Abstract
Electrospun nonwovens have been used extensively for tissue engineering applications due to their inherent similarities with respect to fibre size and morphology to that of native extracellular matrix (ECM). However, fabrication of large scaffold constructs is time consuming, may require harsh organic solvents, and often results in mechanical properties inferior to the tissue being treated. In order to translate nonwoven based tissue engineering scaffold strategies to clinical use, a high throughput, repeatable, scalable, and economic manufacturing process is needed. We suggest that nonwoven industry standard high throughput manufacturing techniques (meltblowing, spunbond, and carding) can meet this need. In this study, meltblown, spunbond and carded poly(lactic acid) (PLA) nonwovens were evaluated as tissue engineering scaffolds using human adipose derived stem cells (hASC) and compared to electrospun nonwovens. Scaffolds were seeded with hASC and viability, proliferation, and differentiation were evaluated over the course of 3 weeks. We found that nonwovens manufactured via these industry standard, commercially relevant manufacturing techniques were capable of supporting hASC attachment, proliferation, and both adipogenic and osteogenic differentiation of hASC, making them promising candidates for commercialization and translation of nonwoven scaffold based tissue engineering strategies.
Export citation and abstract BibTeX RIS
For more information on this article, see medicalphysicsweb.org
1. Introduction
Tissue engineering strategies aim to repair or replace damaged tissues using a stem cell source seeded on a biological or artificial scaffold structure. As the cells proliferate and differentiate creating new tissue, the original scaffolding material is gradually replaced, leaving behind the desired replacement tissue. A wide range of scaffolding materials have been investigated as tissue engineering constructs with varying degrees of success [1]. Nonwoven fabrics are one such example that show great promise as scaffolding for tissue engineering [2–12]. In basic terms, a nonwoven is a fabric or web composed of fibres. The orientation and properties of the fibres and fabric can be controlled to mimic native cellular structures, such as collagen fibres of the extracellular matrix (ECM). Electrospinning is the most common nonwoven manufacturing method used in the field of tissue engineering. The size scale of electrospun fibres mimics that of native ECM and provides an ideal environment for cellular attachment, growth, and differentiation into the target tissue [13–22]. However one of the many hurdles facing translation of tissue engineering strategies to clinical practice lies in scalable and repeatable fabrication of scaffold material on an industrial scale. Fabrication of large scale electrospun scaffolds is time consuming, may require harsh organic solvents, is difficult to generate sufficient 3D structures, and typically results in mechanical properties inferior to the target tissue to be replaced [23–25]. In order to translate nonwoven tissue engineering from the lab to the clinic, a scalable, repeatable, and economical scaffold fabrication method is needed. The goal of this study was to test our hypothesis that other industry standard nonwoven manufacturing technologies may meet this need.
Although there are many nonwoven fabrication processes, three of the most common in use are meltblowing, spunbond, and carding. These processes and the processing parameters affecting fabrication and properties of the resulting nonwovens are described in detail elsewhere [26], but are briefly described here to orient the reader. Meltblowing and spunbond nonwovens are composed of continuous fibre filaments fabricated by forcing molten thermoplastic polymer through very fine orifices arranged in a spinbeam. As the filaments are formed, they are drawn and accelerated, and collected on a moving screen or belt to form the nonwoven web. The main difference in the meltblowing and spunbond processes lies in the drawing step. In the meltblowing process, the filaments are drawn and accelerated toward the collector screen via hot air knives, keeping the filaments in a molten state, allowing for fine fibre attenuation. Collected fibres are still in a tacky state, allowing self-bonding between fibres [26]. Conversely, in the spunbond process, as the filaments exit the spinbeam they are rapidly solidified by cool air before being drawn pneumatically. Drawing of filaments in the solid state increases molecular orientation and leads to improved mechanical properties compared to meltblowing, typically at the cost of greater fibre diameter [27, 28]. From a raw material standpoint, theoretically any thermoplastic polymer is suitable for spunbond and meltblown processing. In practice, the ability to form continuous filaments is dictated by the processing conditions and the material properties of the raw polymer. Polymers suitable for spunbond production typically have a melt flow index (MFI) of 5 to 40 dg min−1 [29]. In the meltblowing process, low viscosity polymers (MFI of greater than 1000) are typically required due to greater pressure drop and decreased spinning orifice diameters [30]. Spunbond and meltblowing techniques can be implemented from lab, pilot, and full production scale. Simulated spunbond methods allow for testing at the lab scale before implementing pilot studies [27]. Similarly, meltblown dies as small as 13 cm [31] up to full production scale of 6 m allow for scalability throughout the design process [32].
