Abstract
A very high abundance of atomic carbon in the interstellar medium (ISM), and the high reactivity of these species toward different hydrocarbon molecules including benzene, raise questions regarding the stability of polycyclic aromatic hydrocarbon (PAH) molecules in space. To test the efficiency of destruction of PAH molecules via reactions with atomic carbon, we performed a set of laboratory and computational studies of the reactions of naphthalene, anthracene, and coronene molecules with carbon atoms in the ground state. The reactions were investigated in liquid helium droplets at T = 0.37 K and by quantum chemical computations. Our studies suggest that all small and all large catacondensed PAHs react barrierlessly with atomic carbon, and therefore should be efficiently destroyed by such reactions in a broad temperature range. At the same time, large compact pericondensed PAHs should be more inert toward such a reaction. In addition, taking into account their higher photostability, much higher abundances of pericondensed PAHs should be expected in various astrophysical environments. The barrierless reactions between carbon atoms and small PAHs also suggest that, in the ISM, these reactions could lead to the bottom-up formation of PAH molecules.
Export citation and abstract BibTeX RIS
1. Introduction
Polycyclic aromatic hydrocarbons (PAHs) are generally considered to be abundant in the universe (Leger & Puget 1984; Tielens 2008). However, to date, no specific PAH molecule can be identified in astrophysical environments. To be abundant, the PAHs should be stable against different destructive mechanisms present in space. Their photostability has been intensively investigated (Jochims et al. 1994, 1999; Allain et al. 1996a, 1996b; Rouillé et al. 2015). The general conclusion of these studies is that small PAHs should be destroyed, while the larger ones, having a minimum of 30–50 carbon atoms, may survive in astrophysical environments. However, Jochims et al. (1999) have demonstrated the importance of structural properties. In that experimental study, many small classical PAHs, including naphthalene and anthracene, were predicted to be stable in H i regions.
Beside UV irradiation, the other factor reducing molecular abundances is chemical reactions. Carbon, being the fourth most abundant element in the universe, plays a key role in many astrophysical processes. The intense destruction process of carbonaceous grains and molecules in the interstellar medium (ISM) is responsible for the high abundance of free carbon atoms in their neutral or ionic form. Different estimations show that 30% and even up to more than 50% of all carbon exists in the form of atomic gas (Snow & Witt 1995; Sofia et al. 2011). Surprisingly, a high abundance of carbon atoms is found even in the center of molecular clouds, where the intensity of UV light is expected to be low (Keene et al. 1985; Ohishi et al. 1992; Tanaka et al. 2011). Moreover, no energy barrier for the reaction between carbon atoms and a broad variety of hydrocarbon molecules including benzene has been found (Kaiser et al. 1997, 1999; Bettinger et al. 2000; Kaiser & Mebel 2002; Smith et al. 2006). Therefore, reactions with carbon atoms are expected to be an efficient method of molecular destruction.
In this paper, we apply theoretical and experimental approaches to test the possibility of PAH destruction by reactions with atomic carbon. The reactions of ground state carbon atoms with naphthalene, anthracene, and coronene molecules inside superfluid helium nanodroplets are studied experimentally, as well as by quantum chemical computations.
In most cases, all reactants picked up by He droplets adopt their temperature (T = 0.37 K) on a nanosecond timescale (Toennies & Vilesov 2004). Therefore, the reactant temperature is well-known. Consequently, this technique avoids the complications inherent to reactions between non-fully equilibrated reactants. Moreover, the nanoscale size of the helium droplets offers the possibility to dope them with just one species of each reactant.
As liquid helium efficiently dissipates the reaction energy, the reactions inside liquid helium resemble those occurring on the surface of dust grains. Thus, helium droplet experiments provide direct information on surface reactions. Moreover, the investigation of a reaction inside liquid helium provides data that allow us to predict the outcome of the same reaction in the gas phase. An occurrence of a reaction inside helium droplets demonstrates that there is no energy barrier in the reaction pathway. Therefore, we can also predict the rates of such gas-phase reactions, which should be mainly defined by the collision rates. At the same time, large molecules can efficiently distribute the reaction energy over their vibrational degrees of freedom and, as a consequence, the difference between surface and gas-phase reactions will vanish.
