Abstract
Nanostructured diffusion-limited-aggregation (DLA) crystal pattern formation in microemulsion consisting of water, styrene, cetyltrimethylammonium chloride (CTACl), potassium persulphate and an oscillating Belousov–Zhabotinsky (BZ) reactant is reported. A variety of spatiotemporal patterns like concentric wave, spatial (stripe) and chaotic patterns appear. A colloidal phase composed of numerous nano-sized particles has been observed. The solid phase nucleation has been found to occur in the colloidal phase and has been found to grow in a symmetric crystal pattern with the progress of the reaction finally exhibiting DLA structures. We show that the formation of a nanostructured DLA crystal pattern is governed by spatial structures emerging in the BZ microemulsion system. Without any spatial structure in the microemulsion system only hydrogel of high viscosity is formed. A nano-sized branched crystal pattern was formed with a particle diameter in the range of 60–100 nm, as evident by transmission electron microscope, powder x-ray diffraction and particle size analyser studies.
Export citation and abstract BibTeX RIS

Content from this work may be used under the terms of the Creative Commons Attribution 3.0 licence. Any further distribution of this work must maintain attribution to the author(s) and the title of the work, journal citation and DOI.
1. Introduction
Pattern formation in nature constitutes a crucial problem for understanding life in general. In fact, several mechanisms have been proposed aiming to understand wave propagation in such a system as well as stationary patterns. Examples of these behaviours are almost ubiquitous in nature [1], intracellular media [2], waves in heart tissue [3], catalytic surface reactions [4], the retina, social amoeba [5] or Belousov–Zhabotinsky (BZ) reactions [6]. The Turing mechanism [7] is believed to explain the problem of symmetry breaking in biological morphogenesis underlying cell differentiation in the first days of development of early embryos [8] or the pigmentation of the skin of animals [9].
The development of meso- or nanomaterials with special size and well-defined shape may open new opportunities for exploring the physical and chemical properties of materials [10]. Among the different nanostructures, diffusion-limited-aggregation (DLA) crystal growth patterns are attracting the attention of many scientists due to their importance connected to some fractal growth phenomenon and crystallography research, and because they have wide applications in micro- and nanodevices. The synthesis of nanostructured material under far-from-equilibrium conditions has emerged as an important new concept in the design of materials [11]. An oscillating chemical reaction is the most appropriate active media for the study of such a synthesis. As a BZ reaction, the catalytic periodic oxidation of malonic acids (MA) by bromate in acidic aqueous solution is the prototype nonlinear reaction for studying chemical oscillations in a stirred tank reactor and waves in spatially extended systems gels [12], membranes [13], mesoporous glasses [14] and ion-exchange resins [15]. The first experimental observations and theoretical analysis of pattern formation in the BZ system dispersed in a water-in-oil aerosol bis(2-ethyhexyl)sulphosuccinate (AOT) microemulsion (BZ-AOT system) was reported by the Epstein group [16, 17]. The BZ reaction dispersed in water-in-oil aerosol AOT microemulsion's (BZ-AOT) system has been shown to be an excellent system for studying pattern formation in reaction–diffusion systems, including Turing patterns [16], oscillations [18] and new wave patterns such as packet waves and anti-spirals [19] dash waves, and segmented spirals, however the DLA pattern formation has not been reported in the microemulsion system to understand the mechanism of biological pattern formation.
