Abstract
Hesperidin, a phytochemical renowned for its therapeutic effects including anticancer, antioxidant, and anti-inflammatory properties, encounters a significant limitation in its application due to its low bioavailability and restricted solubility in water. To surmount these challenges, we employed a spontaneous emulsification method to produce hesperidin nanoparticles. These nanoparticles, averaging 197.2 ± 2.8 nm, exhibited uniform dispersion (polydispersity index: 0.13), a zeta potential (ZP) of −28 mV, encapsulation efficiency of 84.04 ± 1.3%, and demonstrated stable and controlled release across various environments. Assessment of the nanoemulsions stability revealed remarkably high stability levels. Cytotoxicity evaluations (3-(4,5-dimethylthiazol-2-yl)-2,5diphenyl-2-H-tetrazolium bromide, neutral red, trypan blue, and lactate dehydrogenase) indicated that cancer cell viability following treatment with hesperidin nanoemulsion was concentration and time-dependent, significantly lower compared to cells treated with free hesperidin. The colony formation assay and cell morphology evaluation further corroborated the heightened efficacy of hesperidin in its nano form compared to the free form. In summary, hesperidin nanoparticles not only exhibited more potent anticancer activity than free hesperidin but also demonstrated high biocompatibility with minimal cytotoxic effects on healthy cells. These findings underscore the potential for further exploration of hesperidin nanoparticles as an adjunctive therapy in prostate cancer therapy.
Export citation and abstract BibTeX RIS
1. Introduction
Prostate cancer ranks among the top ten malignant diseases affecting men globally, often with a poor prognosis leading to fatalities in many countries [1]. Key characteristics of cancer cells include altered metabolism, disrupted cell cycles, frequent mutations, resistance to immune response, chronic inflammation, metastasis, and induction of angiogenesis [2]. Despite substantial advancements in cancer treatment, cancer stands as the second leading cause of death worldwide, surpassed only by cardiovascular disorders, and its risk escalates with age [3]. In recent years, there has been a notable surge in research dedicated to finding effective treatments for this disease [4]. Advancements in this realm have dramatically reshaped the spectrum of cancer treatment possibilities. A range of modalities, such as surgery, chemotherapy, radiotherapy, hormone therapy, and immunotherapy, are utilized in cancer treatment. Of these, surgery has been extensively employed. Nonetheless, the substantial hurdle of tumor recurrence accompanies this method. The prevalent issues of tumor recurrence and the side effects linked with chemotherapy, hormone therapy, and radiotherapy underscore the primary challenges in current cancer treatments. Consequently, there is a growing inclination towards adopting more targeted approaches for treating tumors, aiming for increased specificity and fewer side effects [5]. A primary advantage of nanotechnology in cancer treatment lies in its precise targeting of tumors. The paramount goal of utilizing nanotechnology in cancer treatment is the ability to discern between cancerous and non-cancerous cells [6]. Currently, most anticancer drugs lack this discriminatory ability, resulting in systemic administration causing widespread toxicity and side effects in various tissues. Consequently, this necessitates a reduction in the maximum therapeutic dose required for treatment [7].
In recent years, notable advancements have been made in the field of drug delivery systems. Effective delivery and increased bioavailability of anticancer drugs are always considered the key to the success of anticancer formulations. Nanoscale drug design has attracted considerable interest due to its promising benefits. These advantages include the ability to modify properties such as solubility, diffusion rate, drug release speed, biodegradability, and immunogenicity [8, 9]. Nanoparticles (NPs) play a dual role: they act as protective shields for drug compounds and also improve intracellular drug delivery by facilitating transport. The goal of these drug delivery systems is to reduce drug toxicity, enhance efficacy, eliminate the need for frequent administrations, and decrease the required injection volume compared to the free form of the drug [10]. More comfortable manufacturing/formulation of nanoemulsions (NEs), kinetic stability, and long-term storage capability are among the advantages of using NEs compared to other complex nano-based formulations [11]. The exploration of natural cytotoxic substances has long been integral to medical studies. The utilization of plant secondary metabolites, particularly those with a polyphenol structure, not only aids in mitigating the side effects associated with standard chemotherapy drugs but also proves economically advantageous. Among the polyphenolic natural products investigated for their anticancer potential, flavonoids stand out as particularly promising [12–14]. Hesperidin, a bioflavonoid, exhibits a diverse array of biological and medicinal activities, including antioxidant, anti-inflammatory, anti-hypertensive, and anticancer properties. Notably, it hinders cell proliferation in various cancer types, including colon, skin, tongue, lung, bladder, liver, and breast cancer, all while sparing normal cells from toxic effects [15]. Despite the numerous advantages of hesperidin, its application in cancer treatment encounters a significant hurdle and that is low bioavailability and limited solubility in water, coupled with susceptibility to environmental factors such as temperature, pH, and light. Even with substantial consumption of hesperidin-rich foods, the presence of its metabolites in the bloodstream remains low. This underscores the limitation of the therapeutic efficacy of oral hesperidin. Enhancing the pharmacokinetic properties of hesperidin post-oral administration becomes imperative [16, 17]. Addressing these challenges entails enhancing the physicochemical properties of hesperidin, which can be achieved by designing nano-carriers such as PLGA or hybrid nanoparticles for its transportation. Nanoencapsulation emerges as a viable solution, providing adequate stability and facilitating effective distribution upon oral administration [18, 19].