In this way, the helium droplet isolation technique is very similar to the more conventional matrix isolation technique, which is commonly used to study chemical reactions important for astronomy (Grim & d'Hendecourt 1986; Apkarian & Schwentner 1999). However, isolation in superfluid helium droplets has several advantages compared to isolation in solid rare gas matrices. It allows the use of a few extra detection techniques. As the reaction energy is dissipated by the evaporation of helium atoms it is possible to estimate the amount of energy released in the reaction by measuring the reduction of the helium droplet size after the incorporation of the reactants (Krasnokutski & Huisken 2010, 2011; Krasnokutski et al. 2014). The helium droplets can be forwarded to a mass spectrometer where the consumption of the reactants and the formation of new reaction products can be detected. Additionally, the nanosized droplets are much better suited for a precise control of the number of reactants involved in the reaction compared to bulk matrices.
2. Experimental
The experiments were carried out in the experimental setup reported earlier (Krasnokutski et al. 2005). Helium droplets are produced by supersonic expansion of helium (Air Liquide 99.9999%) gas at a pressure of 20 bar through a 5 μm diameter nozzle. The average number of He atoms per droplet is estimated based on the data given by Toennies & Vilesov (2004). Arriving at the reaction chamber, the helium droplets are sequentially doped with the reactants.
For the incorporation of C atoms, synthetic graphite powder (Sigma Aldrich, molecular weight 12.01) or 13C powder (Carbon-13C, Sigma Aldrich, 99.2 atom% 13C) was loaded into a 20 mm long tantalum tube with an inner diameter of 1.4 mm with its ends clamped between two electrodes. The tube was made by rolling a 0.05 mm thick tantalum foil. Heating was achieved by direct current provided by a supply operated at constant power output. The source provided an intense flux of low-energy atomic carbon with only a very minor presence of other species (Krasnokutski & Huisken 2014b). In the case of the collisions with helium droplets, carbon atoms were picked up and carried to the point of PAH molecule incorporation.
Commercially available naphthalene-D8 (Sigma-Aldrich 99 atom% D), anthracene-D10 (Sigma-Aldrich 98 atom% D), and coronene (Sigma-Aldrich, 99%) were used without further purification. Naphthalene was introduced from outside into a pick-up cell placed in the main chamber. The pressure in this cell was determined with an ion gauge (Leybold Heraeus IE-20). Anthracene and coronene were placed in a 40 mm long pick-up cell. The temperature of this cell could be varied between −20°C and 400°C. The vapor pressure of the PAHs in this cell was estimated based on the dependence between temperature and vapor pressure given in Oja & Suuberg (1998). Argon (Air Liquide 99.999%) was supplied from outside in the same way as described for naphthalene.
After having passed the main chamber, where the reactants were successively incorporated into the helium droplets, the helium droplet beam arrived into a differentially pumped ultra-high vacuum (UHV) detector chamber. This chamber was equipped with an electron bombardment ionizer and quadrupole mass spectrometer. The doping of the helium droplets was monitored by a mass spectrometer. The intensity of the helium droplet beam was estimated by measuring the helium pressure in the detector chamber. The total pressure in the UHV detector chamber was recorded every second with a high-precision ion gauge (Varian UHV-24). To determine the helium pressure in this chamber, the following procedure was performed: the helium droplet beam was blocked and the pressure measured after 12 s was taken as the residual vapor pressure. This residual pressure was subtracted from the values measured when the He droplet beam was open.
3. Computations
The geometries and the vibrational frequencies of stationary points of the reactants, intermediates and possible product isomers were determined using Becke's three-parameter hybrid functional with the correlation functional of Lee, Yang, and Parr (B3LYP) implemented in the Gaussian09 package (Frisch et al. 2009). The all-electron 6-311+G(d,p) basis set was used for all atoms. The combination of these methods and basis set revealed good agreement with the experimental results obtained for the interaction of PAHs with Li ions (Lee et al. 2011). Relative energies were determined as the difference of electronic and zero point energies.