Recently, there has been increasing interest in fractal growth phenomenon under non-equilibriums conditions [20, 21]. Laplacian growth phenomenon in pattern formation has attracted considerable attention [22]. The Laplacian growth model is consistent with the DLA model [23]. DLA methods have been used to describe the growth behaviour and fractal complexity during pattern formation in a BZ-type reaction system [24, 25]. This method involves a very simple algorithm which can produce highly intricate structures by random aggregation of particles. DLA-like patterns have also been observed in various crystallization phenomena usually at far equilibrium conditions, such as electro-deposition, bacterial colonies, colloidal aggregates, dendrite formation, viscous fingering and many others [26]. Dendrimers, so-called 'cascade molecules', have already found use as drug candidates for receptor–ligand interactions, drug carriers for conferring bio-survival, membrane permeability and targeting, and have found wide use as carriers for vaccine antigens as well. Furthermore, dendrimers have proven very useful as scaffolds in the design of biosensors, imprinting scaffolds and artificial receptors. This will add a new degree of sophistication to well-known drugs and regents, as well as creating entirely new classes of drugs and bioactive substances based on these macromolecular, yet beautifully simple structures. Dendritic structures are, despite their large molecular size, structurally well-defined, with a low polydispersity in comparison with traditional polymers. Thus, the DLA methods have been widely used to explain and analyse the fractal aggregation phenomenon. The size, shape and structure can be controlled by various experimental parameters such as particle flux, substrate concentration and temperature. The dependence of morphology on initial conditions helps to understand the fundamental growth mechanism involved in pattern formation and finally control over-organized growth. It is interesting to investigate how well defined pattern can be selectively grown, tuned and modified through control of the initial condition and temperature in BZ systems. Chemical systems offer the advantage that one can often control the parameters that determine the patterns formed and can thereby probe fundamental issues about pattern formation, with possible insights into biologically relevant phenomena. In some systems, the pattern that emerges depends not only on the chemical and physical parameters, but also on the initial state of the system. Our research interests are to elucidate the mechanistic aspects of aggregation in macromolecules related to the biological pattern formation. It is well known that uncontrolled aggregation of macromolecules leads to serious neuro-related diseases such as Alzheimer's, Creutzfeldt–Jakob's and Parkinson's disease. Thus, understanding the aggregation mechanism of macromolecules is of paramount importance since research in this area provides insightful knowledge towards controlling the aggregation propensity of macromolecules.
The growth of nanostructured dendritic crystals is also a profound example among a wide range of pattern-forming phenomena in nature and biology. In the present work our aim is to use a chemical system with the ability to self-organize to control nanostructures, pointing out the fact that the discrete nature of these systems plays a crucial role in the pattern formation mechanism which is relevant to the pattern formation in complex biological processes occurring in nature. The BZ reaction is one of the best prototype systems for such self-organization phenomenon. In this connection, the growth of nanostructured DLA crystal patterns in this modified chemical system comes under a new study towards crystal growth associated with self-assembly and BZ reaction processes. The formation of nanostructured DLA crystal pattern in reactive microemulsion systems by using styrene as a monomer and cetyltrimethylammonium chloride (CTACl) as cationic surfactant has been achieved. The surfactant plays a key role in tailoring the nanostructured DLA pattern. The colloidal phase of the reaction is composed of numerous fine solid particles (nano size) which are found to form some solid phase nucleation centres of dendritic character. Consequently, these nucleation centres were found to grow into DLA-like patterns which attribute, by self-organization. An attempt has been made to propose the possible reaction mechanism for the growth of DLA crystal patterns.
2. Experimental details
2.1. Materials
Malonic acid (MA) (Sigma), iron (II) sulphate (CDH), 1,10-phenanthroline, styrene (E-merck), cetyl trimethyl ammonium chloride (CTACl) (Aldrich), potassium bromate (KBrO3), potassium persulphate (K2S2O8) (Sigma-Aldrich) and sulphuric acid (H2SO4) (S.D. fine chemicals) of AR grade were used for the experimentation. All the experiments were performed in a flat Petri dish of 9.1 cm (i.d.). Stock solutions of MA (10% w/v) and ferroin ([Fe(phen)3]2+ = 0.025 mol dm−3) were prepared in doubly distilled water (DDW). Stock solution of the KBrO3 prepared in H2SO4 = 1.5 mol dm−3. The ferroin indicator used was prepared by homogeneous mixing of 1,10-phenanthroline and iron(II) sulphate in a molar ratio of 3:1 in DDW. The stock solution of K2S2O8 (PS) (0.036 99 M) was also prepared in DDW. The stock solution of styrene (8.72 M) and CTACl (0.7812 M) was taken directly from a packed bottle. In the present experiment, aqueous solution of CTACl and 99.9% pure styrene monomer were available in the laboratory, which was taken into account during experiment.
