ABSTRACT
The discovery that p-mode frequencies of low degree do not follow changes of solar surface activity during the recent solar minimum offers the possibility of a new diagnostic signature of the responsible pressure perturbation in the wave guiding medium, potentially rich of information regarding the structure of the Sun and the cause of the unusually long solar minimum. Magnetic fields, as well as temperature changes, introduce equilibrium pressure deviations that modify the resonant frequencies of p-mode oscillations. Assuming the perturbation to be caused by a horizontal layer of magnetic field located in a plane-stratified model of the Sun, we compile analytical frequency shifts and process them to allow direct comparison with observations. The effect of magnetism itself on the central p-mode frequencies can be neglected in comparison with the thermal effect of a perturbative layer buried in the solar interior. A parametric study shows that a layer as thin as 2100 km at subsurface depths is able to reproduce reported mean anomalous frequency shifts (not correlated with the surface activity), while a layer of size around 4200 km increasing by a small amount at depths near 0.08 R☉ can explain individual low-degree shifts. It is also possible to obtain the mean shifts via the upward motion through depths near 0.03 R☉ of a rising perturbative layer of thickness around 7000 km. Hence, the anomalous frequency shifts are best explained by thermal effects in the upper regions of the convection zone. The effects of latitudinal distribution are not treated here.
Export citation and abstract BibTeX RIS
1. INTRODUCTION
The 11 year cyclic variation of magnetic activity is the most obvious manifestation of solar variability. Since most forms of solar activity are magnetic in origin, they also follow this 11 year cycle, which refers to a quasi-periodic oscillation in the surface distribution of the solar magnetic field. A large body of helioseismic data convincingly shows that a cyclical variation in p-mode frequencies has been observed on the timescale of the solar activity cycle (see Howe 2008, for a recent review). During different time epochs, several groups have shown that low-degree modes (0 ⩽ l ⩽ 4) at increasingly higher frequencies become more sensitive to solar cycle effects (Woodard & Noyes 1985; Fossat et al. 1987; Pallé et al. 1989; Elsworth et al. 1990, 1994; Anguera Gubau et al. 1992; Chaplin et al. 1998; Jiménez-Reyes et al. 1998). The solar cycle dependence of the intermediate-degree (5 ⩽ l ⩽ 150–300) frequencies in the p-mode spectrum was established later (Libbrecht & Woodard 1990; Rhodes et al. 1993; Ronan et al. 1994; Bhatnagar et al. 1999; Howe et al. 1999). These observations report, over a solar cycle, peak shifts at a frequency of 3900 μHz, which are of order 750 nHz for low-degree modes (Chaplin et al. 1998) and 900 nHz for intermediate-degree modes (Libbrecht & Woodard 1991; Howe et al. 1999).
Bachmann & White (1994) have found hysteresis shapes among seven indices of solar activity in their relative variations during a 20 year period. Such relations were also found in p-mode frequencies of low degree by Jiménez-Reyes et al. (1998). In some cases, these observed hysteresis patterns start to repeat over more than one solar cycle, giving evidence that this is a normal feature of solar variability. Bachmann & White (1994) argue that hysteresis represents a real delay in the onset and decline of solar activity. In this view, Jiménez-Reyes et al. (1998) suggest that the hysteresis shape could be due to time-delayed responses to one single phenomenon, which can be located deep in the Sun, while Moreno-Insertis & Solanki (2000) find that the hysteresis in low-degree shifts is consistent with near-surface magnetic flux distributions. Latest analysis of intermediate-degree p-modes data shows the presence of hysteresis effects in near-surface toroidal magnetic fields (Baldner et al. 2009).