4. Results and Discussion
Figure 1 shows a comparison of the differential mass spectra obtained for the reactions of carbon atoms with benzene and naphthalene. Our mass spectrometric studies of the 13C + C10D8 reaction reveal results which are very similar to the 13C + C6D6 reaction studied previously (Krasnokutski & Huisken 2014c). As can be seen from the figure, we observe in both cases a depletion of the signal on the masses of the benzene or naphthalene molecules (negative peaks) and the appearance of two new peaks on the masses of the reaction products, which result from the addition of 13C to either of the molecules (positive peaks). The ratios between the two peaks belonging to the products with and without hydrogen abstraction are also quite similar in both cases.
Figure 1. Differential mass spectra for the reactions of 13C atoms with benzene (a) and naphthalene (b). The differential mass spectra reveal the effect of carbon incorporation into the helium droplets containing benzene (naphthalene) molecules.
Download figure:
Standard image High-resolution imageSimilar to the reaction of benzene molecules, the loss of a hydrogen atom from the CC or CH insert structure requires a considerable amount of energy (376 or 343 kJ mol−1, respectively). We attempted to detect this energy in the experiment. The dependences of the ion signals on the masses of 13CC10D8 and 13CC10D7 as a function of the helium droplet size are very similar (see Figure 2). The formation of products associated with higher energy release requires larger helium droplets to dissipate this energy. Therefore, ion signals of such products should be much weaker when small helium droplets are used. This is not observed in our experiment and we can conclude that the amount of energy released in the reactions leading to the appearance of both products is very similar. Therefore, the formation of ions observed in the experiment is the result of fragmentation of the main 13CC10D8 product of the low-temperature chemical reaction.
Figure 2. Dependence of the ion signals of the products of the C10D8 + 13C reaction as a function of the helium droplet size. The curves are normalized to that for the largest droplet size.
Download figure:
Standard image High-resolution imageHowever, it should be noted that there is not a complete match between the two curves in Figure 2. The small variance between the curves is assigned to a contamination of the naphthalene sample with water. Due to the similar vapor pressures of both substances, it was difficult to completely remove the water from the naphthalene sample. As a result, water molecules are also incorporated into a few percent of the helium droplets. The probability of water molecule incorporation (and consequently of complex formation between embedded molecules and water) increases with the size of the helium droplets. As the formation of complexes with water protects the species from fragmentation in the ionizer (Ren & Kresin 2008), larger helium droplets demonstrate less fragmentation of the product of the investigated 13C + C10D8 reaction. This effect was confirmed by increasing the water vapor pressure, which resulted in a considerable enhancement of the discrepancy between both curves. For large droplet sizes (>15,000 He atoms), the ion intensity on the mass of 13CC10D8 (149 amu) became even higher than on the mass of the fragment (147 amu).
Due to the high sticking probability of naphthalene molecules to the walls of the experimental setup, it was impossible to carry out the same calorimetric studies as for the 13C + C6D6 reaction presented by Krasnokutski & Huisken (2014c). Instead, we had to confine the naphthalene sample and vapor to the pick-up cell and let the conditions stabilize for at least one hour. Then we monitored the depletion of the helium droplet beam caused by the incorporation of the carbon atoms. The results are shown in Figure 3. Since carbon atoms were produced by evaporation at high temperature and thus have relatively high translation energy, their pick-up caused a considerable depletion of the He droplet beam, namely 9.3%. However, when both reactants were incorporated, the depletion caused by the pick-up of carbon atoms was more pronounced (12.6%). This indicates that there is an extra amount of energy which is released when both reactants are incorporated into the He droplets. The small amount of energy which is released for example as a result of a physisorption process cannot be detected by this technique (Krasnokutski & Huisken 2010, 2014a). It demonstrates the formation of new chemical bonds in the 13C + C10D8 reaction. Unfortunately, in this experiment, we were not able to determine the exact amount of this energy.