In the experiment, K2S2O8 solution (10 ml) was taken in a beaker and heated to 60 °C under stirring. After that, 10 ml of CTACl was added into the solution, followed by the addition of 2 ml styrene. The mixture was continuously stirred at 500 rpm for 5–10 min, resulting in the formation of milky thick solution, which was cooled down and washed with methanol to separate the unreacted styrene monomer in the mixture, by using separating funnel. This solution has been denoted as B. Two stock microemulsions, ME1 and ME2, were then prepared, one containing sulphuric acid (2 ml), malonic acid (MA) (4 ml) and B solution (1.5 ml), and the other containing ferroin (2 ml), potassium bromate (4 ml) and B solution (1.5 ml). The partitioning of the reactants between two stock microemulsions ensured that the BZ reaction did not begin until they were mixed together. Half of the ME1 and ME2 solutions were mixed to the resultant mixture and were well shaken to maintain homogeneity. This stage was marked as initiation of the reaction. Thereafter, reaction mixture (6.3 ml) was immediately taken out from the bulk solution and spread in a Petri dish with uniform thickness (≈ 1 mm). The resultant concentrations of reactants were calculated as: MA (0.5124 mol dm−3), KBrO3 (0.0553 mol dm−3), styrene (1.162 mol dm−3), [Fe(phen)3]2+ (0.0038 mol dm−3), H2SO4 (0.42 mol dm−3), CTACl (0.1562 mol dm−3) and K2S2O8 (0.4932 mol dm−3).
2.2. Characterization
The morphological study and nanostructure of the DLA branched pattern was carried out by transmission electron microscope (TEM) with model Morgani (FEI) Netherland 268 D, operated on accelerating voltage of 60–80 kV at 50 nm resolution, scanning electron microscope (SEM) (Jeol, JSM 6390LV) coupled with energy dispersive spectroscopy (EDS) under vacuum, at an accelerating voltage of 20 kV and by optical microscope (OPM) (Leica, EZ4D) at a specific magnification. The crystal pattern was characterized by x-ray diffraction technology on a D/Max-3C equipment of Rigaku denki Co. Ltd by using CuKα radiation and a fixed powder source (30 kV, 15 mA) were used; the applied scan rate was 2° (2θ) min−1. The 1H NMR spectra were recorded on 400 MHz. JEOL FX90Q FTNMR spectrometer using tetramethysilane (TMS) as an internal standard in dimethyl sulphoxide (DMSO-d6). The average particle size of the DLA crystals' pattern was determined by the dynamic light scattering (DLS) technique (MALVERN; DTS Ver.4.10; serial number MAL500999). The digital photograph of the chemical wave pattern and growth stages of the crystal pattern were monitored with a digital video camera (Nikon) equipped with 5 × optical zoom connected with a PC.
3. Results and discussion
The present BZ microemulsion reaction system consists of some complex reactions, which take place in multi steps with proper sequences, resulting in the formation of the DLA pattern. The study has been carried out in the following section.
3.1. Development of the spatiotemporal pattern in emulsion media
A microemulsion is a thermodynamically stable mixture of water, oil and a surfactant molecule. The surfactant molecule provides a stabilizing interface between two other immiscible phases. It is the thermodynamic stability of microemulsions that distinguishes them from a normal emulsion; mixtures of oil and water are possible (an emulsion), however, such mixtures are unstable and will gradually separate into two phases. Microemulsions, on the other hand, represent an energetically favourable configuration of the water, oil and surfactant constituents.
The water-in-oil bis(2-ethylhexyl) sulphosuccinate (AOT) microemulsion is one of the most-characterized microemulsion systems [18, 19]. Usually, the surfactant molecules form microemulsion with non-polar solvents. Keeping this concept in mind, we have selected styrene/CTACl/water microemulsion system in the present work. The CTACl belongs to a family of molecules known as cationic surfactants, which can be used as emulsifying agents. The water-in-oil micelles are clusters of molecules where the polar groups are in the interior portion of the micelle and the oil soluble portions are on the exterior of the micelle. Figure 1 represents the structure of CTACl molecule and micelles in aqueous solution, respectively.
Figure 1. Structure of CTACl molecule (a) and formation of micelles (b).
Download figure:
Standard image High-resolution imageAt first, the desired quantity of the reaction mixture is poured into the Petri dish and a series of different spatiotemporal patterns were obtained in the reaction–diffusion system (figure 2). The first pattern, which is a concentric ring-type wave, was obtained after 20 s (figure 2(a)). The homogeneous red colour of the first pattern suddenly turned into blue with the formation of the second spatial patterns (figures 2(b) and (c)). This phenomenon completed within 15 s. After a short steady state of 2 min the blue colour turned into light red with the formation of the third pattern (chaotic type pattern figure 2(d)) which is relevant to the chaos observed in a chemical system [27]. At a certain phase of the development of the pattern, light pacemarker centres appear in the mosaic part of the pattern from which chemical waves start to propagate. After its emergence, the pattern remains stationary for a time of the order of 70 min before it gradually fades and then after giving two to three oscillations, solutions turn to blue, where the system stays for few seconds. Finally it settled down in the form of colloidal material spread throughout the Petri dish where the system stays for 6 h and then bifurcate to heterogeneous solution for several hours and finally achieving a DLA crystal pattern (figure 2(e)). This DLA pattern is stable for several days.