In this research, we successfully prepared hesperidin nanoemulsions with optimal physicochemical properties, including a size conducive to cancer cell uptake, uniform dispersion, high encapsulation efficiency (EE), stability with controlled release, and resilience to environmental changes and varying temperatures during two months of storage. Importantly, these nanoemulsions demonstrated compatibility with healthy cells, underscoring their biocompatibility. Our formulation utilized a novel, straightforward, and low-energy approach designed to improve the solubility and bioavailability of hesperidin. We subsequently conducted a comparative evaluation of the cytotoxic activity of these nanoemulsions against pure hesperidin on both prostate cancer and healthy cells. This delivery system for hesperidin shows potential for transporting other hydrophobic molecules or drugs. Notably, to the best of our knowledge, there are no prior reports on the investigation of the anticancer effects of hesperidin nanoemulsions specifically on prostate cancer cells (LNcap cell line).
2. Materials and methods
2.1. Materials
Hesperidin, Tween 80, Lecithin, Dimethyl sulfoxide (DMSO), 3-(4,5-dimethylthiazol-2-yl)-2,5diphenyl-2-H-tetrazolium bromide (MTT), neutral red (NR), RPMI-1640 medium, Trypsin-EDTA, trypan blue (TB), Dulbecco's phosphate-buffered saline, fetal bovine serum (FBS), penicillin, streptomycin, Crystal violet, propidium iodide (PI), and Acridine orange (AO) were obtained from Sigma–Aldrich Co. (Germany). Lactate dehydrogenase (LDH) cytotoxicity detection kit was from Takara Bio Inc (Japan). All other chemicals were of analytical grade.
2.2. Preparation of hesperidin nanoemulsions
The preparation of the hesperidin nanoemulsion followed the spontaneous emulsification method outlined by Saberi et al [20], with some modifications. In a concise overview, hesperidin was dissolved in DMSO and introduced to lecithin at a concentration of 2 wt%, stirred for 30 min at 800 RPM. Subsequently, this solution was combined with Tween 80 at 30 wt% (serving as a surfactant) and stirred for an additional 30 min at 800 RPM. In the final step, the resulting solution was added to deionized water and mixed on a stirrer for 60 min. The optimized conditions encompassed 10%wt oil content, 10%wt surfactant content, and 80% water phase content.
2.3. Microscopic examination of produced nanoemulsions
The morphology of nanoemulsions was investigated using a fluorescence microscope (Nikon Ts2R-FL Inverted Phase Contrast Microscope with NIS-Elements Advanced Research Viewer software).
2.4. Determination of particle size, PDI, and zeta potential (ZP)
The size, PDI, and ZP of the NPs were determined using dynamic light scattering (DLS) (Malvern Zetasizer, ZS, Malvern, UK). This device employs the DLS method to measure the size and PDI of emulsion particles.
2.5. Determination of EE
Initially, 1 ml of the central portion of the sample was extracted and dissolved in DMSO at a 1:10 ratio. Subsequently, the solution underwent centrifugation for 15 min at 3000 rpm. Following this, multiple samples of the supernatant were collected to quantify the enclosed amount of hesperidin in the oil-in-water (O/W) emulsions. Analysis involved measuring the absorbance at 360 nm using a UV/visible spectrophotometer, and EE of hesperidin in the dispersed phase was calculated using the following equation [21].

In the aforementioned formula, Ct denotes the concentration of hesperidin that persists in the emulsion at a given time, while C0 signifies the initial concentration of hesperidin.
2.6. In vitro release study
The release rate of hesperidin from nanoemulsions was assessed using the membrane dialysis technique. In this approach, 3 ml of hesperidin nanoemulsion was introduced into dialysis bags with a molecular weight cutoff of 14–12 kDa. These bags were immersed in 200 ml of pH 7.4 PBS buffer, stirred at 200 rpm, and maintained at a temperature of 37 °C. At specified intervals, 5 ml samples were withdrawn from the release medium, and an equivalent volume of fresh buffer was added to ensure a constant volume. UV-vis spectrophotometry at a wavelength of 360 nm was employed to measure the amount of hesperidin released from the dialysis bag.
2.7. Stability study
After production, the nanoemulsions were immediately stored at temperatures of 4 °C and 25 °C, protected from light. The average diameter, PDI, ZP, EE, phase separation, and turbidity were examined on the 15th, 30th, and 60th days after preparation.
2.8. Turbidity measurement
To assess the turbidity of the nanoemulsion over time, 1 ml of the nanoemulsion was diluted tenfold in deionized water prior to measurement. The turbidity was determined using a UV/visible spectrophotometer at a wavelength of 360 nm, with deionized water serving as the blank. Each measurement was conducted in triplicate, and samples were collected at various intervals for turbidity measurement [22].
2.9. Nanoemulsion stability in cell culture environment
To assess the stability of the synthesized nanoemulsions in cell culture media, both media with and without FBS were utilized. The synthesized nanoemulsions were added to RPMI culture medium with and without 10% FBS, followed by an incubation period under standard conditions for 24 and 48 h. The average particle diameter was measured using DLS.
2.10. Colloidal stability
To simulate their in vivo behavior, the nanoemulsions were diluted at a ratio of 1:4 in PBS, RPMI, and plasma. Subsequently, the diluted nanoemulsions were incubated at 37 °C with agitation at 150 rpm for 24 and 48 h.
2.11. Cell culture
The LNcap cell line was sourced from the Pasteur Cell Bank Institute and cultured in a medium comprising 90% RPMI, 10% FBS, 1% antibiotic (penicillin + streptomycin), and 2% L-glutamine. The cells were then incubated in a controlled environment with 5% CO2 and 95% humidity.