Figure 3. The black solid line shows the intensity of the He droplet beam, measured via the He vapor pressure in the detector chamber. The depletion peaks originate from the doping of the helium droplets by carbon atoms. The dashed blue line shows the naphthalene vapor pressure inside the pick-up cell. The experiment was performed with helium droplets produced at a nozzle temperature of 11 K.
Download figure:
Standard image High-resolution imageTo better understand the reaction between carbon atoms and naphthalene molecules, we performed a quantum chemical modeling of the possible reaction pathways. Due to the lower symmetry of the naphthalene molecule, its reaction with carbon atoms results in a large variety of reaction products, compared to the reactions of benzene. Figure 4 shows the molecular structures of the possible reaction products in the singlet state. These products can be divided into four different groups based on the criteria of how the carbon atom is attached to the naphthalene molecule. As can be seen from the figure, the formation of seven-membered carbon rings is energetically the most favorable process. However, the formation of all products is followed by a considerable energy release. For reaction products in the triplet state, the situation is almost similar, with the only exception that the insertion of the carbon atom into the CH bond of the naphthalene molecule is the most favorable reaction path. For each group, we selected one of the energetically most favorable products and calculated the pathway of its formation. The corresponding energy level diagram found in these calculations is given in Figure 5. As can be seen in the figure, the triplet channel of the reaction has a very small energy barrier, and therefore constitutes the most probable pathway of the reaction inside helium droplets. Due to the high stabilization probability for an intermediate reaction product inside the helium droplet (Krasnokutski & Huisken 2014a, 2014c), the formation of a product with a π-bonded carbon atom in the singlet state (relative energy: −223 kJ mol−1) would very likely be the terminal step of the reaction. Also, the formation of a structure with six-, five-, and four-membered rings and the insertion of the carbon atom into the CH bond of naphthalene are associated with energy barriers in the reaction pathway. On the other hand, our calculations predict a very small energy barrier (∼2 kJ mol−1) for the aromatic ring opening and the formation of a seven-membered ring.
Figure 4. Molecular structures of possible products of the C + C10H8 reaction obtained by quantum chemical [b3lyp/6-311+G(d, p)] computations for the singlet state. The relative energies are given in kJ mol−1.
Download figure:
Standard image High-resolution imageFigure 5. Energy level diagram for the C + C10H8 reaction found at the b3lyp/6-311+G(d, p) level of theory. The dashed red line shows the expected pathway of the reaction inside helium droplets. The energies of the triplet levels are given in italics.
Download figure:
Standard image High-resolution imageTherefore, the reactions of carbon atoms with benzene and naphthalene molecules are predicted to proceed very similarly. At first the carbon atom is attached to a carbon atom of the aromatic ring of the molecule. Then, this initial complex undergoes a ring opening with the formation of a seven-membered ring. This structure, which would be an intermediate state in a gas phase reaction, is stabilized by transferring the reaction energy to the surrounding liquid helium. Finally, we can assume that the structure will convert to the energetically most favorable singlet state. This expected pathway is marked in Figure 5 by the dashed red lines. However, it is interesting to note that, in the case of naphthalene, the carbon atom cannot be inserted into the CC bond common for both aromatic rings. Only a weak bonding (117 kJ mol−1) of the carbon atom on top of this bond is predicted.
Our mass spectrometric studies of the reactions of anthracene and coronene reveal very similar results. For all studied molecules, we were able to observe depletion on the mass of the PAH molecule and the appearance of product peaks, which can be ascribed to the addition of a carbon atom to these molecules (see Figure 6). However, there is some difference in the case of the coronene molecule. The peak, which is attributed to the product associated with the loss of a hydrogen atom, is less intense than the parent product peak. For the cases of benzene and naphthalene, it has been demonstrated that the hydrogen loss does not occur in the chemical reaction but happens as a result of the ionization process. Therefore, the observed difference should be attributed to a different type of the reaction product in the case of coronene. However, at present, it cannot be completely excluded that the reduced fragmentation during ionization is simply due to the larger size of the coronene molecule.