Only if one could remove contributions due to the near-surface perturbations on the p-mode spectrum, one can hope to evaluate the independent signals from the solar interior (Dziembowski & Goode 1997). The recent unusually long solar minimum provides such test ground. As of 2009 September, the start of the new cycle 24 is underway, but in the declining phase of cycle 23 and the following solar minimum, Broomhall et al. (2009b) have found unusually large differences between the frequencies observed in data from the Birmingham Solar Oscillations Network (BiSON) and the surface activity levels traditionally considered as proxy for the "surface frequency shifts". The discrepancy, of the order of 100 nHz, is reported for low-degree modes. Similar discrepancies are reported by Jain et al. (2009) in data from the Global Oscillation Network Group (GONG) and Michelson Doppler Imager (MDI) aboard the Solar and Heliospheric Observatory (SOHO) spacecraft and by Salabert et al. (2009) with data from the Global Oscillations at Low Frequencies (GOLFs) instrument aboard SOHO. The anomalous frequency shifts (not correlated with the surface activity) are therefore suggested to result from effects in the solar interior. They appear also to be a continuation of a possible "quasi-biennial" variation in the frequency shifts, superimposed on the solar cycle variation (Broomhall et al. 2009b). The 2 year period variation may be explained in terms of two types of dynamos operating at the base and in the subsurface of the convection zone (Benevolenskaya 1998). Alternatively, Salabert et al. (2009) attributes shift differences between low-degree modes to latitudinal effects.
There might be a source of confusion between the effect of magnetism and the effect of radial stratification at the base of the convection zone, which is in some sense an abrupt transition for the modes. Through theoretical modeling, Foullon & Roberts (2005) concluded that the effect of a buried magnetic layer on the frequencies of solar p-modes is too small to account for the changes in frequency over the solar cycle. For a realistic magnetic field at the base of the convection zone or in the anchoring zones of sunspots, the observed shifts (over the solar cycle) cannot be reproduced, while an explanation must be found through surface effects, such as the influence of the magnetic atmosphere (e.g., Campbell & Roberts 1989; Evans & Roberts 1992; Jain & Roberts 1996). The sunspot anchoring zone might still be relevant but a more thorough modeling of this region would be needed.
We consider a pressure perturbation on the p-mode spectrum, due to a bundle of magnetic flux localized in the stratified convection zone, which can be modeled as a horizontal layer of magnetic field. Thus, following the theory and a tractable analytical approximation developed by Foullon & Roberts (2005), we compile frequency shifts by surveying parameter conditions in the solar interior. In addition, we treat frequency shifts in the fashion of Broomhall et al. (2009b) in order to demonstrate the expected p-mode spectral signatures of thermal or magnetic perturbations that could be responsible for the anomalous shifts at solar minimum. This work may ultimately provide a diagnostic tool, which may help to locate the depth(s) of the perturbation(s) and possible departures from standard solar models.
2. METHODS
Foullon & Roberts (2005) provide an analytical estimate of the frequency shifts Δν on the p-mode frequencies ν of radial order n and degree l, which result from the effect of a thin non-magnetized isothermal layer of thickness h and lying between depths z = zp and z = zq within a polytrope (see Figure 1(a)). The layer may reside for instance in the convective overshoot region, at the base of the convection zone, or the anchorage zone of sunspots some 50 Mm below the surface. The polytropic index of the adiabatically stratified field-free media external to the layer is m = 3/2. We use the horizontal wavenumber kh, related to the degree l through , for solar radius R☉. The fractional frequency shift, f = Δν/ν, can be approximated for h = zq − zp ≪ zp as (Foullon & Roberts 2005, Equation (63))