Figure 2. Images showing the concentric ring (a), spatial pattern (b) and (c) chaotic pattern (d) and corresponding DLA crystal pattern formed in the microemulsion system (e) microphotograph of the DLA pattern formed in figure (e), (f) composition MA (0.5124 mol dm−3), KBrO3 (0.0553 mol dm−3), styrene (1.162 mol dm−3), [Fe(phen)3]2+ (0.0038 mol dm−3), H2SO4 (0.42 mol dm−3), CTACl (0.1562 mol dm−3) and K2S2O8 (0.4932 mol dm−3).
Download figure:
Standard image High-resolution imageFollowing the idea to find conditions where the BZ system exhibits a spatial pattern and adding a monomer to the system leads to DLA pattern formation. In particular, we have studied the polymerization of styrene (scheme 1). If only styrene is added to the BZ system, only hydrogel of high viscosity is formed at all concentrations of the BZ system we have studied. To accelerate the polymerization process we added the polymerization initiator potassium persulphate (PS).
Scheme 1. The polymerization of styrene (a) and the bromination of polystyrene (b)–(d).
Download figure:
Standard image High-resolution imageIncreasing the concentration of the surfactant has resulted in a more stable DLA crystal pattern formation. On the other hand, increasing the concentration of sulphuric acid supports the formation of the spatial wave pattern in the BZ system but simultaneously inhibits the polymerization processes. To achieve the desired formation of a nanostructured DLA crystal pattern, it is necessary to change initial concentrations of both, bromate and surfactant. For certain concentrations of styrene and K2S2O8, the stationary patterns caused by hydrodynamic instability [28] are observed after adding the later solutions to the BZ reaction mixture. An increase of the initial surfactant concentration supports the formation of these hydrodynamic structures. Finally we found that polymerization occurs and a nanostructured DLA crystal pattern is formed in the BZ system for the following fixed initial concentrations of reagents: MA (0.5124 mol dm−3), KBrO3 (0.0553 mol dm−3), styrene (1.162 mol dm−3), [Fe(phen)3]2+ (0.0038 mol dm−3), H2SO4 (0.42 mol dm−3) and CTACl (0.1562 mol dm−3) and K2S2O8 (0.4932 mol dm−3).
Figure 3(a) has confirmed that there is no DLA crystal formation and only hydrogel of high viscosity is formed in the microemulsion system under conditions where no spatial self-organization phenomena have been observed. In contrast, if BZ spatial patterns appear in the BZ reaction, the crystal pattern obtained is characterized by the nanostructured DLA crystal pattern. Figure 3(b) shows a typical picture of a nanostructured growth DLA crystal pattern at low concentration of styrene. A comparison of the images presented in figures 3(a) and (b) illustrates the difference between the spatially non-formation and formation of the DLA crystal pattern.
Figure 3. Images showing spatially non-formation (a) and formation (b) of DLA crystal patterns under different conditions. Initial concentration of variable components: (a) 0.0490 M KBrO3 and 0.9889 CTACl (b) 0.0553 M KBrO3 and 0.1562 M CTACl. Initial concentration of other components MA (0.5124 M), styrene (1.162 M), [Fe(phen)3]2+ (0.0038 M), H2SO4 (0.42 M) and K2S2O8 (0.4932 mol dm−3).