Figure 6. Differential mass spectra for the reactions of carbon atoms with anthracene and coronene. The differential mass spectra reveal the effect of carbon incorporation into the helium droplets doped by the PAH molecules.
Download figure:
Standard image High-resolution imageCalorimetric studies of the reactions of the anthracene and coronene molecules with carbon atoms are complicated by the difficulty in precisely adjusting the vapor pressure of the PAH molecules. The present temperature stabilization of the evaporative oven seems to be insufficient. We observed quite large discrepancies for the measured depletion intensities caused by the incorporation of the PAH molecules. Therefore, as in our studies of the C + C10D8 reaction, we performed calorimetric measurements monitoring the depletion of the helium droplet beam, which was caused by the incorporation of carbon atoms. For naphthalene, the application of the same technique was for a different reason, namely the long residence time of the naphthalene molecules on the walls of the pick-up cell.
Figure 7 shows a comparison of the depletion results obtained for the reaction of carbon atoms with anthracene and coronene. Additionally, for the purpose of comparison, the results of the same experiment performed with an inert gas (Ar) instead of the PAHs are given. When the coronene vapor pressure was the same as that used in the earlier experiment for naphthalene (see Figure 3), we observed no difference in the depletion signals caused by the carbon atoms between bare and coronene-doped helium droplets. These measurements are not shown in the figure. Only at larger vapor pressures of coronene (p > 1.5 × 10−5 mbar) were we able to observe an additional depletion signal, which could be attributed to a reaction. However, this additional depletion was rather small, just about a few percent. The upper panel of the same figure shows the results of the calorimetric study for the anthracene + C reaction performed at similar high doping conditions. As can be seen, the additional depletion due to the reaction is much more pronounced in the case of anthracene. At the same time, when argon is used as the second "reactant," the depletion caused by the carbon incorporation does not change after argon doping. From these observations, we can conclude that, in the case of coronene, there is some energy release in the reaction with carbon, but it is much smaller compared to the energy released in the reactions of other PAHs with carbon. This finding is in line with the results of the mass spectrometric study presented previously, which suggests a different type of reaction product in the case of coronene.
Figure 7. Partial pressure of helium in the detector chamber as a function of time as shown by the black solid line. The depletion peaks are caused by doping the helium droplets with carbon atoms. The pressures of the second reactants in the pick-up oven are given by the dashed blue curves. For the purpose of comparison, the lower frame shows the results of the same experiment performed with an inert gas (Ar) instead of the PAHs. The numbers indicate the measured depletion of the helium droplet beam caused by the carbon incorporation.
Download figure:
Standard image High-resolution imageWe applied quantum chemical calculations to study the reactions of carbon atoms with anthracene and coronene. In our computational study, we were mainly interested in the investigation of the pathways found previously for the reaction of carbon with benzene (Krasnokutski & Huisken 2014c) and naphthalene. Hence, we focused on the formation of seven-membered carbon rings. Our computations predict the barrierless reaction of carbon atoms with anthracene molecules and the possibility of a ring opening for all three aromatic cycles, i.e., the formation of seven-membered carbon rings in the center as well as on the sides of the molecule. Thus, the calculations reveal the possibility of the same reaction pathways as observed for benzene and naphthalene. This is in line with the experimental results obtained for benzene, naphthalene, and anthracene molecules. However, similar to the case of naphthalene, the carbon atom cannot be inserted into the CC bond shared by two aromatic rings.
