Abstract
Planets and planetesimals acquire their volatiles through ice and gas accretion in protoplanetary disks. In these disks, the division of volatile molecules between the condensed and gaseous phases determines the quantity of volatiles accreted by planets in different regions of the disk. This division can be strongly affected by entrapment of volatiles into less volatile ice matrices, resulting in different radial profiles of common volatiles and elemental ratios than would otherwise be expected. In this study we use laboratory experiments to explore the ability of abundant interstellar and cometary ice matrices, i.e., H2O and CO2, to trap the hypervolatiles 13CO, 12CH4, 15N2, and Ar. We measure entrapment efficiencies through temperature programmed desorption for two ice thicknesses (10 and 50 monolayers) and two mixing ratios (3:1 and 10:1) for each matrix:volatile combination. We find that ice entrapment efficiencies increase with ice thickness and ice mixing ratio to a maximum of ∼65% for all hypervolatiles. Entrapment efficiencies are comparable for all hypervolatiles, and for the two ice matrices. We further find that the entrapment efficiency is relatively insensitive to the ice deposition temperature between 10 and 30 K with the possible exception of CH4 in CO2 ice. Together these results suggest that hypervolatile entrapment at low temperatures (<30 K) is a remarkably robust and species-independent process.
Export citation and abstract BibTeX RIS

Original content from this work may be used under the terms of the Creative Commons Attribution 4.0 licence. Any further distribution of this work must maintain attribution to the author(s) and the title of the work, journal citation and DOI.
1. Introduction
Planets and planetesimals form from dust, ice, and gas in protoplanetary disks around young stars (Williams & Cieza 2011; Andrews et al. 2018; Raymond & Morbidelli 2022), and the resulting planet compositions depend on the abundance and distributions of volatiles compounds within the disk (Öberg et al. 2011b; Henning & Semenov 2013; Madhusudhan 2019). This distribution fundamentally depends on how volatiles are divided between the gaseous and solid phases. This is relatively straightforward to predict if the disk temperature profile is known, transport processes and chemistry are negligible, and volatiles freeze out as pure condensates, for which binding energies and hence desorption rates are known (Öberg & Bergin 2021; Minissale et al. 2022). In reality, however, we do not expect icy grains in the disk to be pure. In the interstellar medium, ices, mostly composed of H2O and CO2, are often mixed with more volatile compounds (Allamandola et al. 1999; Boogert et al. 2015). These mixtures form as a result of codeposition of atoms and molecules during ice formation in interstellar clouds, and subsequent ice chemistry. These mixed ices can survive disk formation (Visser et al. 2009; Drozdovskaya et al. 2016; Altwegg et al. 2019; Öberg & Bergin 2021), and we therefore expect mixed ices to be present in the protoplanetary disks.
The presence of ice mixtures introduces additional factors that regulate the solid/gas division, including entrapment of more volatile species, e.g., CO, CH4, N2, and noble gases, into less volatile ice matrices, such as H2O and CO2 (e.g., Bar-Nun et al. 1988; Collings et al. 2004), where the hypervolatiles might remain until sublimation of the ice matrix. As a consequence, hypervolatiles may be available in solid form closer to the star within the disk than otherwise expected. Understanding ice entrapment in protoplanetary disks is then necessary to predict planet and planetesimal compositions as well as planetary atmospheres. In the solar system this process may be imprinted on both planet and planetesimal compositions. In particular, entrapment of hypervolatiles has been used to explain the abundance of CO, CO2, N2, NH3, O2, HCN, and noble gases in comets (Owen et al. 1999; Villanueva et al. 2011; Rubin et al. 2015b; Ninio Greenberg et al. 2017; Mousis et al. 2017; Rawlings et al. 2019; Cordiner et al. 2020), Jupiter's composition, and Titan's atmospheric inventory (Niemann et al. 2010; Raymond & Morbidelli 2022).
Efficient entrapment has been experimentally measured for a number of hypervolatiles in water ice, i.e., CO2, CO, N2, O2, CH4, and noble gases (Bar-Nun et al. 1987, 1988, 2007; Notesco & Bar-Nun 1996; Collings et al. 2003b, 2004; Gálvez et al. 2008; Edridge et al. 2013; Burke et al. 2015; Lauck et al. 2015; Ninio Greenberg et al. 2017, 2019; Laufer et al. 2017; Maté et al. 2020). Entrapment in water ice is observed whether the ice mixture is codeposited or the hypervolatile is deposited on top or underneath the ice matrix (Collings et al. 2004; Maté et al. 2009). As a body of work, these experiments show that entrapment is possible for different hypervolatiles, for a range of ice thicknesses, dilutions (∼1:1 to 100:1 matrix to hypervolatile ratio), and mixture compositions. Two limitations with the existing literature are the focus on water as an ice matrix, and an unclear dependence of the entrapment efficiency on different ice parameters. With regard to the former, Ninio Greenberg et al. (2017) and Simon et al. (2019) recently demonstrated that CO2 may also act as an efficient entrapment agent, which may further enrich the inner disk regions with hypervolatiles.
In this study, the goal is to quantify the entrapment efficiencies of water and CO2 ices for the hypervolatiles CO, CH4, N2, and Ar. We use a large experimental parameter study to explore how the entrapment efficiency depends on the chemical composition and thickness of the ice matrix, the nature of the hypervolatile, the matrix:hypervolatile mixture ratio, and the ice deposition temperature. The paper is organized as follows. In Section 2, the experimental setup and experimental procedures are presented. The entrapment results are given in Section 3, where we quantify the hypervolatiles' entrapment efficiency in CO2 and water ice as a function of composition, mixing ratio, ice thickness, and deposition temperature. Our updated understanding of ice entrapment and the astrophysical implications are discussed in Section 4.
2. Methods
2.1. Experimental Setup
The experimental setup used for this work is designed to investigate thermal processes in interstellar ice analogs and is shown in Figure 1. It is colloquially referred to as SPACE–KITTEN (Surface Processing Apparatus for Chemical Experimentation--Kinetics of Ice Transformation in Thermal ENvironments) due to its resemblance to our larger setup SPACECAT (Lauck et al. 2015). The setup was previously described in Simon et al. (2019) but is here presented in more detail.
Figure 1. (a) 3D sketch of the complete setup used in this study except for the pump stations and frame. (b) Zoom-in sketch of the sample substrate holder present at the center of the chamber. (c) Photograph of the experimental setup. (d) 3D sketch of the gas mixing line connected to the chamber.
Download figure:
Standard image High-resolution imageSPACE–KITTEN consists of a 65 ultrahigh vacuum (UHV) chamber reaching a base pressure of ≈4 × 10−9 Torr at room temperature. The vacuum is monitored by an MKS series 999 Quattro multisensor vacuum transducer, coupled with a series PDR900 single-channel controller, which provides a continuous pressure measurement from 10−10 Torr to atmospheric pressure. The UHV chamber is pumped using a turbomolecular pump (Pfeiffer Vacuum Inc. HiPace 400 with pump speed of 350 liter s−1 for N2) backed by a Kashiyama NeoDry 15E dry pump with a maximum pumping speed of 250 liter minute−1.