where

and L1, L2 are generalized Laguerre polynomials,

Figure 1. (a) Sketch of equilibrium configuration for the three-layer model: an isothermal field-free slab () lies between two polytropes (
and
). In the polytropes, the square of the sound speed c2s(z) has a linear profile with slope A = g/m for constant gravitational acceleration, g. The sound speed is zero at the solar surface z = 0 and is assumed to be continuous across each interface, giving c2s(z) = c2c = Azp within the slab. See Foullon & Roberts (2005) for further descriptions of the model. (b) Representation of the effect of a thin isothermal layer of thickness h/2 rising from depths zp + h/2 to zp.
Download figure:
Standard image High-resolution imageThe density scale height, Ho, and κ are elements of the magnetoatmospheric wave equation (Goedbloed 1971; Nye & Thomas 1976; Foullon 2002; Foullon & Roberts 2005). In the field-free case, they can be fully developed as a function of m and khzp, namely,

and

f(h, zp) in Equation (1) is an approximation to the shifts between the model including the isothermal layer and the simple polytrope. Such an analytical approximation avoids essentially the numerical task to find roots to a dispersion relation that includes confluent hypergeometric functions. Since the model extends to infinite depth, the shifts include the effect of equilibrium pressure changes at depths z > zq. To estimate the shifts due solely to the thermal effect of a thin isothermal layer, one can first consider the analytical shifts f(h/2, zp) for a field-free layer of thickness h/2 located at depth zp and subtract from them the analytical shifts f(h/2, zp + h/2) for a layer of same thickness located immediately below the first layer (at depth zp + h/2). The result

represented in Figure 1(b), is an attractive analytical solution to the effect of a thin isothermal layer of thickness h/2 rising from depths zp + h/2 to zp and can be used to give an overall perturbation of thickness h to a polytropic profile of the solar interior, without the effect of equilibrium pressure changes at infinite depths. At depth zp + h/2, the associated change in the square of the sound speed, c2s, is

and the associated change in density, ρ, is
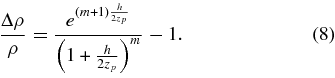
Note from Equation (1) that this model yields zero shifts for degree l = 0. Hence, in the two-dimensional parameter space of varying depths, zp, and layer thickness, h, and for any degree l > 0 and radial order n, we obtain separate fractional frequency shifts Δν/ν as a result of the presence of a field-free layer. The effect of magnetic fields structured in such a way as to produce a constant Alfvén speed may easily be introduced. However, since realistic magnetic fields can be neglected in this model (Foullon & Roberts 2005), we will not treat them here.
To allow comparison of the results with the helioseismic observations, we first obtain the frequency shift δνnl for a given l and n by multiplication of Δν/ν to the corresponding average values νnl pertinent to a quiet Sun as listed by Broomhall et al. (2009a, their Table 3). We then follow the mode inertia weighting procedure of Chaplin et al. (2004; see also Chaplin et al. 2007a),

which involves the mode inertia ratio, Qnl (Christensen-Dalsgaard & Berthomieu 1991), and the scaling function, F, to the frequencies νnl (Chaplin et al. 2004)