Figure 8 shows the molecular structures of the initial associative complexes between coronene and the carbon atom. It can be seen from this figure that, similar to the cases of the other PAHs studied, the carbon atom lands on the top of the CC bonds. The binding of the carbon atom to the bonds of the central ring is the weakest, and increases when the carbon atom moves to the side of the coronene molecule. Similar to the previously studied PAH molecules, the carbon atom cannot be inserted into the CC bond shared by the two aromatic rings. Therefore, the formation of a seven-membered ring in the center of coronene is not possible. Such a structure was found to be a transition state, with negative binding energy of the carbon atom. The binding of the carbon atom to the coronene molecule was enhanced going from the initial triplet state to the singlet state of the system. The highest binding energy (186.2 kJ mol−1) was found for the carbon atom on top of the peripheral CC bonds in the singlet state. With a relatively small energy barrier, the carbon atom can be inserted into the peripheral CC bonds of the coronene molecule. In this case, the reaction pathway is the same as for the other molecules studied. However, in the case of coronene, there is a considerable increase in the number of CC bonds, which cannot be opened. Therefore, landing of carbon atoms on these sites would not lead to the formation of a seven-membered ring. Two of the four complexes shown in Figure 8, namely complexes 1 and 2, have rather low binding energy. Moreover, the energy barrier for the formation of a seven-membered carbon ring was computed to be a little higher compared to the case of benzene and naphthalene molecules. Therefore, there is a higher chance that the initial associative complexes 3 and 4 could also be frozen inside the liquid helium droplets. This explains the results of the calorimetric measurements showing only low energy released in the reaction of carbon atoms with coronene molecules.
Figure 8. Molecular structures of the initial associative complexes of carbon atom with coronene molecules. The numbers without parentheses show the binding energies of the carbon atom in kJ mol−1.
Download figure:
Standard image High-resolution image5. Astrophysical Implications
Atomic carbon is expected to be involved in the reaction network, which leads to the formation of carbonaceous molecules and finally to carbonaceous grains. If larger molecules are produced due to the addition of small reactive radicals, the abundance of molecules is expected to decrease with increasing molecular size. This is in line with astrophysical observations, which in general demonstrate the decrease of molecular abundance toward larger size (Ohishi et al. 1992). As a result, such a model leads to a large variety of molecules, where the abundance of each specific large molecule is extremely low. Due to the low abundance, such individual molecules are very difficult or even impossible to detect in astrophysical environments. Therefore, the high reactivity of carbon atoms toward small PAH molecules can explain the failure to detect PAHs in the ISM.
However, if any specific product of such a reaction network becomes chemically inert, its abundance would build up over time, probably reaching the level at which it finally can be detected. In other words, the molecule has to be chemically inert to reach a pronounced abundance in astrophysical environments. Obviously, this inertness should be toward reactions with the most abundant species present in the ISM. As discussed in the introduction, the inertness of a hydrocarbon molecule toward the reaction with atomic carbon should be an important criterion for whether such a molecule reaches a noticeable abundance in space.
Our experiments demonstrate the barrierless reaction of carbon atoms with small PAH molecules leading to the formation of seven-membered carbon rings. Therefore, such reactions should be fast in the entire range of low temperatures found in the ISM and are expected to be an effective method of PAH destruction. As is demonstrated by our experiment, the simple addition of carbon atoms to PAHs is expected on the surface of grains. In the gas phase, we also expect a radiative association reaction, if a seven-membered carbon ring is formed for any PAH larger than naphthalene. Therefore, the reactions with carbon atoms increase the molecular size of the PAHs, leading to the formation of nonclassical PAH molecules. On the other hand, further reactions with hydrogen or UV irradiation could result in the formation of classical PAH molecules with larger molecular size. The balance between these two processes should depend on the specific conditions and is difficult to predict. Therefore, the reactions with carbon atoms may lead to the presence of a substantial portion of nonclassical small PAHs in astrophysical environments.