An IR-transparent 2 mm thick CsI window (075 clear view), mounted on an optical ring sample holder, is suspended at the center of the chamber and attached to a closed-cycle helium cryostat (Advanced Research Systems model DE- 204S, Figure 1(b)) with a DMX-20B interface. The DMX-20B interface uses helium exchange gas to decouple the sample holder from the cold tip of the DE-204 cryocooler. This prevents most of the vibrations from the cold head being transferred to the CsI window and the IR spectrometer. The cryostat assembly can be rotated 360° using a differentially pumped rotary seal (Thermionics Vacuum Products, RNN-400).
A nickel-plated copper-polished OFHC (oxygen-free high-conductivity copper) radiation shield is attached to the first stage of the cryostat, enabling the CsI substrate to reach base temperatures of 12 K. The sample temperature is controlled between 12 and 350 K with ±0.1 K precision and ∼2 K absolute accuracy using a Lakeshore Model 335 temperature controller. A 75 W resistive thermofoil heater mounted above the sample holder is used to heat the CsI substrate. A calibrated Si diode sensor (LS-670B-SD) is located on the cryocooler tip near the heater. A second Si diode sensor is mounted directly to the CsI substrate to measure the ice temperature.
SPACE–KITTEN is equipped with a QMG 220M2, PrismaPlus Compact quadrupole mass spectrometer (QMS) from Pfeiffer Vacuum with a Faraday detector as well as secondary electron multiplier detector. The QMS is situated in proximity to the sample holder, and has a mass range from 1 to 200 atomic mass units (amu). It is used to analyze the gas composition in the chamber both during ice deposition and during ice sublimation.
SPACE–KITTEN also employs a Fourier transform infrared spectrometer (FTIR, Bruker Vertex 70) with a liquid N2-cooled mercury cadmium telluride detector in transmission mode to measure the abundance of infrared-active species in the ice. The design of the SPACE–KITTEN incorporates the UHV chamber inside the sample compartment of the FTIR spectrometer such that the IR optical path overlaps with the horizontal axis of the chamber. The UHV chamber and the spectrometer are interfaced with two differentially pumped IR-transparent KBr windows on each side along with vibration damping mounts. The entire optical path of the IR chamber is continuously purged with pure dry nitrogen gas using a Parker 75–62 purge gas generator.
Ices are grown on the cold CsI substrate by condensation of gas mixtures. The gas mixtures are mixed in a stainless steel gasline (Figure 1(d)) prior to ice deposition (see below for the detailed procedure). Ice growth is monitored by FTIR (IR-active species) and QMS (IR-inactive species). The gasline is pumped to pressures <5 × 10−4 Torr using a turbopump station (Pfeiffer Vacuum Inc. HiCube Eco station with pump speed of 67 liter s−1 for N2). The pressure in the gasline is monitored using two active capacitance transmitters (Pfeiffer Vacuum Inc.) CMR 361 and CMR 365 from 5 × 10−4 to 1100 Torr. The gas mixture is typically introduced at a normal incidence to the substrate through a 4.8 mm diameter stainless steel tube doser positioned 2 inches from the substrate. The gas doser is attached to a VAT variable leak valve and compact z-stage (MDC Vacuum Products, LLC). The number of molecules deposited is monitored using a combination of FTIR and QMS measurements.
2.2. Experimental Procedure
The experimental series were designed to compare the entrapment efficiencies of CO2 and H2O ice for four different hypervolatiles: 13CO, 12CH4, 15N2, and Ar. To simultaneously evaluate the role of ice thickness and mixing ratio in entrapment efficiency we ran experiments at two different thicknesses (10 and 50 monolayers, ML) and two different ratios (3:1 and 10:1) for all matrix:hypervolatile combinations, resulting in a total of 32 unique experiments. We repeated a subset of these experiments at different deposition temperatures, and ran selected experiments in triplicate to estimate experimental uncertainties.
The experiments were performed using 12CO2 (99.9% purity, Sigma), 13CO (99% purity, Sigma), 12CH4 (99.9% purity, Sigma), 15N2 (98% purity, Sigma), Ar (99.95% purity, Sigma), and deionized H2O. H2O is purified using multiple freeze–thaw cycles with liquid nitrogen. We use 15N2 (m/z = 30) rather than 14N2 (m/z = 28), since the latter can be confused with carbon monoxide (12C16O), a potential fragment of CO2 in CO2:15N2 mixtures. For the same reason, 13CO is used instead of 12CO. Finally, when monitoring 12CH4 gas we focus on m/z = 15 since m/z = 16 also traces oxygen due to H2O or CO2 fragmentation in the QMS.
In order to create the desired composition, the gas mixtures are prepared within an hour of the experiment inside the gasline attached to the chamber (Figure 1(d)). For each binary mixture, a specific amount of each gas is introduced into the gasline and collected in flasks with predetermined volumes. For a matrix:hypervolatile ratio of 3:1, we put 12 Torr of the matrix gas in the designated flask and 4 Torr of the hypervolatile. In the case of a 10:1 ratio, we put 14.5 Torr of matrix gas in the flask and 1.5 Torr of the hypervolatile. After measuring the desired amount of each gas, the two substances are allowed to mix in the gasline volume by releasing the quantities from the flasks. The gas mixture is allowed to equilibrate for up to an hour. Then, the gas mixture is deposited onto the cryocooled substrate at 12 K (or 20–30 K when investigating the role of the deposition temperature) until the desired thickness is reached. The process takes ≈3–15 minutes, depending on the ice thickness aimed for. During deposition we monitored the gas mixture composition using the QMS and we saw no significant changes in the mixing ratio between the beginning and end of the deposition process. For mixtures containing CO or CH4, we also acquired multiple FTIR spectra during deposition, and inspected those for ice mixture consistency over time. Also with this method we saw no changes in mixing ratio during ice deposition. We calculate the absolute ice mixing ratio for IR-inactive species using the QMS calibration described in Section 2.3.
The thickness of the deposited ice matrix is determined using FTIR. For all experiments, the infrared spectra are taken with a resolution of 1 cm−1 from 800 to 4000 cm−1. All spectra presented are averages of 128 scans, which take approximately 3 minutes to complete. Figure 2 shows representative infrared spectra for H2O:13CO (top) and CO2:13CO (bottom) ice mixtures. The absorption bands employed in this study are listed together with their characteristics in Table 1. The band positions and strengths are taken from Bouilloud et al. (2015).
Figure 2. Infrared spectra of H2O:13CO (upper panel) and CO2:13CO (lower panel) ice mixtures at ∼50 ML at 3:1 ratio. The 13CO peak is zoomed-in in the rectangular boxes to show its structure.
Download figure:
Standard image High-resolution imageTable 1. Integration Ranges and Band Strengths
Species | IR Band | Band Position (cm−1) | A (cm) |
---|---|---|---|
13CO | C–O str. | 2080–2110 | 1.4 × 10−17 |
12CH4 | C–H str. | 1290–1320 | 8.4 × 10−18 |
12CO2 | C–O str. | 2320–2380 | 1.3 × 10−16 |
H2O | O–H str. | 3000–3600 | 2.2 × 10−16 |
Download table as: ASCIITypeset image
The thickness measurement starts by recording the background spectra before ice deposition. During mixture dosing, FTIR spectra are continuously recorded, and the dosing is stopped when the H2O or CO2 ice band has reached the desired strength for the targeted ice thickness. The ice thickness is calculated using

where Ni is the column density (molecule cm−2), θ is the angle of incidence between the IR field vector and the ice surface normal, ∫τi (v)dv is the integrated optical depth of the IR band area, and Ai is the band strength of the species. For this setup θ is 55° when the sample holder faces the doser and 0° when it faces the FTIR. The column density in molecules cm−2 is converted to monolayers of ice (ML) by using a standard conversion factor of 1015 molecules cm−2. For the IR-active hypervolatiles 12CH4 and 13CO, we also used IR spectroscopy to determine the precise ice mixing ratio achieved in each experiment.
