the coherence length ξ. While the density of states at the Fermi level ρ(EF) of a superconductor is strongly suppressed by the energy gap opening below Tc, ρ(EF) recovers toward the normal-state value at a vortex core owing to the destruction of the energy gap. Since an STM can measure the spatial distribution of ρ(EF) by mapping the differential conductance (dI/dV) signal at V = 0, it can directly image superconducting vortices in real-space.30) Such an experiment was performed for metal-induced surface reconstructions on silicon using Si(111)–SIC–Pb and Si(111)–()-Pb, but the influence of surface defects on the vortex formation was not clear.11,31) We successfully revealed the effect of an atomic step on vortices using the Si(111)–(
)-In surface, which led to the observation of the so-called Josephson vortex with an STM for the first time.32)
The experiment was performed using a UHV–LT STM accommodated in a liquid helium 3 cryostat with a superconducting magnet, which was constructed at the Institute for Solid State Physics, the University of Tokyo.33) Figure 4(a) shows a topographic image of the sample obtained at T = 0.5 K, where flat terraces are separated by atomic steps α − δ. A dI/dV image of the same area is displayed in Fig. 4(b), which was taken at a sample bias voltage Vs = 0 and under a perpendicular magnetic field of Bext = 0.08 T. The figure features round bright spots on the terraces that form triangular lattices. Since they correspond to the regions with a high density of states at the Fermi level, ρ(EF), they are assigned to the vortex cores where superconductivity is suppressed. Reduction of the magnetic field to Bext = 0.04 T led to a decrease in the number of vortices as expected, and to an extension of the dark-colored superconducting regions with low ρ(EF) [see Fig. 4(c)]. The above interpretation was corroborated by dI/dV spectra taken at the vortex center and sufficiently far away from it [Fig. 4(e)]. At the latter site, characteristic dI/dV spectra originating from the superconducting energy gap were clearly observed, for which the energy gap Δ = 0.39 meV was deduced through fitting to a theoretical form (the red solid and black dashed lines). In contrast, the superconducting energy gap was strongly suppressed at the former site (blue solid line).
Fig. 4. (a) Topographic STM image of a ()-In surface. Flat terraces are separated by the atomic steps α, β, γ, δ. Sample bias voltage: Vs = 900 mV, tunneling current It = 10 pA. (b)–(d) ZBC image taken on the same surface region as in (a). Magnetic fields Bext were applied in the surface normal direction [(b) Bext = 0.08 T, (c) Bext = 0.04 T, and (e) Bext = 0.00 T]. The locations of the atomic steps are indicated by white solid lines. (e) dI/dV spectra taken outside of (red solid line) and at the center of (blue solid line) a vortex. The dashed line is the fitted result using the Dynes formula. (f) Profiles of ZBC measured along the solid lines indicated in (c) and (d). Adapted from Ref. 32.
Download figure:
Standard image High-resolution imageWhen the magnetic field was set at zero, anomalous behaviors were observed for vortices trapped along atomic steps, as shown in Fig. 4(d). The vortices indicated as A', B', and C' are elongated along the steps compared with the vortices with round shapes found within the terraces [for example, see vortex D in Fig. 4(c)]. Furthermore, the cores of vortices A'–C' appear less bright than those of vortices on terraces, indicating that superconductivity was partially recovered at the cores. This is quantitatively displayed in Fig. 4(f), where the profiles of the dI/dV signals acquired at Vs = 0 V (zero-bias conductance, ZBC) are plotted for vortices A'–C' and D. Note that these anomalies are enhanced in the order of A' → B' → C'. Vortex C' is elongated along the step over such a long distance that it suffers spatial inhomogeneities. Nevertheless, the assignment of this feature to a vortex can be assured by comparing it to region C in Fig. 4(c) [see also Fig. 4(f) for the profiles]; the observation that ZBC was higher at C' than at C despite the reduction of Bext to zero means that a vortex was driven into this region.
The anomalies described above are the consequences of the fact that an atomic step works as a Josephson junction. As schematically shown in Fig. 3(c), the circulation of supercurrents in a vortex trapped at a step can be completed only if they run over the step. If an atomic step is a Josephson junction, the critical current density at the step, Jc, is smaller than that on the terrace, J0. This gives rise to a superconducting phase difference Δϕ at the step that depends on the supercurrent density J:

In this case, the vortex core, the usual size of which is the coherence length ξ, is expanded by a factor of (Jc/J0)−1 in the direction parallel to the step.34) Simultaneously, the superconducting energy gap Δ is recovered from zero to Δ0[1 − (Jc/J0)2] at the vortex core. Particularly for Jc/J0 ≪ 1, the vortex becomes substantially elongated along the step, and Δ and ρ(EF) are nearly equal to those of the surrounding superconducting regions.