Download figure:
Standard image High-resolution imageFigure 2 illustrates the relation between spatial structures in the BZ system and nanostructured growth DLA spatial crystal patterns. Figures 2(a)–(d) display spatiotemporal BZ patterns, i.e. concentric BZ wave, spatial pattern and chaotic pattern observed in the BZ system at the particular moment of time that may correspond to the duel frequency oscillations in the BZ system [29, 30]. This moment of time lies between 4 and 8 min after mixing the reaction components. Figure 2(e) presents images of fully grown nanostructured DLA crystal patterns obtained for conditions corresponding to those in figures 2(a)–(d). For the conditions corresponding to figure 3 it is obvious that the structure of DLA crystal patterns mimics the stationary pattern observed in the BZ system. Our experiments have shown that an increase in the initial concentrations of styrene and MA leads to the appearance of DLA ordered crystal structure. These structures are governed by BZ patterns, i.e. concentric BZ wave, spatial and chaotic patterns formed in the BZ system. The formation of several types of stationary patterns, such as stripe, labyrinth, hexagonal, rhombic and black eye, in quasi-two-dimensional reaction–diffusion systems has been reported [31]. Here in the liquid phase, various types of BZ patterns as shown in figures 2(a)–(d), were generated in the reaction–diffusion system. Varying the initial concentration of MA indicates a critical minimal concentration of 0.040 45 M of MA below which a DLA crystal pattern does not form. Varying the initial concentrations of bromate ions led us to the conclusion that a DLA structured crystal pattern may be obtained only if spatial BZ patterns appear in the BZ system. Below a critical initial concentration of bromate ions of 0.0748 M there are no spatial structures in the BZ system and a non-formation of the DLA crystal pattern is formed.
The experimental data reported here may be supported by the kinetic scheme 1 and table 1.
Table 1. The reaction occurred in the BZ system.
Reaction | Number |
---|---|
H++HBrO2+HBrO3↔HBrO2++BrO•2+H2O | (1) |
BrO•2+H+↔HBrO2+ | (2) |
Fe(phen)32++HBrO2+↔Fe(phen)33++HBrO2 | (3) |
2 HBrO2↔HOBr+HBrO3 | (4) |
H++Br−↔HBrO2+2 HOBr | (5) |
H++Br−+HOBr↔Br2+H2O | (6) |
H++Br−+HBrO3↔HBrO2+HOBr | (7) |
Fe(phen)33++CHBr(COOH)2↔Fe(phen)32++H++•CBr(COOH)2 | (8) |
H2O+•CBr(COOH)2↔H++Br−+•COH(COOH)2 | (9) |
HOBr+CHBr(COOH)2↔CBr2(COOH)2+H2O | (10) |
Br2+CHBr(COOH)2↔CBr2(COOH)2+H++Br− | (11) |
CHBr(COOH)2+H2O↔CHOH(COOH)2+H++Br− | (12) |
In scheme 1(a), the polymerization of styrene has been presented, which was initiated by the peroxysulphate ions generated from the reaction system. This polymerization reaction gets stable with the addition of the CTACl surfactant [32]. The polymerization reaction occurs before we added this mixture to the BZ microemulsion system. Table 1 shows the reaction occurred in the BZ system, catalyzed by ferroin coupled to the bromination of polystyrene. All these reaction steps are related entirely to the BZ chemistry [33]. In the ferroin catalyzed BZ system, when these reactions coupled with diffusion, resulting in the development of various spatial self-organization phenomena, viz. waves, target patterns, spirals, twinkling patterns and Turing patterns in microemulsion systems [18, 19]. It is clear from the reaction scheme that the free radical itself has been generated from the BZ system according to reactions (8) and (9). These free radicals initiated the bromination of polystyrene, presented in schemes 1(b)–(d). A sufficient source of radicals can be provided through these reactions. These radicals actually initiate the H-abstraction from polystyrene molecule, and resulting in the attachment of bromine in that position, formed brominated polystyrene. As we have separated the styrene monomer before the mixture got introduced to the BZ system, therefore bromination of the polystyrene molecule is the most possible final step of the reaction series happened in the BZ microemulsion system which was catalyzed by the iron through a ferroin indicator.
3.2. Growth phenomenon of nanostructured DLA crystal pattern formation
A colloidal phase composed of numerous fine solid particles (nano-sized) suspended in the solution matrix has been observed after 6 h. These colloidal particles have started self-aggregation, and exhibit solid phase nucleation centres of dendritic character, as shown in figure 4. The nuclei centres formed at an early stage were found to dominate over nuclei centres at the later stage, and exclusively took part in the growth process.
Figure 4. A schematic representation of the proposed growth mechanism.