Two sources of errors are relevant to IR-based determinations of ice thickness and mixing ratio: spectral line fit errors (including baseline selection) and band strength uncertainties. Formal spectral line fit errors are always insignificant compared to other sources of error, since our selected spectral features are relatively isolated, and they never exceed 1%. The uncertainties of ice thicknesses and relevant ice mixture ratios are instead dominated by band strength uncertainties of ∼20% due to variations in IR band strengths with temperature and ice mixture composition (Gerakines et al. 1995; Bouilloud et al. 2015).
Following ice deposition, the ice mixture is heated in a temperature programmed desorption (TPD) experiment using a linear heating ramp of 1 K minute−1 until ice desorption is complete (based on the IR spectra). During warm-up, the desorption rates of the different ice components are monitored using the QMS. We also acquired an IR spectrum every 5 minutes, which can be used to assess the ice composition and structure during heating.
Entrapment is measured using the QMS TPD curves. The integrated area of a TPD curve between two time stamps is proportional to the number of molecules desorbed during that time as long as the TPD curve can be uniquely assigned to one species. The entrapment efficiency is determined based on the integrated curve above some temperature threshold compared to the integrated area of the entire TPD curve. Figure 3 shows representative TPD curves for H2O:13CO (top) and CO2:13CO ice mixture (bottom). The two ice matrices desorb at distinct temperatures, while 13CO desorption is more complex. The 13CO line shows different numbers of desorption peaks depending on the mixture. In the case of water, two well-separated 13CO peaks are observed, the first one corresponding to the molecules desorbing close to the pure 13CO desorption temperature. The second peak coincides with the onset of water crystallization (Collings et al. 2004) and is due to 13CO entrapment in H2O. In the case of the CO2:13CO mixture, the 13CO line presents multiple peaks. We only consider 13CO codesorbing with CO2 (>70 K) trapped. The trapped fraction of hypervolatile (13CO in this case) is calculated from the integrated area of the hypervolatile's "trapped" peak (i.e., the peak desorbing at the same temperature as the ice) divided by the integrated area of the hypervolatile's entire curve.
Figure 3. TPD curves of H2O:13CO (upper panel) and CO2:13CO (lower panel) ice mixtures. In both panels the orange solid or dashed curve traces m/z = 29, i.e., 13CO. In the upper panel the solid purple curve is m/z = 18, i.e., H2O, and in the lower panel the dashed purple line traces m/z = 44, i.e., CO2. The 13CO peak in the rectangular boxes is the quantity considered as trapped.
Download figure:
Standard image High-resolution image2.3. Calibration of the QMS Signal for the Infrared-inactive Species
In the case of 13CO and 12CH4 the ice mixture ratio can be verified through infrared spectroscopy since those species are infrared-active. Because 15N2 and Ar are IR-inactive we instead use the integrated TPD curves to calculate the achieved ice mixing ratio. The integrated ion current measured by a QMS corresponding to a mass fragment m/z of a given species desorbed during TPD experiments is proportional to the total number of molecules desorbed. Following Martín-Doménech et al. (2015), the relationship between QMS signal and ice column density can be calculating for a species X using

where A(m/z) is the integrated area under the QMS signal of a given mass fragment m/z during thermal desorption; σ+(X) is the ionization cross section for the first ionization of the species (i.e Ar, 15N2, or 13CO) at the incident electron energy of the mass spectrometer (i.e., 70 eV for our setup); N(X) is the total number of desorbed molecules in ML; IF (X) is the fraction of ionized molecules with charge z, which is ≈1 for the considered species; FF (X) is the fractionation pattern, i.e., the fraction of molecules leading to a fragment of mass m in the mass spectrometer; S(m/z) is the sensitivity of the QMS to the mass fragment m/z; and kQMS a proportionality constant for this experimental setup.
To determine the experiment-specific factor, kQMS, we follow Martín-Doménech et al. (2015) and use 13CO TPD ice experiments under UHV conditions to calibrate the QMS signal. Table 2 summarizes all the other parameters for 13CO, 15N2, and Ar. Any QMS measurement can then be expressed relative to CO:
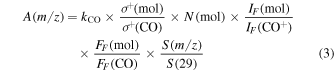
where kCO is the proportionality constant:

Equations (2) and (3) are equivalent, since
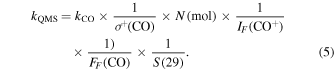
Table 2. Summary of the Calibration Parameters
X | σ+(mol) a | FF (X) a | IF (X) | m/z | S(m/z) d |
---|---|---|---|---|---|
13CO | 2.516 | 0.949 | 1 b | 29 | 3.17 × 1014 |
15N2 | 2.508 | 0.933 | 1 a | 30 | 3.10 × 1014 |
Ar | 2.520 | 1 | 0.948 c | 40 | 2.47 × 1014 |
Notes.
a Extracted from the online database of the National Institute of Standards and Technology (NIST). b We assumed that no double ionization of the molecules took place in the QMS. c Rejoub et al. (2002). d Sensitivity of the QMS calculated for our experimental setup.Download table as: ASCIITypeset image
CO is the chosen reference species since its ice abundance is easily determined using IR spectroscopy. We calculated kCO based on both H2O and CO2 ice mixture experiments and found slightly different values of (2.03 ± 0.24) × 10−9 and (2.41 ± 0.29) × 10−9, respectively, where the error comes from the dispersion based on multiple experiments for each kind of mixture. The dispersion of kCO is ≈12% and we add the uncertainty of kCO in quadrature with the CO band strength uncertainty to obtain uncertainties in ice column density for 15N2 and Ar of ∼23%.
2.4. Entrapment Uncertainties
We estimate the uncertainty of measured entrapment efficiencies based on the dispersion between similar experiments. To calculate these dispersion-based uncertainties, we ran multiple experiments for all matrix and hypervolatile combinations at 50 ML using a 3:1 ratio, and also at 50 ML for a ratio of 10:1 for CO2:13CO and H2O:13CO (Table 5 in the Appendix). Figure 4 shows the results for the 13CO dispersion experiments. We observe fractional uncertainties in the measured entrapment efficiency between 0.02 and 0.19. The lowest dispersions are seen for dilute (10:1) experiments and experiments with an H2O ice matrix, while CO2 ice matrix experiments and 3:1 experiments have higher dispersions. There is no obvious trend with the kind of hypervolatile and we therefore apply a fractional uncertainty of ∼0.2 for CO2:hypervolatile 3:1 mixtures, a fractional uncertainty of ∼0.1 for H2O:hypervolatile 3:1 mixtures, and a fractional uncertainty of 0.04 for all 10:1 experiments.
Figure 4. TPD profiles of 50 ML CO2:13CO and H2O:13CO mixtures in ratios of 3:1 and 10:1 used to determine of entrapment uncertainties.