where ai∈ [−2.37116, 5.91549 × 10−3, −5.54457 × 10−6, 2.37824 × 10−9, −0.45240 × 10−12, 0.03275 × 10−15], with νnl in μHz.
3. RESULTS AND DISCUSSION
3.1. Mean Frequency Shifts
We first compile a mean frequency shift 〈δν〉 for νnl ranging between 2100 and 3500 μHz, to allow comparison with the BiSON observations (Broomhall et al. 2009b). The discrepancy between the observed frequency shifts and the surface activity proxies is of the order of 100 nHz (Broomhall et al. 2009b, their Figure 2). Figure 2(a) presents plots of 〈δν〉 in the h–zp parameter space. One feature of particular interest is a sign change in 〈δν〉 near depth zp ∼ 0.03 R☉ for every thickness h, from negative shifts in the deeper convection zone to positive shifts nearer the surface. Effects on frequencies expected from model changes in global mode helioseismology (e.g., Gough & Thompson 1991) indicate that the density profile changes may yield shifts of opposite sign to those expected from sound speed changes alone. As zp → 0, the density change associated with the layer model increases with an exponential factor compared to the sound speed change (see Equations (7) and (8)). This effect is due to the isothermal profile used in the layer. Thus, it is not surprising to find, for this particular model, a sign reversal zone near the surface.
Figure 2. Effect of a field-free layer in the h–zp parameter space as obtained in (a) mean frequency shift 〈δν〉, frequency shift for individual degrees (b) 〈δν1〉 for l = 1 and (c) 〈δν2〉 for l = 2, and (d) difference 〈δν2〉−〈δν1〉. Negative shifts are shown increasing in level (toward zero) with colors changing from blue to green and with dashed isocontours (of −500, −100, −50, and −10 nHz). Positive shifts are shown increasing in level with colors changing from red to yellow and with plain isocontours (of 10, 50, 100, and 500 nHz). White round markers in panels (b), (c), and (d) indicate the range of parameters taken to illustrate scenarios reproducing observed signatures of individual degree shifts. See text for details.
Download figure:
Standard image High-resolution imageThe thermal effect of an overall layer as thin as 0.003 R☉ ∼ 2100 km at subsurface depths is able to yield a positive 100 nHz shift, while a layer as thick as 0.03 R☉ ∼ 21 Mm near the base of the convection zone could yield a negative 100 nHz shift. Note that the sign of 〈δν〉 can be reversed by considering a downward motion or perturbation in the layer model (i.e., reversing the sign in Equation (6)). Considering a fixed thickness h, the shift increases in absolute value as zp decreases (except near the zone of sign reversal), which could be used to represent an upward motion of the layer. Thus, it is possible to obtain the observed anomalous shifts via the upward motion of a perturbative layer of thickness 0.01 R☉ ∼ 7000 km through the sign reversal zone (zp ∼ 0.03 R☉) and over a 2 year period. An expansion of the layer during such rising motion, which corresponds to an increase in thickness h while zp decreases, can also yield a non-negligible shift.
3.2. Frequency Shifts for Individual Degrees
We then compile the frequency shifts 〈δν1〉 and 〈δν2〉 for individual degrees l = 1 and l = 2, respectively, and for νnl ranging between 2000 and 3000 μHz, to allow comparison with the GOLF results (Salabert et al. 2009). Panels (b) and (c) of Figure 2 show similar sign changes at a given depth zp for every thickness h, as in panel (a), but the sign change in 〈δν1〉 occurs much deeper, near depth zp ∼ 0.08 R☉.
Panel (d) of Figure 2 shows the difference, 〈δν2〉−〈δν1〉, due to the presence of the thermal layer. This difference may be related to the negative 50 nHz difference observed during the recent solar activity minimum (Salabert et al. 2009, their Figures 2 and 3). A layer as thin as 0.006 R☉ ∼ 4200 km is able to generate such values near shallow depths of 0.06 R☉, and a layer twice thicker (0.012 R☉) located near 0.21 R☉ can also yield this effect. In addition, panels (b) and (c) show that it is possible to obtain an increase in the absolute value of 〈δν2〉, while 〈δν1〉 remains unchanged. Considering a perturbative layer located at the depth zp ∼ 0.08 R☉ with initial thickness h ∼ 0.005 R☉ increasing by a small amount (1400 km) to h ∼ 0.007 R☉ allows 〈δν1〉 to vary negligibly (around a value of 10 nHz) while 〈δν2〉 decreases by −50 nHz. The same scenario can be obtained at depth zp ∼ 0.21 R☉. White round markers in panels (b)–(d) indicate the range of parameters taken to illustrate both cases.
3.3. Correlation with Solar Activity from the Interior
Considering, for instance, a change in layer thickness, which may be related to changes in field strength in the buried magnetic layer, the associated frequency shifts are expected to be out of phase or even anticorrelated with the typical observed shifts that are themselves correlated with surface activity. This complies with the basic ideas for a dynamo based on magnetic flux tubes. In the progress of injection of a flux tube into the convection zone, the deeper it originates, the more amplified is its magnetic field strength when it emerges from the overshoot layer, the faster it rises and the lower in latitude it emerges at the solar surface as the cycle evolves (Fan et al. 1993). Hence, as the activity increases at the solar surface, the effect on p-modes of a buried layer decreasing in thickness (linked to a toroidal magnetic field decreasing in strength) would be expected to yield independent positive frequency shifts (see Figure 2(a)). The premise that the shifts due to a buried layer would not be correlated with the typical observed ones (correlated with surface activity) gives ground for inferring that the observed anomalous frequency shifts are more a consequence of buried layer than surface magnetism.