2.12. Cell cytotoxicity evaluation tests of hesperidin nanoemulsions
2.12.1. TB exclusion assay
LNcap cells were seeded at a density of 5 × 105 cells per well in a six-well culture plate, with each well containing 2 ml of complete culture medium. The cells were then cultured at 37 °C and allowed to incubate for 24 h. Subsequently, they underwent treatment with various concentrations of hesperidin nanoemulsions (0, 10, 20, 30, 40, 50, and 60 μg ml−1) and free hesperidin (0, 10, 20, 30, 40, 50, and 60 μg ml−1) for a duration of 48 h. Three replicates were maintained for each concentration. Following the treatment period, the cells were harvested, stained with TB, and the number of living cells, as well as the total cell count, was determined using an inverted microscope.
2.12.2. MTT assay
A total of 104 cells were seeded into each well of a 96-well culture plate and incubated for 24 h at 37 °C. Subsequently, the cells were treated in triplicate with varying concentrations of hesperidin nanoemulsion (0, 10, 20, 30, 40, 50, and 60 μg ml−1) and free hesperidin (0, 10, 20, 30, 40, 50, and 60 μg ml−1). The cells were further incubated for 24 and 48 h. Following this incubation period, 20 μl of MTT solution (5 mg ml−1 in PBS) was added to each well and incubated for 4 h in the dark. Afterward, 100 μl of DMSO was added to dissolve the formazan crystals, and the plate was shaken. Using a microplate reader, the absorbance of the samples was measured at 570 nm, and the results were calculated using the following formula:
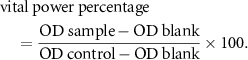
2.12.3. Neutral Red Uptake assay (NRU)
A total of 104 LNcap cells were seeded into each well of a 96-well plate and incubated for 24 h under standard conditions to ensure cell attachment to the well bottom. Subsequently, varying concentrations of hesperidin nanoemulsion (0, 10, 20, 30, 40, 50, and 60 μg ml−1) and free hesperidin (0, 10, 20, 30, 40, 50, and 60 μg ml−1) were added to the wells in triplicate and incubated for 24 and 48 h in the incubator. At the end of each treatment period (24 and 48 h), 10 μl of NR was added to each well and incubated for 2 h at 37 °C. The supernatant was then discarded, and the cells were washed three times with PBS. Subsequently, lysing buffer (composed of 50% 96% alcohol, 4% acetic acid, and 46% distilled water) was added. Finally, using a microplate reader at a wavelength of 540 nm, the optical density of the samples was measured.
2.12.4. Lactate dehydrogenase (LDH) leakage assay
To quantify the amount of released LDH, a colorimetric method was employed. A total of 104 LNcap cells were seeded into each well of a 96-well culture microplate. Once the cells adhered, they were treated with varying concentrations of hesperidin nanoemulsion and free hesperidin, followed by a 48 h incubation under standard cell culture conditions. Subsequently, 100 μl of the supernatant from each well was transferred to a flat-bottomed 96-well plate. Then, 100 microliters of the reaction solution, prepared according to the kit's instructions, was added to each well and shaken for 30 min at room temperature using an orbital shaker. Following this, the absorption was measured using a microplate reader at a wavelength of 490 nm. A 0.1% solution of Triton X-100 in PBS served as a positive control, while cells cultured in an empty culture medium were employed as a negative control. Finally, the LDH release was calculated using the provided formula [23].

2.13. Cell morphology
Morphological changes and the growth rate of cancer cells were examined using an inverted light microscope. Cancer cells treated with the IC50 concentration obtained for both hesperidin nanoemulsion and free hesperidin were incubated for 48 h. Cell images were captured using an inverted light microscope at a magnification of 400X.
2.14. Clonogenic assay
For the investigation of colony formation, cancer cells were cultured in 6-well culture plates with an initial seeding of 1000 cells per well. After ensuring cell attachment, the cells were treated with hesperidin nanoemulsion and free hesperidin at their respective IC50 concentrations. The cells were then incubated at a temperature of 37 °C with 5% CO2 for 7–10 d until optimal colonies were formed. Fixation was carried out using methanol, and the colonies were visualized by staining with 1% crystal violet dye. The quantification of colonies was performed using Image J software [24].
2.15. Biocompatibility evaluation tests
2.15.1. Preparation of Peripheral blood mononuclear cells (PBMCs) and assessment of nanoemulsion cytotoxicity
PBMCs were isolated from healthy volunteers using histopaque and the standard laboratory method for density gradient centrifugation. Subsequently, 105 cells were seeded into each well of a 96-well culture plate. The cells were treated with varying concentrations of hesperidin nanoemulsion (0, 10, 20, 30, 40, 50, and 60 μg ml−1) and free hesperidin (0, 10, 20, 30, 40, 50, and 60 μg ml−1) in triplicate and incubated for 24 and 48 h. The cytotoxicity of hesperidin nanoemulsion and free hesperidin on PBMCs was then assessed using the MTT assay.