Download figure:
Standard image High-resolution image3. Results
3.1. Overview of Hypervolatile Desorption and Entrapment
Table 3 lists the experiments used for determining hypervolatile entrapment into CO2 and H2O ice matrices, deposited at the fiducial substrate temperature of 12 K. We ran four experiments for each matrix:hypervolatile mixture using two different ratios of 3:1 and 10:1, and two different ice matrix thicknesses of 10 and 50 ML for each ratio. Figure 5 shows the TPD profiles of each hypervolatile, 13CO, 12CH4, 15N2, and Ar, desorbing from ice matrices of H2O (top panels) and CO2 (bottom panels). The experiments are further organized based on ice thickness and ice mixing ratio. Within each panel, and throughout the paper, the TPD traces are color-coded: orange for 13CO, crimson for 12CH4, purple for 15N2 and black for Ar.
Figure 5. TPD data for the experiments listed in Table 3 organized by ice matrix (H2O top and CO2O bottom panels), and ice thickness and ice mixing ratio (left to right). Within each panel, orange is for 13CO, crimson for 12CH4, purple for 15N2, and black for Ar.
Download figure:
Standard image High-resolution imageTable 3. Fiducial Ice Entrapment Experiments
HV | Ratio | Matrix | Volatile | Entrapment |
---|---|---|---|---|
(ML) | (ML) | (%) | ||
CO2 | ||||
5.0:1 | 11 | 2.3 | 33% ± 7% | |
13CO | 3.3:1 | 55 | 17 | 35% ± 7% |
12:1 | 12 | 0.98 | 48% ± 1% | |
10:1 | 56 | 5.5 | 65% ± 1% | |
2.5:1 | 17 | 6.7 | 14% ± 3% | |
12CH4 | 3.2:1 | 57 | 18 | 24% ± 5% |
9.4:1 | 13 | 1.3 | 43% ± 1% | |
14.5:1 | 56 | 3.8 | 57% ± 1% | |
3.4:1 | 11 | 3.3 | 19% ± 4% | |
15N2 | 3.2:1 | 54 | 17 | 40% ± 8% |
9.8:1 | 9.3 | 0.95 | 29% ± 1% | |
11.7 | 55 | 4.7 | 61% ± 1% | |
2.5:1 | 11 | 4.3 | 24% ± 5% | |
Ar | 2.8:1 | 52 | 18 | 30% ± 6% |
11.4:1 | 12 | 1.1 | 40% ± 1% | |
11.3:1 | 50 | 4.4 | 57% ± 1% | |
H2O | ||||
5.0:1 | 12 | 2.4 | 27% ± 3% | |
13CO | 3.0:1 | 53 | 16 | 29% ± 3% |
10:1 | 13 | 1.3 | 38% ± 2% | |
10:1 | 53 | 5.3 | 61% ± 2% | |
2.6:1 | 11 | 4.4 | 15% ± 2% | |
12CH4 | 2.5:1 | 53 | 24 | 30% ± 3% |
9:1 | 12 | 1.3 | 46% ± 2% | |
11.9:1 | 55 | 4.6 | 63% ± 3% | |
2.9:1 | 12 | 4.1 | 15% ± 2% | |
15N2 | 2.9:1 | 54 | 17 | 19% ± 2% |
11:1 | 10 | 0.95 | 43% ± 2% | |
12:1 | 73 | 6.1 | 58% ± 2% | |
4.7:1 | 19 | 4.0 | 24% ± 2% | |
Ar | 2.8:1 | 50 | 18 | 43% ± 4% |
11:1 | 12 | 1.1 | 36% ± 1% | |
13:1 | 52 | 4.0 | 37% ± 2% |
Download table as: ASCIITypeset image
In the H2O:hypervolatile experiments, the TPD profiles show two desorption peaks: the first one is at 35–40 K and the second at ∼140 K. These peaks correspond to hypervolatile desorption from a water ice surface and hypervolatile entrapment. The ∼140 K peak does not correspond to desorption from water ice, however, but rather to the crystallization of water ice. Substantial entrapment is observed in all H2O ice matrix experiments.
In the CO2 ice matrix experiments, the hypervolatiles generally desorb in three or more temperature ranges, with a first desorption peak at 35–45 K, corresponding to hypervolatile desorption from CO2 ice. The last major desorption peak occurs around 80–85 K, corresponding to hypervolatiles entrapped in the CO2 ice matrix, and the presence of an ∼80 K peak in all CO2 experiments demonstrates that CO2 is capable of entrapping all considered hypervolatiles under a range of ice conditions. In the CO2 experiments we also observe some hypervolatile desorption between these two peaks, at ∼45–70 K. The precise temperature of this desorption feature depends on the experimental conditions. In the 50 ML, dilute experiments, the desorption peak is more subtle and shifts to higher temperatures, while in the concentrated, thin (10 ML) ice mixtures the desorption pattern is more complex, with several intermediate desorption peaks.
3.2. Entrapment Efficiencies for Cold-deposited (12 K) Ices
Figure 6 shows and Table 3 reports calculated entrapment fractions of the experiments displayed in Figure 5. Entrapment efficiencies vary between 14% and 65% of the initial hypervolatile abundance. This range is almost identical for the CO2 and H2O matrix experiments. We first compare how the entrapment efficiency of each hypervolatile in each ice matrix depends on experimental parameters (Figure 6, upper panels). For each ice-matrix:hypervolatile, the entrapment efficiency increases with ice thickness and hypervolatile dilution. One exception to this general rule is that CO2:13CO and CO2:Ar exhibit the same entrapment efficiencies for both ice thicknesses when the matrix:hypervolatile ratio is 3:1; another is that for argon in H2O, entrapment efficiencies stay almost constant at ∼35% for the different experimental parameters. The average entrapment efficiencies in the four kinds of experiments are ∼20% (3:1, 10 ML), ∼30% (3:1, 50 ML), ∼41% (10:1, 10 ML), and ∼55% (10:1, 50 ML), respectively.
Figure 6. Histograms showing the percentage of entrapment in CO2 and H2O ice matrices for the experiments listed in Table 3. The bars are color-coded by hypervolatile, while a solid bar indicates a CO2 matrix and a patterned bar an H2O matrix. Top panels: comparison of entrapment efficiencies between different experimental conditions for each hypervolatile. Bottom panels: comparison of entrapment efficiencies between the four hypervolatiles for each experimental condition.
Download figure:
Standard image High-resolution imageFigure 6 (bottom panels) rearranges the data from the upper panels to instead show how entrapment efficiencies of different hypervolatiles compare under identical experimental conditions. No hypervolatile is consistently the least or most entrapped, and in most cases the entrapment efficiency is constant within uncertainties for the different hypervolatiles. The exceptions to the latter are the significantly lower entrapment of N2 in 10 ML 10:1 CO2 ice and Ar in 10:1 50 Ml ices in H2O ice compared to other hypervolatiles. We note, however, that in both cases the mixing ratio is less well constrained due to the lack of IR spectroscopy of the two hypervolatiles, which may in part explain these deviations. We also observe that in the case of CO2 matrices, CO is consistently better trapped than Ar and N2.
In Figure 7, we further rearrange the data in Figure 6 to focus on comparing entrapment in H2O and CO2 matrices for the different experimental conditions and hypervolatiles. For each set of parameters, CO2 and H2O trap approximately the same amount of volatile, with the exception of 10:1 50 ML CO2/H2O:Ar and 3:1 50 ML CO2/H2O:15N2, where the entrapment appears significantly higher in the CO2 ice matrix. We also note that 13CO is the only case for which entrapment in CO2 is more efficient than water for each set of parameters, but the individual differences are within uncertainties. Overall neither H2O nor CO2 is clearly a better entrapment agent; however, CO2 has the higher entrapment efficiency in 9/16 pairwise comparisons, and H2O has the higher one in 7/16.