Download figure:
Standard image High-resolution imageAfter 4 h from the beginning of the reaction process, the solid-phase nucleation phenomenon started occurring. Initially, the system exhibits numerous fine solid particles settled down in the matrix of reaction solution, called the colloidal state. These particles always play an important role in the solid-phase nucleation and subsequently in the growth of crystal patterns. The solid-phase nucleation that occurs during the colloidal state of the reaction initially emerges in the form of certain discrete points called nucleus centres, which are possibly formed by random aggregation of colloidal particles. It has also been observed that fine solid particles are distributed around the dendritic nucleation centre. Thus a concentration gradient between nucleation centres and symmetrically distributed fine solid particles cannot be ignored. Under such conditions, an organized nucleus structure can be produced through a diffusion mediated aggregation process. In early phase, this structure has a large surface of curvature and might be composed of mesoscopic crystals, which attributes high surface energy. Therefore, further growth can lead to the formation of a stable and specified geometric crystal pattern which was essentially monitored by lowering the surface energies.
The formation of ordered dendritic crystal structure can be favoured by two factors relating to conventional BZ reaction systems [34]. Firstly, the dynamic instability generates a concentration gradient between nucleation centres and fine solid particles. The concentration gradient facilitates colloidal particles to fuse into the solid interfaces in a synchronized manner. The effective transport properties of the particles depend upon the diffusion coefficients that can allow particles to fuse either with higher or lower mobility. It has also been assumed that the dynamic instabilities could also dictate the orientated aggregation of fine solid particles during formation of dendritic nucleus structure. Secondly, surface anisotropy determines the randomness in the resulting structure. This can be induced by non-uniform surfaces of the metal catalysts and also due to probable multi crystal phases. In this circumstance, a DLA mechanism could facilitate coherent side branching in the dendritic crystal patterns. In addition to this the metal catalysts (Fe2+/Fe3+) present in the reaction system participate in wave and pattern formation, during solution phase reaction. Due to their non-uniform surfaces, they can provide long-lived dynamic heterogeneities to the reaction system [35]. This heterogeneity can build up a transportation gradient between nucleation sites and the symmetrically distributed colloidal particles. As expected, the fine solid particles are more than a single type which differs in diffusion coefficients. Depending upon characteristics and size of these particles, the diffusion coefficients allow aggregation with much higher or lower mobility towards the nucleation sites. Under diffusion conditions, this aggregation mechanism is similar to cycles of oscillations or periodic routes [34]. It results in a fine-tuned ordered structure of dendritic patterns, as observed during the experiment.
In recent times, there has been a renewed interest in understanding the growth processes of solid phase within another solid in the nanometric regime. Interestingly, the kinetics of the growth of a solid from a solution, which is the most popular chemical method to produce a wide variety of systems with dimensions in the nanometre region, has been relatively less investigated [36]. However, there is a popular belief that the growth in solution occurs via a diffusion limited Ostwald ripening process [37].
During colloidal-phase reaction, the dendritic nucleus centre has been found to grow in specified geometry, which results in DLA branched crystal patterns. The resulting morphology is shown in figures 5(a) and (b) confirms the apparent growth behaviour and branching mode of DLA patterns.
Figure 5. Growth of DLA-like branched crystal pattern (a) after 8 h and (b) 10 h.
Download figure:
Standard image High-resolution imageThe DLA branched patterns exhibit a coherent side branching similar to the symmetric dendrites. The morphology of the studied DLA patterns closely resemble some simulated DLA-like patterns [26]. Similar to the DLA model, the growth process begins from prerequisite nucleation sites. The solvent acts as a medium which allows the fine solid particles to aggregate towards the nucleation site by random walks. Nucleation occurs over some time with constant monomer (styrene) concentration. Eventually, surface growth of the cluster begins to occur which depletes the monomer (styrene) supply. Here nucleation does not further occur, when the monomer concentration falls below the critical level for nucleation. A general analysis of the growth processes is then important to understand nanocrystal synthesis. In general, the surface to volume ratio in smaller particles is quite high. As a result of the large surface area present, it is observed that surface excess energy becomes more important in very small particles, constituting a non-negligible percentage of the total energy. Hence for a solution that is initially not in thermodynamic equilibrium, a mechanism that allows the formation of larger particles at the cost of smaller particles reduces the surface energy and hence and plays a key role in the growth of nanocrystals. A colloidal particle grows by a sequence of monomer (styrene) diffusion towards the surface followed by reaction of the monomers at the surface of the nanocrystals. The diffusion process is dominated by the surface energy of the nanoparticles. The interfacial energy is the energy associated with an interface due to differences between the chemical potential of atoms in an interfacial region and atoms in neighbouring bulk phases. For a solid species present at a solid/liquid interface, the chemical potential of a particle increases with decreasing particle size, the equilibrium solute concentration for a small particle is much higher than for a large particle. The equilibrium concentration of the nano-sized particle in the liquid phase is dependent on the local curvature of the solid phase. Difference in the local equilibrium concentrations, due to the variations in curvature set up concentration gradients and provide the driving force for the growth of larger particles at the expense of smaller particles [38]. The possible nucleus centres have been found to exhibit formation of akin crystal patterns. The possibility of fine solid particles to enter most exterior segments of the crystal surface are least, subsequently, the nucleation centres formed at a later stage can start to grow at a moderate rate while the growth rate of the nucleation centres formed in earlier stages become slow due to the screening effect similar to the DLA experiment. Colloidal particles aggregate during the growth of the DLA crystal patterns. These colloidal particles have been regarded as growth units which participated in the growth of DLA crystal patterns.