2.15.2. Hemolysis test
Fresh blood samples obtained from healthy volunteers were collected in heparin tubes. Red blood cells (RBCs) were isolated by centrifuging blood samples at 800 g for 10 min and washed with PBS. Subsequently, 200 μl of each concentration of hesperidin nanoemulsion and free hesperidin (ranging from 0 to 60 μg ml−1) in triplicate were added to 200 μl of a 10% v/v RBC suspension and incubated at 37 °C for 1 h. Following incubation, the samples were centrifuged at 13 400 RPM for 5 min. The amount of hemoglobin released from RBCs into the supernatant of the samples was measured using a microplate reader at a wavelength of 540 nm. Triton X-100 served as a positive control (inducing 100% lysis of RBCs), and PBS was used as a negative control (without lysis of RBCs). Hemolysis percentages were calculated using the provided formula [25],

2.16. PI/AO double staining
In this study, to assess the occurrence of apoptosis or necrosis in LNcap cells following treatment, distinct T25 flasks were set up for various treatment and control groups. Subsequently, each flask was seeded with one million LNcap cells and treated with the previously determined IC50 concentration for hesperidin and hesperidin nanoemulsion. One flask was designated as the control group and remained untreated. After a 48 h incubation period, the culture medium was aspirated, and cells were thoroughly washed with fresh culture medium. Following this, one milliliter of culture medium containing 10 μl of AO was added to each flask, and the mixture was incubated for 20 min in darkness. Subsequent to another wash with culture medium, a solution comprising 1 ml of culture medium and 10 μl of PI was introduced, and the flasks were kept in the dark for 5 min. Ultimately, cells were observed and examined using a fluorescence microscope [26].
3. Results and discussion
3.1. Morphology of prepared NPs
The physicochemical characteristics of the produced NPs are pivotal considerations as they significantly influence nanoparticle performance within the body. Factors such as size, shape, particle charge, surface alterations, stability, and toxicity are intimately connected to the behavior of nanoparticles [27]. Microscopic analysis of hesperidin NPs revealed spherical particles characterized by smooth surfaces and a uniform distribution (figure 1). The presence of small droplet sizes in nanoemulsion signifies a dispersed and stable system, and the spherical nature of particles is indicative of system stability [28]. Additionally, studies by Zhou et al suggest that spherical nanoparticles exhibit efficient cellular penetration [29].
Figure 1. Microscopic image of hesperidin nanoemulsions: The microscopic image of hesperidin nanoemulsions showed spherical particles with smooth surfaces and uniform distribution of particles.
Download figure:
Standard image High-resolution image3.2. Evaluation of size and size distribution of nanoemulsions
The size and size distribution of hesperidin nanoemulsion were analyzed using DLS at 25 °C. The measurements revealed nanoparticles with an average diameter of 197.2 ± 2.8 nm and a PDI of 0.13, indicating a monodisperse distribution (figure 2). The distribution of particle sizes is a crucial factor for efficiency, physical stability, and rheological properties of the product. It serves as an indicator of particle size uniformity, ranging between 0 (monodisperse) and 1 (polydisperse) [1]. Smaller particle sizes contribute to enhanced bioavailability, surface-to-volume ratio, stability, and transparency of the nanoparticle system [20].
Figure 2. Particle size and size distribution analysis using dynamic light scattering (DLS).
Download figure:
Standard image High-resolution image3.3. Determination of EE
The EE, representing the loading efficiency of the formulation, was notably high for the produced hesperidin nanoemulsion, measuring approximately 84.04 ± 1.3%.
3.4. Storage stability study
In commercial applications, ensuring the physical and chemical stability of nanoemulsion-based products is paramount, particularly when subjected to adverse environmental conditions such as temperature variations, mechanical stresses, and alterations in ionic strength during production, storage, transportation, or application (e.g. injection into the body). Emulsion instability can lead to droplet accumulation, resulting in creaming and eventual separation of the oily phase from the aqueous phase [30]. The small size of nanoemulsion particles confers resistance to sedimentation, as the diffusion speed driven by Brownian motion surpasses the sedimentation speed induced by gravity. The small particle size, high stability, and transparency of nanoemulsions, particularly those based on lecithin, make them a preferred choice for various applications, especially in pharmaceutical formulations, owing to their small droplet size and superior storage stability [31, 32].
Particle size measurement serves as an excellent indicator for studying formulation stability, with a rapid increase in particle size over time signaling low system stability. Figure 3(a) depicts changes in nanoemulsion droplet size during a 60 day storage period at two temperatures, 4 and 25 °C. The increase in droplet size occurred at a relatively low rate during the storage period at both temperatures. In this study, droplet size varied from 197.2–219.9 nm (4 °C) and 197.2–240.5 nm (25 °C) (p < 0.05), remaining within an acceptable range conducive to optimal uptake by tumor cells. The observed increase in droplet size over time is attributed to the movement of dispersed phase droplets within the continuous phase, enhancing the likelihood of droplet collisions. Due to the direct influence of temperature on particle movement, there was a more notable increase in particle size observed at 25 °C compared to 4 °C. Throughout the 60 day evaluation period, the nanoemulsions maintained a PDI below 0.2 at 4 °C and a dispersion index below 0.3 at 25 °C, highlighting the sustained stability of this formulation and the success of the synthesis, as depicted in figure 3(b). The heightened surface charge of the particles ensures a uniform and homogeneous dispersion, preventing sedimentation. In a standard oil-in-water emulsion, a more negative ZP correlates with increased emulsion stability. Moreover, particles with a partial negative charge and an average size of 150 nm exhibit enhanced accumulation within tumor cells [33]. Figure 3(c) illustrates the measurement of the ZP of the nanoemulsion over a 60 day period at two temperatures, 4 and 25 °C. The ZP value of hesperidin nanoemulsion on day zero was −28 mV, which showed a minimal change to −24.7 mV at 25 °C and −25.8 mV at 4 °C after 60 d of storage. Importantly, these variations were not statistically significant (p > 0.05).
Figure 3. Evaluation of the stability of nanoemulsions during 60 d of storage at different temperatures. Different lowercase letters indicate significance at the p < 0.05 level. Values and bars represent mean ± SD (n = 3). (a); particle size (b); particle size distribution (c); zeta potential (d); turbidity (e); encapsulation efficiency.