Figure 7. Comparison of entrapment in CO2 and H2O ice matrices for each hypervolatile, ice thickness, and mixing ratio.
Download figure:
Standard image High-resolution image3.3. Dependences of Entrapment Efficiencies on the Ice Deposition Temperature
To complement the parameter study above, we ran a set of experiments where we kept the ice thickness and ice mixing ratio constant at ∼50 ML and 10:1, but increased the ice deposition temperature to 20 or 30 K. 30 K is just below the desorption temperature of the most volatile of our hypervolatiles from a water ice surface, and therefore sets the upper bound. Table 4 lists the experimental parameters and Figure 8 shows the results. We chose the 10:1 ratio and 50 ML ice, since the entrapment efficiencies have the smallest error bars for this set of parameters, as well as the highest entrapment efficiency for each hypervolatile. For most combinations of matrix and hypervolatile there is no discernable trend with deposition temperature between 12 and 30 K. The most notable exception is 12CH4 entrapment in CO2, which decreases by almost a factor of two between 12 and 30 K. We also note a smaller decrease, of ∼15%, in 13CO entrapment in H2O ice at 20 and 30 K, compared to 12 K, and a small increase in Ar entrapment at 20 and 30 K, compared to 12 K.
Figure 8. Comparison of CO2 and H2O ice matrices for each experiment. Each panel shows the comparisons of one compound at the different combination of parameters.
Download figure:
Standard image High-resolution imageTable 4. Experiments Relevant to Section 3.3 on the Role of the Ice Deposition Temperature
Date | Deposition Temp. | Ice | Volatile | Ratio | Thickness of Ice | Thickness of Volatile | % Entrapment |
---|---|---|---|---|---|---|---|
05/29/21 | 15 K | CO2 | 13CO | 11:1 | 53 | 4.9 | 65% |
05/27/21 | 20 K | CO2 | 13CO | 11:1 | 59 | 5.3 | 64% |
05/31/21 | 25 K | CO2 | 13CO | 13:1 | 54 | 4.0 | 60% |
05/27/21 | 30 K | CO2 | 13CO | 10:1 | 55 | 5.4 | 67% |
05/21/21 | 15 K | CO2 | 12CH4 | 12:1 | 51 | 4.2 | 54% |
05/21/21 | 20 K | CO2 | 12CH4 | 13:1 | 52 | 3.9 | 60% |
05/21/21 | 25 K | CO2 | 12CH4 | 9.0:1 | 52 | 5.7 | 37% |
05/21/21 | 30 K | CO2 | 12CH4 | 8.8:1 | 51 | 5.9 | 35% |
05/24/21 | 20 K | CO2 | 15N2 | 19:1 | 62 | 3.2 | 67% |
05/24/21 | 30 K | CO2 | 15N2 | 18:1 | 58 | 3.3 | 60% |
05/25/21 | 20 K | CO2 | Ar | 13:1 | 58 | 4.5 | 59% |
05/25/21 | 30 K | CO2 | Ar | 9.9:1 | 58 | 5.9 | 52% |
05/27/21 | 20 K | H2O | 13CO | 10:1 | 52 | 5.5 | 46% |
05/27/21 | 30 K | H2O | 13CO | 10:1 | 55 | 5.5 | 46% |
05/25/21-B | 20 K | H2O | 12CH4 | 14:1 | 47 | 3.4 | 62% |
05/25/21-B | 30 K | H2O | 12CH4 | 14:1 | 50 | 3.5 | 59% |
05/24/21 | 20 K | H2O | 15N2 | 11:1 | 46 | 4.1 | 59% |
05/24/21 | 30 K | H2O | 15N2 | 13:1 | 55 | 4.3 | 53% |
05/25/21 | 20 K | H2O | Ar | 6.1:1 | 66 | 11 | 52% |
05/25/21 | 30 K | H2O | Ar | 8.3:1 | 58 | 7.0 | 54% |
Download table as: ASCIITypeset image
4. Discussion
In this section, we discuss the entrapment behavior that we have observed experimentally, focusing on the roles of ice thickness, mixing ratio, and temperature (Section 4.1), the nature of the ice matrix (Section 4.2), and the kind of entrapped hypervolatile (Section 4.3). Finally, astrophysical implications are considered in Section 4.4.
4.1. Entrapment under Different Experimental Conditions
In the presented experiments, we varied three experimental parameters: hypervolatile concentration or matrix:hypervolatile mixing ratio, ice matrix thickness, and ice deposition temperature. Consistent with previous experiments, we found that increased dilution results in increased entrapment. For example, Fayolle et al. (2011) found an increase in CO2 entrapment by 1/3 when changing the H2O:CO2 ratio from 5:1 to 10:1, and an increase in CO entrapment by a factor of 6 when changing the H2O:CO ratio from 1:1 to 5:1. Simon et al. (2019) investigated this effect in detail for CO2:CO ices and found an increasing entrapment efficiency with dilution up to a ratio of ∼8:1, after which any additional dilution has a negligible effect on entrapment. The current study shows that this trend holds for a range of hypervolatiles in H2O and CO2 ice matrices. As discussed in detail in Simon et al. (2019), we intuitively expect a higher percentage of entrapment in more dilute ices because of a limited availability of strongly binding sites or nanopores in an ice matrix. As more hypervolatiles are added to the ice mixture, a larger proportion of hypervolatiles will likely be situated in more weakly binding sites, from which diffusion and desorption can occur more readily. This would then result in a decreasing percentage of entrapment with increased volatile concentration. Moreover, very concentrated ice mixtures, where a hypervolatile molecule is likely to have at least one hypervolatile neighbor rather than be surrounded by matrix molecules, may also introduce "veins" of hypervolatiles stretching from the ice surface into the ice. Dependent on the relative rates of hypervolatile desorption and pore collapse during warm-up, these "veins" may result in hypervolatile desorption from deeper ice layers than would otherwise be expected. Theoretical modeling is required, however, to quantitatively link hypervolatile concentration, nanopore and binding-site availability, and entrapment efficiencies (see, e.g., Ciesla et al. 2018).
The second experimental parameter, ice matrix thickness, also strongly impacts entrapment efficiencies, with thicker ice resulting in higher entrapment efficiencies. This too is consistent with theory (Ciesla et al. 2018) and studies investigating CO entrapment in CO2 and H2O ice (Simon et al. 2019), CO2 entrapment in H2O (Fayolle et al. 2011), and Ar entrapment in H2O (Notesco & Bar-Nun 2005). The latter is especially noteworthy, since it operates in a different thickness regime, with ice thicknesses between ∼0.1 and 5 μm. To explain this trend we need to consider the mechanism(s) by which hypervolatiles can escape from an ice matrix. In a rigid ice, where diffusion of molecules through the ice matrix is slow, escape should mainly occur from the ice surface and through pores connecting the ice–vacuum interface and the ice interior. Assuming that these pores have some characteristic length scale, we expect less escape and hence more entrapment for thicker ices. This may explain the trends observed for H2O ice, which does not experience any major phase changes and hence pore opening or closure until after the "natural" desorption peak of the hypervolatiles in this study (Collings et al. 2003a). The similar trends for CO2 may require a different explanation since CO2 crystallization begins at relatively low temperatures (He & Vidali 2018), and CO2 pores may open and close during hypervolatile desorption, changing the depth into the ice from which hypervolatiles can escape. Still also in this more dynamic scenario, escape should be easier closer to the ice–vacuum interface and some dependence on ice thickness would be expected. What is somewhat mysterious is that the trend appears quantitatively similar for H2O and CO2.