The average radius of the particles is assumed as r. The bulk liquid phase is considered to have a uniform supersaturated monomer concentration, cb, while the monomer concentration at the particle interface, ci and the solubility of the particle with a radius r is presented by cr. The flux of monomer, J, passing through a spherical surface with radius x within the diffusion layer, is given by Fick's first law [39] as
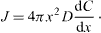
At steady state, where J is constant over the diffusion layer x. After integration, the above equation can be written as
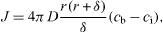
where δ is the thickness of the diffusion layer.
The DLA is a particular model of a random irreversible growth. The growth process starts from seed particles. A second particle attaches far from the seed by random walk. It sticks and forms a two-particle cluster extending the initial seed. By repeating the processes one observes the growth of a random object which has an open structure. The fractal dimension of an open pattern was found as 1.70 in agreement with the DLA model. The presence of cationic surfactant CTACl to the system leads to the formation of compact structures. The typical morphology of the DLA has been found to resemble polymeric spherulites [40]. It has also been observed that the DLA pattern grows radially from their nucleation site, branching intermittently to maintain a space-filling character. The size of the fully grown DLA crystal pattern is in the range 2–3 cm (diameter), much larger than most of the studied polymers or others spherulites. The observed DLA patterns were stable indefinitely at room temperature if protected from moisture. At high humidity, the DLA deliquesced in 2–3 days. The DLA crystal patterns are stable on heating up to 90 °C. The crystal materials of DLA crystal patterns are frequently soluble in polar solvents such as RCOOH, ROH, RCOOEt, etc.
3.3. Morphological study of nanostructured DLA crystal patterns
The branching orders of DLA branched crystal patterns were considerably characterized with the help of OPM, SEM and TEM. The crystal pattern was composed of large numbers of well-aligned crystals as branches. The branches associated with a common point (nucleus centre), correspond to the primary branches which form the initial outline of the DLA branched crystal patterns. Secondary and tertiary branches are in turn associated with primary branches which ultimately results in densely branched crystal patterns. Due to diffusion field restrictions, all three kinds of branches, as observed, do not intersect with each other. It implies that the aggregation process has not been completely random. The microphotograph of the colloidal phase particle and DLA branched crystal pattern are shown in figure 6.
Figure 6. OPM photograph of the growth of the colloidal phase particle (a), (b) and nanostructured DLA branched crystal pattern (c), (d).
Download figure:
Standard image High-resolution imageThe microphotograph of the DLA branched pattern shows several nodes and branching in the form of long thin wires bunched together as appear in the structure. The DLA branched pattern exhibits a highly branched morphology. There is a slight correlation among the side-branched positions on either side. The regularity in the structures is reminiscent with regular dendritic growth subject to periodic external perturbations [41]. The side branching was found as broad or expanded growing tips. These growing tips intermittingly become coarse by newly formed branches normally predominant over others as the reaction time progresses.
The branching patterns and crystal character of the DLA-like branched crystal pattern are examined by the SEM technique. The SEM images and EDS spectra of the DLA-like branched pattern are shown in figure 7.
Figure 7. SEM images of nanostructure crystalline particles (a), (b); SEM images of DLA crystal patterns (c), (d) and EDS spectra of DLA crystal patterns.