Download figure:
Standard image High-resolution imageThe turbidity of the produced nanoemulsions was assessed at temperatures of 25 and 4 °C over a 60 day period. As depicted in figure 3(d), the turbidity of stored nanoemulsions increased during the storage time, demonstrating statistical significance (p < 0.05). Additionally, higher turbidity was observed in nanoemulsions stored at 25 °C compared to 4 °C (figure 3(d)). The reduction in transparency of the nanoemulsion at higher temperatures can be attributed to increased droplet movement and collision, leading to an augmentation in particle size. Moreover, the results indicate that changes in nanoemulsion turbidity are primarily dependent on alterations in particle size. Given their diminutive droplet size, nanoemulsions typically exhibit transparency and enhanced stability compared to microemulsions against coagulation and aggregation. These physicochemical traits render nanoemulsions highly suitable for practical and pharmaceutical applications, emphasizing their small droplet size and prolonged stability. The minimal scattering of light waves by the tiny droplets enhances the overall transparency of the nanoemulsion. Due to the hydrophobic nature of hesperidin, consistent with expectations from nanoemulsion systems, the encapsulation content of hesperidin remained high throughout all the times and temperatures studied. EE was 84.04% on day 0, and it showed robust values, specifically 74.9% at 25 °C and 79.3% at 4 °C. Notably, the percentage of EE after 60 d of storage was statistically significant when compared to day 0 (p < 0.05). A study by Zeng et al which stored hesperetin nanoemulsion for 50 d at 25 °C, observed variations in average particle size (12.63–16.19 nm), drug content (9.49–9.72 mg ml−1), and PDI (0.072–0.095). The physicochemical properties of hesperetin nanoemulsion exhibited minimal fluctuations, maintaining a pale yellow and transparent appearance throughout the storage period, indicative of the excellent stability of the produced nanoemulsions (figure 3(e)) [34].
3.5. Colloidal stability
Hesperidin nanoemulsion was subjected to incubation for 24 and 48 h in varying environments, including PBS, plasma, RPMI culture medium, and culture medium containing 10% FBS, all at 37 °C. Subsequently, the particle size was meticulously examined. Figure 4 illustrates that, remarkably, the diameter of the nanoemulsions remained unchanged in all tested environments. This steadfast behavior underscores the robust stability of the nanoemulsions across diverse conditions.
Figure 4. Evaluation of colloidal stability of nanoemulsions in different environments, including plasma, phosphate-buffered saline (PBS), and cell culture medium. Different lowercase letters indicate significance at the p < 0.05 level. Values and bars represent mean ± SD (n = 3).
Download figure:
Standard image High-resolution image3.6. In vitro release study
An insightful study for predicting the behavior of nanoemulsion in the body involves evaluating the release of the encapsulated substance. In the case of O/W nanoemulsions, the substance enclosed in the oily phase is released into the aqueous phase. The advantageous aspect of smaller nanoemulsion particles is the larger contact surfaces, favoring substance release [34]. To assess the release of hesperidin from the produced nanoemulsions, the release was measured in PBS over 72 h. The release of hesperidin exhibited a biphasic pattern, as depicted in figure 5. It commenced with an initial phase characterized by a steep slope, followed by a subsequent phase of slow and controlled release. During the initial hours, a substantial portion of loaded hesperidin, approximately 30% of the total, was rapidly released within the first 10 h. The gradual release of hesperidin continued to increase over time, reaching maximum release after 72 h. The rapid initial release is attributed to hesperidin adsorbed on the nanoparticle surface, while the sustained release affirms the encapsulation of hesperidin within the nanoparticles.
Figure 5. Hesperidin release from nanoparticles over time. Different lowercase letters indicate significance at the p < 0.05 level. Values and bars represent mean ± SD (n = 3).
Download figure:
Standard image High-resolution image3.7. Cytotoxicity evaluation tests of hesperidin nanoemulsions
3.7.1. Cell viability assay
As depicted in figure 6, the hesperidin nanoemulsion exhibited an IC50 concentration of 31.25 ± 2.5 μg ml−1, while free hesperidin demonstrated an IC50 concentration of 60.25 ± 2.5 μg ml−1, effectively eliminating 50% of prostate cancer cells. The impact of both hesperidin nanoemulsion and free hesperidin on cancer cell viability was concentration-dependent. Notably, hesperidin nanoemulsion induced a more pronounced reduction in cell viability compared to free hesperidin. At a concentration of 60 μg ml−1, hesperidin nanoemulsion achieved an 83% reduction in LNcap cell viability. In a 2010 study, Lee et al explored the effects of hesperidin on the growth of androgen-dependent prostate cancer and breast cancer cells. Their findings revealed that hesperidin significantly reduces cell growth and inhibits cancer cell adhesion to the extracellular matrix in both cancer types. This suggests that hesperidin has the potential to inhibit the growth of androgen-dependent prostate cancer and breast cancer cells [35].
Figure 6. Viability of cancer cells after 48 h of treatment with different concentrations of free hesperidin (Hes) and hesperidin nanoemulsions (Hes-NE) compared to the control group. Different uppercase letters indicate significance at the p < 0.05 level. Values and bars represent mean ± SD (n = 3).