With increasing molecular size, PAHs become more inert toward reaction with carbon atoms. As was found in our studies, the formation of a seven-membered ring is only possible if the carbon atom initially lands on a peripheral CC bond of the PAH molecule. With increasing PAH size, the portion of peripheral CC bonds is decreasing. The landing of the carbon atom in the center of the PAH molecule only leads to a weak bonding of the carbon atom on top of the ring. The energy of such a bond is below 100 kJ mol−1, which is much weaker than any chemical bond in the PAH molecules. Therefore, the collision of a carbon atom with the center of a PAH molecule may not even lead to the formation of a complex, due to the poor coupling between the C−PAH stretching vibration and the vibrations of the PAH molecule (Omont 1986). In the case of a complex formation, it can be very efficiently dissociated upon UV photon absorption, giving back the same PAH, so that its concentration will not be affected. Therefore, with increasing molecular size, we expect a larger portion of classical PAH molecules compared to nonclassical ones. Moreover, the reaction with carbon atoms favors the formation of the pericondensed PAH molecules over the catacondensed ones, and the more circular compact geometries over the elongated structures. It is interesting to note that these conclusions are in line with the photostabilities of the PAH molecules (Jochims et al. 1999). However, the selection of PAHs based on their photostability should be less important for larger molecular sizes. This is due to the fact that large PAHs, even with an unfavorable structural arrangement, are expected to be stable against UV irradiation. At the same time, the reactions with carbon atoms would still favor the formation of compact structures even for PAHs containing more than 50 carbon atoms. However, in spite of some selection rules, which may result from reactions with carbon atoms, a large variety of compact PAH molecules is still expected to be present in the ISM.
A quantitative estimation of the reactions of carbon with PAH molecules was performed by Allain et al. (1996a). This study addressed the competition between photodestruction and growth of PAH molecules. The PAHs growth time was given by
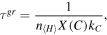
where , X(C), and kC represented the total hydrogen density, the carbon abundance, and the reaction rate for the accretion of carbon atoms or cations, respectively. For X (C) and
, values of 3 × 10−4 and 10−12 cm3 s−1 were taken. However, the value for the reaction rate was adopted from Omont (1986), who assumed that the carbon atom should land on the chemically active site of the PAH molecule, which was produced, for example, by photodesorption of a hydrogen atom. It was noted that the actual rate should be much higher if the landing on any position would cause the reaction. Our results show that C + PAH reactions are fast even without active PAH sites as the carbon atoms land on the top of the PAHs and cause an opening of an aromatic ring, which is followed by the formation of a seven-membered carbon ring. As such reactions are found to be barrierless, the rate of such a reaction should be mainly defined by the collision rate, and it seems reasonable to use a value of 5 × 10−10 cm3 s−1 for the reaction of small PAHs with carbon atoms. We recalculated the time necessary for an addition of a single carbon atom to a PAH molecule in the following common astrophysical environments considered by Allain et al. (1996a): planetary nebula NGC 7027 (2 year), H ii region Orion Bar (3 year), reflection nebula NGC 7023 (52 year), Diffuse Medium Galactic plane (3.5 × 105 year). It should be noted that, in the denser regions of the ISM, the abundance of carbon atoms and cations is expected to decrease with time due to the oxidation of carbon (C+ → C → CO). Thus, it could be lower than 3 × 10−4 as assumed by Allain et al. (1996a). However, the denser areas are also characterized by lower photon fluxes. Therefore, the high reaction rate of carbon atoms makes the growth of PAHs more competitive with their photodestruction. Additionaly, taking into account the fact that even small PAHs with favorable structural arrangement were predicted to survive the absorption of UV photons in H i regions (Jochims et al. 1999), we conclude that the formation of PAHs in the ISM could occur through sequences of bottom-up reactions involving carbon and hydrogen atoms.
6. Conclusion
The barrierless reaction of small PAH molecules with carbon atoms was observed in our experiment. The carbon atoms land on top of the CC bonds of the PAH molecules. The formation of this initial associative complex is followed by a ring opening and the formation of a seven-membered ring. This reaction is expected to destroy PAH molecules in the ISM. At the same time, the reactivity with carbon atoms leads to a growth of the molecular size of the PAHs. Due to the fact that such a reaction pathway is only applicable for carbon atoms landing on top of peripheral CC bonds, larger PAHs are expected to be more inert toward the reaction with carbon atoms. Moreover, these reactions should favor the formation of compact pericondensed PAH molecules having fewer peripheral bonds. The barrierless C + PAHs reactions suggest that the formation and growth of PAHs could occur through sequences of bottom-up reactions even in the low-temperature areas of the ISM.
The authors are grateful for the support by the Max Planck Institute for Astronomy (MPIA) and the Deutsche Forschungsgemeinschaft DFG (Contract No. KR 3995/3-1).