In the case of the third experimental parameter, deposition temperature, we may expect to see diminished entrapment with increased deposition temperature, based on the intuition that a higher temperature would allow hypervolatiles to diffuse and clump together closer to the ice–vacuum interface during ice deposition. This was not observed for ice deposition temperatures between 12 and 30 K, with the exception of CH4 in CO2 ice. A similar lack of an effect was reported by Bar-Nun et al. (1988) for thicker ice mixtures, and by Collings et al. (2003b) when measuring entrapment of CO deposited on top of an amorphous H2O ice as long as the temperature was <35 K. Furthermore, our result appears consistent with the model from Ciesla et al. (2018), taking into account that our ice deposition rates are quite high, of the order of 10 ML minute−1 or 10−2–10−3 μm per minute.
The overall consistency with both existing experiments and theory makes the one exception especially interesting: the entrapment of CH4 in CO2 decreases by a factor of two between 12 and 30 K. To ensure that this was a robust result, we repeated the CO2:CH4 experiment at 30 K deposition temperature three times, and each time obtained the same low entrapment efficiency. This behavior might be expected if CH4 bonded more weakly to CO2 than the other hypervolatiles. Based on our TPD curves, however, CH4 has a slightly higher desorption temperature than CO, N2, and Ar from CO2 ice, which typically corresponds to a higher hypervolatile–matrix binding energy, and we would therefore have expected methane to be less impacted than the other hypervolatiles by the higher ice deposition temperature. We speculate that the lower CH4 entrapment efficiency is instead due either to the size of CH4 or to some more subtle CH4–CO2 interaction. CH4 has a larger size (defined by its collision cross section) than the other hypervolatiles. A larger size may make it more vulnerable to escape because there may be fewer nanopores by which it can be absorbed into the CO2 ice compared to smaller molecules. Speaking against this explanation is the fact that while CH4 is larger than the other molecules, the difference in size is quite small and it is unclear if such a small size difference could explain the dramatic difference in entrapment efficiency. Detailed modeling is needed of both CO2 ice pore sizes and the ice dynamics during warm-up to test whether this is a plausible explanation. In addition, it would be useful to model the details of CH4 bonding in a CO2 ice matrix and whether it is distinct from the binding behavior of the other hypervolatiles.
4.2. Comparison of Entrapment Efficiency between CO2 and H2O Ice Matrices
Based on the experiments reported here as well as those of Simon et al. (2019), CO2 entraps hypervolatiles at least as well as H2O up until 70 K. We define a hypervolatile as entrapped in CO2 ice if it desorbs with CO2, and as entrapped in H2O ice if it desorbs with H2O or during H2O crystallization. This is somewhat surprising, since H2O ice is characterized by stronger matrix bonds, which intuitively might result in more efficient entrapment. More generally we consider three aspects that may affect entrapment: rigidity of the ice matrix, pore collapse dynamics, and hypervolatile–matrix interactions. Addressing the latter, Figure 9 shows the first desorption peak of the four hypervolatiles desorbing from H2O and CO2 ice matrices. For each hypervolatile, the desorption peaks are offset by at most a few degrees between the two ice matrices, demonstrating very similar binding energies for the hypervolatiles to H2O and CO2. This result is consistent with the similar ice entrapment efficiencies of H2O and CO2.
Figure 9. TPD curves of hypervolatiles from CO2 (dashed) and H2O (solid) 50 ML ice matrices with an initial 10:1 mixing ratio showing the temperature of the first desorption peak, when hypervolatiles that are not entrapped desorb from the ice surface and out of ice pores.
Download figure:
Standard image High-resolution imageA high degree of ice matrix rigidity should result in entrapment for hypervolatiles that are closed off to the ice–vacuum interface, which should favor entrapment in water ice over the more loosely bound CO2 ice matrix. Additional entrapment may occur, however, due to pore collapse prior to or during hypervolatile desorption. H2O ice is known to experience such pore collapse in the 30–70 K regime, i.e., during "natural" hypervolatile desorption (Collings et al. 2003b). This too should favor entrapment in H2O ice. The porosity structure of cold-deposited CO2 ice has not been as well studied, but CO2 clearly experiences a major reorganization in the same temperature regime, which appears connected to CO2 crystallization (He & Vidali 2018). This kind of restructuring may have both constructive and negative impacts on entrapment. Similar to the pore collapse experienced by H2O ice, the CO2 ice crystallization may lock in hypervolatiles that were initially exposed to the ice–vacuum interface due to ice porosity. Crystallization may also expose previously buried hypervolatiles, resulting in a so-called "volcano" desorption as is seen for hypervolatiles trapped in H2O ice around H2O crystallization. A priori there is little reason to expect close to identical entrapment fractions for these two ice matrices, considering their different rigidities and dynamics in the considered temperature interval, and the fact that the entrapped hypervolatiles have "natural" desorption temperatures ∼10 K apart (Figure 9). More theory on entrapment in both H2O and non-H2O ice matrices is clearly warranted.
While CO2 and H2O entrapment efficiencies are similar when considering the amount of hypervolatile in the ice around 70 K, the two ice matrices do differ in their entrapment efficiencies at higher and lower temperatures. At higher temperatures, the CO2 ice matrix begins to desorb, resulting in complete release of hypervolatiles, while hypervolatiles stay trapped in the H2O ice matrix above 140 K. Furthermore, the hypervolatile desorption and entrapment kinetics at lower temperatures (<70 K) are more complex in the CO2 ice mixtures due to substantial hypervolatile loss during CO2 ice restructuring and crystallization in between the "natural" hypervolatile and CO2 desorption peaks. These hypervolatiles are in some sense also trapped, even though they have not been defined as such throughout this study. If we consider all hypervolatiles that outgas during CO2 restructuring as trapped, the entrapment efficiency of CO2 increases by ∼15%–45%. This is shown in Figure 10, where the more transparent bars include the quantity trapped from ∼45 to 70 K, resulting in total entrapment efficiencies of up to ∼80%, well above those of water. We return to the potential importance of this in Section 4.4.
Figure 10. Histograms showing the percentage of entrapment in CO2 ice matrices. The bars are color-coded by hypervolatile, while a solid bar indicates a hypervolatile trapped between ∼70 and 100 K (last TPD peak), and a more transparent bar indicates a hypervolatile quantity trapped from ∼45 to 100 K (intermediate and last TPD peaks).
Download figure:
Standard image High-resolution image4.3. Entrapment Similarities and Differences for Different Hypervolatiles
Based on previous experiments it was unclear whether we should have expected similar or different entrapment efficiencies for the four hypervolatiles: Bar-Nun et al. (1988) found similar entrapment efficiencies for CO, CH4, Ar, and N2 binary ice mixtures deposited at 24–35 K, while Bar-Nun et al. (2007) found substantially lower N2 entrapment in a complex ice mixture deposited at ∼27 K. Based on the presented experiments, entrapment efficiencies are close to identical for hypervolatiles in thin H2O and CO2 ices when all other experimental parameters are constant. We speculate that the different results of Bar-Nun et al. (2007) may be due to competitive entrapment in multicomponent ice mixtures, but more experiments are warranted to test this in detail. In the meantime we next discuss why entrapment efficiencies of different hypervolatiles may be similar overall, as well as possible origins for some observed minor differences.