Helioseismic inversions for a change in sound speed (broadly meaning the effective wave speed at which the mode travels), as a function of depth, give further credence to this interpretation. By performing an inversion of the central frequencies in both MDI and GONG data, Eff-Darwich & Korzennik (2000) found small temporal variations of the sound speed distribution at the boundary between the radiative and convective zones. The changes, obtained with negligible error bars in the area of the conspicuous peak (relative to a grand average), follow a downward trend, which starts much earlier than the rise of the sunspot number, therefore indicating that physical processes take place before sunspot activity increases on the solar surface. Recently, Baldner & Basu (2008) obtained temporal variations in the sound speed at the base of the convection zone, as a result of solar activity, by inversion of a derived principal frequency component (reduced in data noise) and for MDI and GONG data sets spanning a complete solar cycle. Unlike atmospheric effects, the wave speed variations at the base of the convection zone, presumably caused by magnetic fields, are not expected to contribute significantly to the observed frequency shifts over the solar cycle (Foullon & Roberts 2005). However, their detection by inversion techniques could be an indication of the presence of a toroidal magnetic field stored in this region and whose field strength decreases as the solar activity increases at the surface.
Accordingly, considering a thermal perturbation in the upper regions of the convection zone related to the presence of this magnetic layer whose thickness varies with the field strength, one can explain the anomalous shifts observed in the present solar minimum.
4. CONCLUSION
The discovery that p-mode frequencies of low degree do not follow changes of solar surface activity during the recent solar minimum is potentially rich of information regarding the structure of the Sun, which has been neglected in the standard model of the solar interior. To tackle this problem, we have transformed a useful approximation to the effect of a thermal layer in a plane-stratified model of the Sun, initially developed to consider the effect of buried magnetic fields. The analytical approach provides a useful alternative to the perturbation theory approach. For any orientation of the (horizontal) magnetic field, the effect of magnetism itself on the central p-mode frequencies can be neglected in comparison with the thermal effect of the perturbative layer.
Our parametric study demonstrates the range of plausible diagnostics to the frequency shifts observed. Reported mean frequency shifts can be reproduced with a layer as thin as 2100 km at subsurface depths, while individual low-degree shifts can be explained with a layer of size ∼4200 km increasing by a small amount at depths near 0.08 R☉. It is also possible to obtain the mean shifts via the upward motion through the convection zone of a rising perturbative layer of thickness ∼7000 km. In this later scenario, the layer, presumably signaling new cycle magnetic flux, is expected to rise over a 2 year period through depths near 0.03 R☉. The scenario of a rising layer is consistent with the basic ideas for a dynamo based on magnetic flux tubes and previous observations of variations of the sound speed distribution with time at the base of the convective zone. Given that the inferred anomalous shifts are rough estimates and the model is a simplified one, we do not require to have mean frequency shifts and individual low-degree shifts explained by one single scenario, although this would be desirable for validation in future developments.
The discussed scenarios place the source of the anomalous shifts in the upper regions of the convection zone. They differ from the latitudinal effects proposed by Salabert et al. (2009), which are not treated here and may play an important role. Still, helioseismic inversions of data in the recent solar minimum would be desirable to locate more precisely the source of temporal variations of the interior stratification. The discussed diagnostics are not affected by absolute determinations of the base of the convection zone. While recent solar photospheric abundance analyses put new constraints on solar models, which in turn predict the base of the convection zone to be at a shallower depth than previously thought (e.g., Guzik et al. 2005), the results are still under intense debate (e.g., Basu et al. 2007; Chaplin et al. 2007b). Further analysis of the p-mode multi-modal parameters in the minimum epochs of solar activity is required to improve the diagnostics and locate the independent signals of the buried layers. Of particular interest are the details of theoretical oscillatory patterns in the frequency shifts of individual modes (Foullon & Roberts 2005), which could be used, if found in the helioseismic data, for further diagnostics. Modeling of the perturbative field-free layer could be improved analytically by an investigation of a polytropic layer with variable indices. Ultimately, such signatures of the responsible pressure perturbation in the wave guiding medium may help to understand departures from standard solar models and to address the cause of the recent unusually long solar minimum.
C.F. thanks A.-M. Broomhall from the BiSON group for helpful discussions and B. Roberts, with whom earlier work inspired this study.