Download figure:
Standard image High-resolution image3.7.2. MTT assay
The MTT test results revealed concentration-and time-dependent cytotoxic effects of both hesperidin and hesperidin nanoemulsion on cancer cells (p < 0.05). As illustrated in figure 7, hesperidin nanoemulsion exhibited the ability to induce a 50% reduction in cancer cell viability at a concentration of 31.08 ± 2 μg ml−1 after 24 h and at a concentration of 20.4 ± 3.5 μg ml−1 after 48 h (IC50). In contrast, free hesperidin did not achieve a 50% reduction in cancer cell viability within the 24 h period for any concentration used in this study. However, after 48 h, it demonstrated a 50% reduction at a concentration of 47.6 ± 1.5 μg ml−1. A comparable study by Ersoz et al demonstrated that hesperetin nanoparticles exert a dose-and time-dependent decrease in the mitochondrial function of C6 glioma cancer cells. The cell viability after 48 h significantly decreased compared to 24 h, indicating the controlled release of hesperetin from PLGA nanoparticles. Moreover, this study highlighted the enhanced cytotoxic activity of hesperetin nanoparticles compared to free hesperetin [36]. In another investigation, the cytotoxic effects of zinc cross-linked nanocomposites containing hesperidin were assessed on HCT116 colon cancer cells. MTT results confirmed that nanocomposites containing hesperidin decreased cancer cell viability depending on concentration [37]. Moreover, Sulaiman et al noted that hesperidin loaded into gold nanoparticles exhibited increased anticancer activity against MDA-MB-231 breast cancer cells compared to free hesperidin and gold nanoparticles alone. The cytotoxic effects of hesperidin loaded into gold nanoparticles displayed a dose-dependent increase, underscoring the enhanced anticancer activity of hesperidin after functionalization with gold nanoparticles. It is noteworthy that the concentration of Hsp-AuNPs synthesized in this instance was notably lower than that of hesperidin. The decline in cell viability with increasing nanoparticle concentration signifies a greater accumulation of nanoparticles within cells, inducing stress and eventual cell demise [33].
Figure 7. MTT assay; Study of cytotoxicity of different concentrations of free hesperidin (Hes) and hesperidin nanoemulsions (Hes-NE) after 24 (a) and 48 (b) hours. Different uppercase letters indicate significance at the p < 0.05 level. values and bars represent mean ± SD (n = 3).
Download figure:
Standard image High-resolution image3.7.3. NRU assay
NRU assay, widely used in biomedicine, relies on the interaction of live cells with NR dye within lysosomes. NR, a neutral cationic dye capable of penetrating cells, is absorbed based on the cell's ability to maintain a pH gradient. At physiological pH, the dye, being charged, enters the cell. However, within lysosomes where there's a proton gradient (low pH), the dye becomes charged and stays confined [38, 39]. Comparing the viability results of cancer cells treated with different concentrations of hesperidin nanoemulsion and free hesperidin after 24 and 48 h using the NR method, consistent outcomes were observed with the MTT and TB tests. Hesperidin nanoemulsion, at a concentration of 38.4 ± 2.6 μg ml−1 after 24 h and 29.4 ± 3.5 μg ml−1 after 48 h, demonstrated the ability to induce a 50% reduction in LNcap cells (IC50). Free hesperidin, within 24 h, did not achieve a 50% reduction. However, after 48 h, it accomplished a 50% reduction at a concentration of 52.08 ± 2.1 μg ml−1, indicative of its IC50 (figure 8). The NRU results further confirmed that the cytotoxicity of both hesperidin nanoemulsion and free hesperidin is concentration and time-dependent, with a higher lethality rate observed at 48 h compared to 24 h.
Figure 8. Neutral red uptake (NRU) assay: Study of cytotoxicity of different concentrations of free hesperidin (Hes) and hesperidin nanoemulsions (Hes-NE) after 24 (a) and 48 (b) hours. Different uppercase letters indicate significance at the p < 0.05 level. Values and bars represent mean ± SD (n = 3).
Download figure:
Standard image High-resolution image3.7.4. LDH assay
LDH is a reliable indicator of cell death, as it is released into the extracellular space when the plasma membrane is damaged during prolonged exposure to cytotoxic compounds. The level of cell viability can be determined by measuring the amount of LDH released into the supernatant [33, 40]. In the study, the supernatant of cells treated with different concentrations of hesperidin nanoemulsion and free hesperidin was analyzed for LDH release. As illustrated in figure 9, the LDH level in the supernatant of all treated cells escalated in a concentration-dependent manner. The percentage of cytotoxicity in cells exposed to hesperidin nanoemulsion surpassed that of thosetreated with free hesperidin. Specifically, at a concentration of 60 μg ml−1, hesperidin nanoemulsion demonstrated approximately 67.3% cytotoxicity, whereas free hesperidin at the same concentration exhibited roughly 40.9% cytotoxicity against LNcap cells compared to the positive control group (100%). This aligns with findings from Natarajan et al who observed that hesperidin at a concentration of 80 M significantly increased LDH release from treated MCF7 cells [40].
Figure 9. Lactate dehydrogenase (LDH) assay; Study of cytotoxicity of different concentrations of free hesperidin (Hes) and hesperidin nanoemulsions (Hes-NE). Different uppercase letters indicate significance at the p < 0.05 level. values and bars represent mean ± SD (n = 3).