This situation was reproduced in a more quantitative manner by numerically solving the Bogoliubov–de Gennes equation for a modeled Josephson junction. The model adopted here consisted of two 2D square lattices with the intersite transfer amplitude t, which were coupled by a smaller transfer amplitude ts. The boundary of the two lattices corresponds to a Josephson junction. Figure 5 displays the result of the calculations for a vortex trapped at the junction for different ts/t values. The amplitude and phase of the superconducting order parameter Ψ(r) [proportional to the superconducting energy gap Δ(r) in this case] are respectively plotted in the upper and lower panels of Figs. 5(a), 5(c), and 5(e), while ρ(EF) is displayed in a color scale in Figs. 5(b), 5(d), and 5(f). It was found that, as ts/t was reduced from 0.8 to 0.1, |Ψ(r)| recovered and, consequently, ρ(EF) decreased at the center of the vortex. Simultaneously, the shape of the vortex became elongated along the direction of the junction line. These variations correspond exactly to the experimentally observed vortices A', B', and C' in Fig. 4(d), which clearly shows that an atomic step works as a Josephson junction. In particular, a vortex corresponding to Jc/J0 ≪ 1 (ts/t ≪ 1) can be regarded as a Josephson vortex. While Josephson vortices play important roles in the physics of high-Tc cuprates,35) their electronic states were observed with an STM for the first time in our experiment.
Fig. 5. Superconducting order parameter Ψ(r) (a, c, e) and local density of states at the Fermi level ρ(EF) (b, d, f). The upper and lower panels of (a), (c), and (e) show the amplitude and phase of Ψ(r), respectively. The dashed line indicates the location of the modeled Josephson junction. (a, b) ts/t = 0.8, (c, d) ts/t = 0.4, (e, f) ts/t = 0.1. Adapted from Ref. 32.
Download figure:
Standard image High-resolution image4. Summary and outlook
In the system of a metal-induced surface reconstruction on silicon, Cooper pairs responsible for superconductivity are confined within the atomic layers on the surface. This naturally leads to a high sensitivity of superconductivity to surface defects. Here, an atomic step was found to work as a Josephson junction because of this surface sensitivity, which led to the regulation of macroscopic supercurrents and the emergence of Josephson vortices at atomic steps. In this regard, point defects in the surface atomic layer are also interesting to investigate. Indeed, our recent STM experiment has revealed that a high density of defects in the Si(111)–()-In surface led to a suppression of the density of states around the Fermi level as a result of the Coulomb interaction.36) This was accompanied by the disappearance of the superconducting energy gap, leading to the real-space observation of a superconductor-metal transition in the surface atomic layer system. Currently, we are investigating the effects of self-assembled magnetic molecules on the superconductivity of the Si(111)–(
)-In surface. Such a high sensitivity of macroscopic superconducting properties to surface defects and adsorbates will be a central topic in forthcoming studies.
Another important feature of the present system is the space-inversion symmetry breaking at the surface. Because of this intrinsic property, if the surface metal layer is composed of a heavy element such as Pb, the resulting strong spin–orbit interaction leads to the manifestation of the Rashba effect.37,38) The influence of the Rashba effect on superconductivity has been studied so far in the field of rare-earth-based heavy-fermion compounds. Many intriguing phenomena have been theoretically predicted, including spin singlet–triplet mixing of Cooper pairs, a spatially modulated superconducting order parameter, and an extremely high critical magnetic field in the in-plane direction.39) Recently, the Rashba-split Fermi surface of the Si(111)–()-TlPb surface, which exhibits superconductivity at 2.25 K, was directly observed by ARPES.29) The signatures of the Rashba effects were already detected in the superconducting properties of some of the surface atomic layer systems.28,31) Furthermore, the combination of a magnetic layer and the Rashba effect with 2D superconductivity may lead to the realization of topological superconductivity and Majorana fermions, which are some of the hottest topics in condensed matter physics.40,41) The metal-induced surface reconstructions on silicon introduced in the present review will provide an ideal platform for the study of new exotic superconductors in the future.
Acknowledgments
This work was financially supported by JSPS KAKENHI Grant Number 25247053 and by the World Premier International Research Center (WPI) Initiative on Materials Nanoarchitectonics, MEXT, Japan. The author thanks S. Yoshizawa, P. Mishra, T. Nakayama, M. Aono, T. Kawakami, Y. Nagai, X. Hu, H. Kim, and Y. Hasegawa for their contributions to the studies presented here. He also thanks A. Tanaka and M. Kohno for fruitful discussions on 2D superconductivity.
Biographies
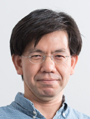
Takashi Uchihashi is the group leader of Surface Quantum Phase Materials Group, International Center for Materials Nanoarchitectonics (MANA), National Institute for Materials Science (NIMS), Japan. He received a doctor degree in 1995 from the University of Tokyo. He started working as a researcher at National Research Institute for Metals, a former institute of NIMS, in 1995. He was a visiting researcher at Institute of Experimental and Applied Physics, Christian-Albrechts-Universität zu Kiel, Germany in 2006, and has been a visiting professor at Yokohama City University since 2015. His main research fields are surface science, nanotechnology, and low temperature physics.