Download figure:
Standard image High-resolution imageIn figures 7(a) and (b), the SEM images of the nanostructure particle are presented. From the SEM images it was found that the shapes of some of the nanostructured particles are cubic, while others are hexagonal. The result emphasizes that the main architecture of both types of DLA-like branched patterns were composed with fibre-like microcrystals (figures 7(c) and (d)). The large number of long fibrils has been involved in forming a densely branched organization of the DLA pattern. They are associated with notches and some joints as seen in the microphotograph. The self-organization among the crystallizing units can be carried out through the mentioned fabrications [41], as shown in figures 7(c) and (d). The EDS spectra of nanostructured DLA crystal patterns show the presence of bromine and iron (figure 7(e)). The DLA branched crystal patterns were further characterized by TEM (figure 8(a)) and figure 8(b) shows the areas for the electron diffraction pattern of the resultant DLA crystal pattern. The TEM studied revealed the formation of nano-sized particles with particle diameters in the range of 20–100 nm. The selected area diffraction (SEAD) pattern is taken from the branch and trunk. We observed three main fringe patterns with their radii in the ratio of 0.25:1.80, which are close to the interplanner spacing values for DLA crystalline particles to the (220) and (311) planes.
Figure 8. TEM images of the nanostructured DLA crystalline particles (a). Inset: SAED pattern of the DLA branched crystal pattern (b).
Download figure:
Standard image High-resolution image3.4. X-ray analysis
The powered x-ray diffraction (XRD) spectra of nanostructure DLA branched crystal patterns are shown in figure 9. Evidence of a sharp peak in the region of high intensities suggested that the DLA structure must be composed of a majority of crystalline matter. Some broadened peaks were observed in the region of low intensities that emphasize that the certain amorphous mass has also been trapped in the structure of the DLA crystal pattern. The amorphous phase may serve as binding units of multi crystal phases in a specific frame during the growth of the nanostructured DLA pattern.
Figure 9. XRD graph of the DLA-like branched crystal pattern.
Download figure:
Standard image High-resolution imageThe particle diameter D was calculated by XRD studies using the Debye–Scherrer equations [42]

where β is the half-maximum width, λ is the wavelength and θβ is the Bragg's angle corresponding to the β value. The particle diameter was found to be 35 nm, in agreement with TEM result.
3.5. Particle size analysis
The average particle size analyses of DLA crystal patterns formed in microemulsion system were carried out by the DLS technique, as shown in figure 10. The average particle size has found to be in the range of 50–80 nm, as is evident by TEM analysis. It further supports the self-organization during the growth of DLA pattern formation in the system. The particles of nanometre range can be self-organized by a short range of intermolecular attraction, which may favour the formation of a fibrous network and also a highly ordered crystal pattern.
Figure 10. Particle size analyzer spectra of the nanostructured DLA crystal pattern.
Download figure:
Standard image High-resolution image3.6. Structural determination
The structural determination of DLA crystal pattern formation which is the composition of bromo-polystyrene was confirmed by 1H NMR. The spectral data were obtained as follows:
1H NMR (CDCl3, 300 MHz): δ (ppm) = 7.36–6.38 (br m, arom. H), 4.48 (br m, CH2–CH(Ph)–Br, 2.17–1.24(br m, backbone CH2, CH), 1.11–1.02 (br s, H3C–CH(Ph)–CH2).
4. Conclusion
Diffusion-limited reactions are commonly found in biochemical processes such as enzyme catalysis, colloid and protein aggregation and binding between different macromolecules in cells. Dendrimers are polymeric substances that recently achieved importance in biochemistry as they are used as targeted vehicles for drug delivery in living organisms. Many of the potential application systems are also important from the pattern formation point of view. We have demonstrated throughout this paper that DLA patterns play an important role in pattern formation mechanisms. The TEM, XRD and particle size analyser graph shows that crystalline particle size is in the range of 20–100 nm. The fibrillar organization of DLA was also supported by the SEM result. The system of colloidal particles formed during reaction plays a significant role for solid-phase nucleation initially and subsequently in the growth of DLA-like crystal patterns. The expression of possible reactions has been shown and properly described. With the help of the fundamentals of the DLA mechanism, the growth behaviour and fractal characteristics of the DLA-like crystal pattern are described. This investigated system may be helpful to understand the pattern formation mechanism, which is relevant to the pattern formation in complex biological processes occurring in nature.
Acknowledgments
Authors are thankful to the Institute for providing fellowship to RS. Authors would like to thank Central Instrument Facility, BIT Mesra for providing characterization facility.