Download figure:
Standard image High-resolution image3.8. Biocompatibility evaluation tests
3.8.1. Assessment of hesperidin nanoemulsion cytotoxicity on PBMC cells
The biocompatibility of nanoparticles is crucial in developing drug delivery systems to minimize side effects on normal cells [41]. In this study, PBMC cells were used to investigate the cytotoxic effects of hesperidin nanoemulsion and free hesperidin. The MTT test results indicated that both hesperidin nanoemulsion and free hesperidin had minimal toxic effects on PBMC cells, and IC50 concentrations were not achieved in any of the concentrations used. Specifically, at the highest concentration (60 μg ml−1), free hesperidin killed only 20% of normal cells in 24 h and 31% in 48 h. Hesperidin nanoemulsion, at the highest concentration used, killed 17% of PBMC cells in 24 h and 26% in 48 h (figure 10). These results suggest that hesperidin nanoemulsion, compared to free hesperidin at the concentrations used, had relatively fewer toxic effects on PBMC cells. This could be attributed to the different mechanisms by which free hesperidin and hesperidin nanoemulsion enter the cells. Free hesperidin enters the cell via diffusion through the membrane, while hesperidin nanoemulsions enter the cell through endocytosis, gradually releasing the encapsulated substance inside the cell and resulting in fewer cytotoxic effects. Overall, this indicates the potential use of this drug delivery system for cancer treatment with reduced side effects. Maheswari and colleagues employed the L929 fibroblast cell line to assess the biocompatibility of the produced nanosystem. They observed that natural phenolic cytotoxic drugs, such as hesperidin and eugenol, were well-tolerated by L929 cells and had minimal effects on cell viability. Intriguingly, they found that eugenol displayed lower biocompatibility for normal cells compared to hesperidin, especially at higher concentrations. However, following encapsulation, the biocompatibility of both substances increased, with all samples demonstrating good biocompatibility, achieving IC50 values above 250 μg ml−1 [41]. In another study, the highest cytotoxic effect of gold nanoparticles containing hesperidin on breast cancer cells was observed at a dose of 125 μg ml−1 (88%). Treatment with hesperidin alone induced 45.3% toxicity. Notably, such activity was not observed in the normal human breast epithelial cell line (HBL-100), where the viability of normal cells was higher compared to cancer cells [33]. Duranoğlu et al investigated the effects of different concentrations of hesperetin and hesperetin nanoparticles on L929 fibroblast cells (normal cells). Their findings led to the conclusion that hesperetin and hesperetin nanoparticles have mild and similar cytotoxic effects on normal cells, indicating biocompatibility [42]. In a recent study, the selective toxicity effects of a nanoemulsion containing Ferula Gummosa essential oil on cancer cells were confirmed in comparison with normal cells. This selectivity was attributed to the complex variations in the biochemical responses of cancer cells compared to normal cells. Additionally, the heightened metabolic activities of cancer cells make them more susceptible to cytotoxic compounds due to the dynamic antioxidant defense network [43].
Figure 10. 3-(4, 5-dimethylthiazolyl-2)-2, 5-diphenyltetrazolium bromide (MTT) assay: Study of cytotoxicity of different concentrations of free hesperidin (Hes) and hesperidin nanoemulsions (Hes-NE) on peripheral blood mononuclear cells (PBMC) after 24 (a) and 48 (b) hours. Different uppercase letters indicate significance at the p < 0.05 level. values and bars represent mean ± SD (n = 3).
Download figure:
Standard image High-resolution image3.8.2. Hemolytic assay
Figure 11 displays the percentage of hemolysis in RBCs after exposure to six different concentrations of free hesperidin and hesperidin nanoemulsion for 1 h at 37 °C. Notably, the hemolysis induced by hesperidin was consistently higher than that caused by hesperidin nanoemulsion across all concentrations. The hemolysis percentage for hesperidin nanoemulsion at concentrations of 10, 20, 30, and 40 μg ml−1 remained below 5%, meeting the acceptable criterion according to ASTM E2524-08 standards [44]. However, concentrations of 50 and 60 μg ml−1 led to hemolysis exceeding 5%. Free hesperidin demonstrated acceptable hemolysis levels (below 5%) at concentrations of 10 and 20 μg ml−1. Following ASTM standards, hesperidin nanoemulsion at low concentrations appears safe for internal use. In a study by Sulaiman et al gold nanoparticles containing hesperidin exhibited no harmful activity on RBC morphology at low concentrations, indicating high blood compatibility. In contrast, free hesperidin exhibited more pronounced destructive effects on RBC cell membranes. This study highlights the potential of hesperidin nanoparticles for therapeutic applications with minimal side effects [33].
Figure 11. In vitro hemolytic activity of different concentrations of free hesperidin and hesperidin nanoemulsions. Different uppercase letters indicate significance at the p < 0.05 level. values and bars represent mean ± SD (n = 3).
Download figure:
Standard image High-resolution image3.9. Characteristics of growth and morphology of cancer cells
Cell morphological changes induced by the treatments were examined using an inverted light microscope. In the control group, cancer cells exhibited a robust growth and proliferation rate, maintaining a healthy appearance with no signs of apoptosis (figure 12(a)). On the contrary, both the hesperidin nanoemulsion and free hesperidin treatment cohorts exhibited a substantial decrease in cell growth compared to the control group. Distinctive alterations, including variations in cell size, shrinkage, and a rounded morphology, were evident in both treatment groups. Notably, the hesperidin nanoemulsion treatment induced more pronounced cell changes than free hesperidin. This included a significant reduction in the number of viable cells and increased intercellular distances, indicative of apoptosis (figures 12(b) and (c)). In a similar vein, Ali et al reported analogous findings, observing that hesperidin nanoparticles triggered apoptotic features, including nuclear fragmentation and changes in cancer cell morphology, leading to a reduced cell population. Control cells maintained a normal nucleus with standard size and shape [44]. Consistent with these findings, Sulaiman and colleagues observed various morphological changes in cells treated with gold nanoparticles containing hesperidin, including the formation of clusters with reduced expansion and diminished cell-cell communication, distinguishing them from the control cells [33].
Figure 12. Morphological changes induced in LNCaP cells treated with IC50 concentration of hesperidin nanoemulsions and free hesperidin (scale bar 12.5 μm, magnification 400X) (a); LNCaP cells without treatment (b); cells treated with IC50 concentration of free hesperidin (c); cells treated with IC50 concentration of hesperidin nanoemulsions.