First we note that while the sizes, desorption temperatures, and ability to form intermolecular bonds are different for the four hypervolatiles, they are perhaps not different enough to substantially affect entrapment in cold (<35 K) ices. Figure 9 shows that the hypervolatile desorption peaks are located at ∼35–47 K for H2O and ∼39–45 K for CO2, indicative of matrix–hypervolatile bond strengths that are within 30% of one another. Importantly, we find no clear trend in entrapment efficiency with "natural" desorption temperature. For both H2O and CO2 the trend in desorption temperature is CH4 > CO > N2 > Ar, while the trends in entrapment for the 50 ML 10:1 ice mixture deposited at 12 K (which has the smallest error bars) are CH4 > CO > N2 > Ar for H2O, but CO > N2 > Ar ≈ CH4 for CO2, i.e., perhaps there is a small effect for entrapment in H2O. The overall conclusion, however, is that at low temperatures, entrapment efficiencies do not seem to depend strongly on small differences in matrix–volatile interactions.
A disconnect between low-temperature ice entrapment efficiencies and matrix–hypervolatile interactions can also be inferred from IR spectroscopy. Figure 11 shows the IR spectra of the CO2 stretching band and the H2O dangling OH band for the 50 ML 3:1 ice mixtures at 12 K. We choose to show the 50 ML 3:1 mixtures because they show the clearest spectroscopic changes, but the trends are the same for all considered experimental parameters. Compared to pure CO2, CH4 appears to have the greatest impact on the CO2 ice structure, and CO the smallest, yet CO is the better entrapped in CO2 than CH4. In the case of H2O, CO appears to produce the most dangling OH bonds, i.e., disruptions in the H2O ice matrix, and Ar the least, while CH4 with an intermediate effect was the best entrapped. This supports a scenario where low-temperature entrapment is mainly a mechanical process that depends little on the precise nature of the hypervolatile and matrix interaction. An important caveat, however, is that these experiments only contained one hypervolatile, and small differences in hypervolatile–matrix interactions may play a large role in cases where hypervolatiles compete for the best entrapment sites, which may also explain the differences between this study and Bar-Nun et al. (2007). Furthermore, we emphasize that at higher ice deposition temperatures, entrapment efficiencies appear to be much more species-dependent, and may be matrix-dependent as well.
Figure 11. Infrared spectra centered at the CO2 (ν3) band (∼2345 cm−1) on the left, and H2O dangling O–H band (∼3600 cm−1) on the right. Each panel shows the four hypervolatiles at the 3:1 50 ML parameter with the associated pure 50 ML CO2 or H2O in light gray.
Download figure:
Standard image High-resolution image4.4. Astrophysical Implication
The present study yields three results with direct astrophysical implications: (1) entrapment in ices happens for ice thicknesses as low as 10 ML, (2) CO2 entraps hypervolatiles at least as well as H2O below 70 K, and (3) in cold (<35 K) binary ices where competition between hypervolatiles is unimportant, CO, N2, CH4, and Ar are all entrapped equally well. We discuss the implications of these three results in more detail next. First we note, however, that establishing these kinds of relationships quantitatively requires large experimental studies, which enable us to identify how combinations of factors affect entrapment, such as ice thickness, ice deposition temperature, mixing ratio, and the nature of the hypervolatile and matrix.
Hypervolatile entrapment has been invoked for decades to explain and predict hypervolatile ice abundances in the solar nebula and other protoplanetary disks (Korsun et al. 2008; Biver et al. 2018; Altwegg et al. 2019; McKay et al. 2019; Rubin et al. 2020; Mousis et al. 2021). Most previous entrapment studies have focused on thicker ices, of the order of ∼1 μm (Collings et al. 2004; Notesco & Bar-Nun 2005; Edridge et al. 2013). Such ice thicknesses are appropriate for ice pebbles in mature protoplanetary disks, but may not apply to the original interstellar icy grains, which set the initial distribution of volatiles in a disk. This study shows that the entrapment efficiencies and trends previously observed for thicker ices hold also for this thinner ice regime. In particular we find entrapment efficiencies of 30%–50% in thin (10 ML) dilute ice mixtures, and even for concentrated ice mixtures (3:1) up to 30% of the initial hypervolatile abundance remains trapped until ice matrix crystallization or desorption. This implies that any mixed inherited interstellar ices should retain a substantial portion of their initial hypervolatile budget up until the H2O and CO2 snowlines.
The second result, that CO2 is an excellent entrapment agent, immediately increases the amount of hypervolatiles that could be trapped exterior to the CO2 snowline in a disk. More broadly this result suggests that entrapment is a general phenomenon, and when estimating entrapment efficiencies we need to take into account the total abundance of volatile (not hypervolatile) ice species. Though H2O ice is generally assumed to be the major ice constituent integrated across a protoplanetary disk (Boogert et al. 2015; Öberg & Bergin 2021), recent theoretical work has shown that in the outer disk ices other than H2O may sometimes locally dominate the ice budget (Price et al. 2021). A more general model of ice entrapment in different ice matrices is therefore desirable to better predict entrapment across all disk radii. Until more experiments exists it seems reasonable to assume that all these species (NH3, CH3OH, etc.) entrap hypervolatiles with similar efficiencies up until their respective snowlines, at least if the ice is cold-deposited. Additional experiments are needed to determine whether all such ice species have the same entrapment characteristics as H2O and CO2 for low ice deposition temperatures, and how the relative entrapment efficiency of different matrices changes above 30 K.
Third, we note that our finding that different hypervolatiles are similarly entrapped when deposited below 35 K is consistent with most, though not all, previous studies (Bar-Nun et al. 1988, 2007; Almayrac et al. 2022). This result can be used together with absolute entrapment percentages to constrain the origins of comets and other solar system bodies. For example, Rubin et al. (2015a, 2020) found an N2/CO ratio in the comet 67P between 5.7 × 10−3 and 2.9 × 10−2, which can be compared with the expected protosolar nebula ratio of 0.145 ± 0.048 (Lodders et al. 2009). This comparison combined with our and previous laboratory experiments immediately reveals that the N2 and CO in this comet cannot originate from a cold H2O or CO2 interstellar ice with initially similar abundances of CO and N2 mixed into it. Whether this on its own rules out a cold origin of the cometary ice is somewhat more complicated since CO and N2 may form at somewhat different times in the interstellar medium (ISM) (Öberg & Bergin 2021), and hence not necessarily become incorporated into H2O ice in the same proportions as CO and N2 abundances expected in the much later protoplanetary disk. The low CH4/H2O ratio of 0.34% in 67P is another piece of evidence indicating that the 67P H2O ice is not pristine, however, since in the ISM the typical H2O ice contains several percent of CH4 ice mixed into it (Öberg et al. 2011a; Rubin et al. 2020). Detailed modeling that takes into account both ISM chemistry and the entrapment efficiencies found here is needed to quantitatively explore this question further.
Finally we note that the current study has some important limitations and cannot on its own provide definitive data on entrapment efficiencies of hypervolatiles under all relevant nebular conditions. First, the experiments only cover thin ices, while relevant ice thicknesses for protoplanetary disks range from monolayers to macroscopic ices. Comparisons with previous experiments indicate that entrapment is efficient across multiple scales, but additional quantitative data are desirable. Second, this study only considered ice deposition temperatures up to 30 K, while relevant nebular temperatures would be between 10 K and the H2O snowline, and previous experiments have shown that ice deposition temperature is an important parameter for both absolute and relative entrapment efficiencies. Third, we only considered two ice matrices out of a larger set of relevant ones for disk and planetary environments. Fourth, we only considered pure ice matrices, while ISM observations reveal, e.g., that the H2O ice matrix often contains substantial amounts of CO2, which may affect the entrapment efficiencies of more volatile species (Boogert et al. 2015). Fifth, and perhaps most importantly, we only considered binary mixtures and can hence not take into account the possibility of competitive entrapment between different hypervolatiles (Bar-Nun et al. 2007), though we expect the latter to mainly matter for more concentrated ice mixtures where there is a scarcity of good entrapment sites. Each of these should warrant its own experimental investigation, and in the meantime any conclusions drawn based on this and other existing entrapment experiments must be considered as preliminary.
5. Conclusions
In this study we systematically investigated the entrapment efficiencies of the four hypervolatiles CO, N2, Ar, and CH4 in H2O and CO2 ice matrices using laboratory experiments with thin and thick (10 ML versus 50 ML) ices, concentrated and dilute ice mixtures (3:1 versus 10:1), and different deposition temperatures between 12 and 30 K. Our main findings are as follows.
- 1.Substantial entrapment is observed for all hypervolatiles, ice matrices, and experimental conditions: 14%–65% if the initial hypervolatile is entrapped, with lower percentages obtained for thinner (10 ML) ices and more concentrated (3:1) ice matrix:hypervolatile mixtures. Conversely the highest entrapment efficiencies are measured for thicker (50 ML) and more dilute (10:1) ice mixtures.
- 2.The entrapment efficiencies are remarkably similar for the four hypervolatiles when the ice mixtures are deposited at 12 K, indicative of a low-temperature ice entrapment mechanism that is relatively independent of the specific matrix–hypervolatile interactions. This implies that the composition of cold-deposited ice should approximately reflect the relative abundances of hypervolatiles in the gaseous phase during ice mantle build-up.
- 3.H2O and CO2 entrap hypervolatiles with a similar efficiency up to the CO2 ice desorption temperature, which means that in addition to H2O, CO2 and other common ices may play a significant role in hypervolatile entrapment in protoplanetary disks.
- 4.Entrapment efficiencies remain (mostly) constant when increasing the ice deposition temperature from 12 K to 20 and 30 K. Below the "natural" desorption temperature of hypervolatiles, the ice deposition temperature hence appears to not strongly affect hypervolatile entrapment. When combining these results with previous experiments found in the literature we further find that entrapment is an important process across a range of ice thicknesses and other ice characteristics. Incorporating entrapment into astrochemical models is crucial to correctly predict the volatile abundances across protoplanetary disks and hence the compositions of the forming planets.
Acknowledgments
The authors are grateful to Elettra Piacentino and Rafael Martin Domenech for their assistance with laboratory experiment methods and interpretation. K.I.Ö. acknowledges support from the Simons Foundation (SCOL #321183) and an award from the Simons Foundation (#321183FY19).
Appendix:
Table 5 lists a series of experiments used to calculate entrapment uncertainties, the resulting entrapment measurements, and estimated uncertainties based on the dispersion between similar experiments, all explained in Section 2.4. We repeated all experiments with 50 ML thickness and 3:1 ratio at least three times and measured the dispersion for each matrix:hypervolatile combination. The resulting fractional uncertainties vary between 0.04 and 0.19 with no clear trend among the different hypervolatiles, but a higher uncertainty for CO2 than for H2O ice matrices. We also repeated the 50 ML matrix:CO 10:1 experiments to check whether the entrapment uncertainty is dependent on ice mixing ratio and found lower fractional uncertainties than for 3:1 ice matrices. The listed H2O, CO2, CO, and CH4 thickness uncertainties have uncertainties of 20%, while Ar and N2 thickness uncertainties are somewhat higher at ∼23%.
Table 5. Entrapment Efficiency Dispersions for Identical Experiments Deposited at 12 K
Date | Mixture | Ratio | Thickness of Ice | Thickness of Volatile | Entrapment | Entrapment Uncertainty |
---|---|---|---|---|---|---|
(%/Fraction) | ||||||
11/14/2021 | CO2:13CO | 2.8:1 | 66 | 24 | 32% | 6/0.19 |
11/15/2021-A | 2.8:1 | 66 | 23 | 32% | ||
12/05/2021-A | 3.3:1 | 55 | 17 | 35% | ||
01/05/2022-A | 3.0:1 | 65 | 21 | 22% | ||
03/27/2021 | CO2:13CO | 14:1 | 51 | 3.5 | 67% | 2/0.02 |
01/06/2022-A | 10:1 | 56 | 5.5 | 65% | ||
01/06/2022-B | 7.8:1 | 50 | 6.4 | 64% | ||
03/14/2021 | H2O:13CO | 3.0:1 | 53 | 16 | 29% | 2/0.06 |
11/24/2021-B | 3.6:1 | 46 | 13 | 26% | ||
11/25/2021-A | 4.1:1 | 52 | 13 | 27% | ||
03/18/2021 | H2O:13CO | 13:1 | 51 | 3.9 | 58% | 3/0.04 |
01/17/2022-A | 11:1 | 56 | 4.9 | 63% | ||
01/17/2022-B | 10:1 | 53 | 5.3 | 61% | ||
01/11/2022-A | CO2:12CH4 | 2.5:1 | 57 | 22 | 16% | 4/0.18 |
01/11/2022-B | 2.6:1 | 57 | 22 | 21% | ||
01/11/2022-C | 2.5:1 | 59 | 23 | 23% | ||
03/31/2021 | H2O:12CH4 | 2.5:1 | 53 | 21 | 30% | 2/0.07 |
11/25/2021-C | 2.6:1 | 53 | 21 | 32% | ||
11/27/2021-A | 2.7:1 | 54 | 20 | 28% | ||
12/14/2020-B | CO2:15N2 | 2.9:1 | 51 | 18 | 25% | 3/0.08 |
03/22/2021 | 3.8:1 | 54 | 14 | 42% | ||
12/05/2021-C | 4.5:1 | 54 | 12 | 37% | ||
12/08/2021-A | 4.5:1 | 47 | 10 | 35% | ||
01/18/2022 | 3.2:1 | 54 | 17 | 40% | ||
11/27/2021-B | H2O:15N2 | 2.9:1 | 54 | 19 | 19% | 1/0.04 |
11/27/2021-C | 2.9:1 | 53 | 18 | 18% | ||
12/12/2020-B | CO2:Ar | 2.8:1 | 53 | 19 | 40% | 7/0.2 |
12/08/2021-B | 2.8:1 | 52 | 18 | 30% | ||
12/08/2021-C | 3.5:1 | 54 | 16 | 28% | ||
01/05/2022-C | 5.8:1 | 48 | 8.2 | 42% | ||
12/14/2020 | H2O:Ar | 1.8:1 | 56 | 31 | 36% | 4/0.1 |
11/28/2021 | 2.5:1 | 53 | 22 | 40% | ||
11/29/2021-A | 2.0:1 | 51 | 25 | 35% | ||
11/29/2021-B | 2.8:1 | 50 | 18 | 43% |
Download table as: ASCIITypeset image