Download figure:
Standard image High-resolution image3.10. Colony formation assay
The clonogenic assay, a standard method for assessing a cell's ability to form colonies, was employed to validate the growth inhibition results of hesperidin nanoemulsion. LNcap cell line cells were treated with IC50 concentrations determined for both hesperidin nanoemulsion and free hesperidin over a period of 7–10 d. Figure 13 illustrates that treatment with hesperidin nanoemulsion and free hesperidin resulted in a reduced capacity for colony formation in LNcap cells compared to the control group. The reduction in colony formations was significantly (p < 0.05) greater in the group treated with hesperidin nanoemulsion than in the group treated with free hesperidin. Ali et al demonstrated that hesperidin nanoparticles significantly decreased the number of colonies in MCF-7 cells in a concentration-dependent manner, surpassing the efficacy of free hesperidin. This heightened performance of hesperidin nanoparticles may be attributed to the slow absorption of nanoparticles into cells and the gradual release of hesperidin from within the nanoparticles [44]. Additionally, another study confirmed the inhibition of cancer cell colony formation by hesperetin [45].
Figure 13. Colony formation assay; (I) macroscopic images; colony formation capacity changes induced in LNCaP cells treated with IC50 concentration of hesperidin nanoemulsions and free hesperidin. (a); LNCaP cells without treatment (b); cells treated with IC50 concentration of free hesperidin (c); cells treated with IC50 concentration of hesperidin nanoemulsions. (II) Microscopic images (crystal violet staining); colony formation capacity changes induced in LNCaP cells treated with IC50 concentration of hesperidin nanoemulsions and free hesperidin. (a); LNCaP cells without treatment (b); cells treated with IC50 concentration of free hesperidin (c); cells treated with IC50 concentration of hesperidin nanoemulsions (scale bar 50 μm, magnification 400X). (III) percentage of colony formation capacity in LNCaP cells treated with IC50 concentration of hesperidin nanoemulsions and free hesperidin. Different uppercase letters indicate significance at the p < 0.05 level. values and bars represent mean ± SD (n = 3).
Download figure:
Standard image High-resolution image3.11. AO/PI test results
Among the various tests that are used to detect the type of cell death, the AO/PI test is one of the simple and highly accurate tests because it allows the detection of early or delayed apoptotic cells and necrosis. Chromatin condensation and nuclear fragmentation are hallmarks of apoptotic cells, in AO/PI double staining, AO penetrates all cells and shows nuclei green, while PI turns the core orange only when cell membrane integrity is lost. Thus, living cells show a normal green nucleus, early apoptotic cells show a bright green nucleus with condensed or fragmented chromatin, late apoptotic cells show condensed and fragmented orange chromatin, and cells that have undergone necrosis show an orange nucleus with a normal structure. In the present study, LNcap cancer cells were treated for 48 h with the IC50 concentration obtained for hesperidin nanoemulsion and free hesperidin, and the type of cell death of the cells was investigated using a fluorescence microscope. As can be seen in figure 14, the number of apoptotic cells in the group treated with hesperidin nanoemulsion has clearly increased compared to free hesperidin. Cells treated with free hesperidin underwent both primary and secondary apoptosis, while most cells treated with hesperidin nanoemulsion underwent secondary apoptosis. The percentage of necrosis in both treated groups was low. The results of AO/PI test showed that hesperidin and especially hesperidin nanoemulsion induces apoptosis in prostate cancer cells. Park et al also stated in 2008 that hesperidin increases apoptosis and decreases the proliferation of colon cancer cells, SNU-C4 cell line [46].
Figure 14. Fluorescence images of LNCaP cells treated with IC50 concentration of hesperidin nanoemulsions and free hesperidin (scale bar 50 μm, magnification 400X) (a); LNCaP cells without treatment (b); cells treated with IC50 concentration of free hesperidin (c); cells treated with IC50 concentration of hesperidin nanoemulsions.
Download figure:
Standard image High-resolution image4. Conclusion
In this research, we successfully synthesized hesperidin nanoemulsion with favorable physicochemical properties using a straightforward and low-energy method. Subsequently, we evaluated its anticancer effects on the prostate cancer cell line (LNcap). The results from MTT, NR, TB, and LDH tests indicated the remarkably high cytotoxic effects of hesperidin nanoemulsion on the prostate cancer cell line. Further validation through cell morphology evaluation and a colony formation assay corroborated these findings. Remarkably, hesperidin nanoemulsion exhibited not only significantly stronger anticancer activity compared to free hesperidin but also displayed high biocompatibility with minimal cytotoxic effects on healthy cells. It is well-established that for a compound to be considered for cancer treatment, must exhibit toxicity towards cancer cells while being biocompatible with healthy cells—a criterion met by the hesperidin nanoemulsion synthesized in this study. In conclusion, the successful synthesis of hesperidin nanoemulsion highlights its potential utility alongside chemotherapy drugs to enhance efficacy while mitigating associated side effects in prostate cancer treatment
Acknowledgments
The authors are grateful for the support provided by Urmia University.
Data availability statement
All data that support the findings of this study are included within the article (and any supplementary files).
Funding
This research did not receive any specific Grant from funding agencies in the public, commercial, or not-for-profit sectors.
Conflict of interest
The authors declare that there are no conflicts of interest.
Compliance with ethics requirements
The execution of all procedures adhered to ethical standards, as approved by the responsible committee on human experimentation, and complied with the Helsinki Declaration.