In the carding process, short fibres of a few inches in length (staple fibres) are separated and entangled by a series of specialized combed rollers to form an unbonded web. Unlike spunbond and meltblown processing that require a thermoplastic polymer for meltspinning, a variety of fibre materials can be processed via carding technology, including natural and synthetic polymers, glass, and metal fibres [33]. Carded webs are typically layered to increase the fabric basis weight and improve fibre orientation (crosslapping) before being bonded to lock the structure in place [26]. As with spunbond and meltblowing processes, a variety of carding configurations are available ranging from small model carding units to full-scale production lines. Typical processing capabilities and fibre and fabric properties achievable via the methods described here are presented in table 1. The high speed, repeatable, economical production capabilities of these techniques make them attractive candidates for the commercial production of nonwoven based tissue engineering scaffolding materials.
Table 1. Estimated typical processing capabilities and cost of commercial nonwoven manufacturing techniques.
Electrospinning [34] | Meltblowing [32] | Spunbond [32] | Carding [33] | |
---|---|---|---|---|
Max line speed (m min−1) | 20 | 300 | 600 | 200 |
Max line width (m) | 1.6 | 6 | 6 | 5.5 |
Fiber diameter range (μm) | 0.08–0.7 | 0.5–10 | 10–50 | 10–50 |
Basis weight range (g m−2) | 0.02–8 | 5–500 | 10–800 | 10–2000 (with crosslap) |
Typical cost for PLA ($ m−2) | 2–5 | 1–2 | 0.3–3 | 0.1–3 |
Interest in human adipose derived stem cells (hASC) for tissue engineering applications has increased recently given their relative ease of harvest, accessibility, and multipotent differentiation capacity, in particular for lineages derived from the mesoderm [35–40]. Ease of harvest from excessive adipose tissue makes them particularly attractive compared to other mesodermal cells such as mesenchymal stem cells that require invasive collection procedures and yield smaller quantities. The goal of this study was to compare industry standard nonwovens fabricated via meltblowing, spunbond, and carding to electrospun materials as tissue engineering scaffolds with respect to their effects on viability, proliferation, and differentiation of hASC.
2. Methods
2.1. Scaffold fabrication
All nonwoven scaffolds used in this study were composed of poly(lactic acid) (PLA) 6202D (NatureWorks LLC, Minnetonka, MN), with a melt index (g/10 min at 210 °C) of 15–30, engineered for staple fibre and spunbond production. Spunbond, carded, and meltblown nonwovens were fabricated at the Nonwovens Institute pilot facilities (NWI, Raleigh, NC). Meltblown processing of this PLA grade was achieved via a 5 inch Biax Fibre-Film (Greenville, WI) co-concentric spinnerette mounted on a custom meltblowing unit. Electrospun scaffolds were produced by dissolving PLA in chloroform and dimethylformamide at a ratio of 3 : 1 (Sigma, St Louis, MO) at a concentration of 11 wt% for 4 h at 80 °C. Electrospinning was performed at room temperature at a feed rate of 0.7 μl h−1, 15 kV, and spinning distance of 15 cm on a static grounded collector for 3 h on a custom electrospinning system.
2.2. Scaffold characterization
Mechanical and physical properties of nonwovens used in this study were characterized prior to cell seeding. Fabric surface and fibre morphology was imaged on a Phenom G2 Pro scanning electron microscope (SEM) (Eindhoven, Netherlands). Fibre diameter was calculated using ImageJ software (NIH, Bethesda, MD) with a minimum of 100 fibres measured. Fabric thickness was measured using a Hanatek FT3 Precision Thickness Gauge (East Sussex, UK). Thickness measurements were taken from random locations across fabric surfaces, at least two inches from the fabric edge (n = 10) in accordance with ASTM D5729. Mean flow pore size was analyzed using a Porous Materials INC advanced capillary flow porometer (Ithaca, NY) using GalWick as the wetting agent in a wet up/dry up, no wait at dry configuration. Basis weight samples of each fabric type were cut and weighed in accordance with IST 130.2. The solid volume fraction (SVF) was calculated from thickness and basis weight measurements and fibre density (1.24 g cm−3) according to equation (1). Fabric porosity is reported as the difference of 100% and the SVF.
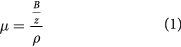
where: μ = solid volume fraction
B = fabric basis weight
z = fabric thickness
ρ = fibre mass density
A strip test in the machine direction (MD) and cross direction (CD) was used to evaluate mechanical properties measured on an Instron 4400R (Norwood, MA) in accordance with ASTM D5035. Fabric width was insufficient for strip test in the CD for meltblown fabrics (less than 6 inches) and both MD and CD for the electrospun fabrics.
2.3. hASC isolation
A pre-menopausal hASC superlot that we have previously described, consisting of five gender and age matched donors, was used for cellular growth and differentiation studies [40]. Use of a superlot allows streamlining of the experimental process, reducing time and reagents required to test individual donor cells, while capturing cellular proliferation and differentiation behaviours of the individual cell lines [40]. hASC were obtained from excess waste tissue from liposuction procedures performed at the University of North Carolina at Chapel Hill under an IRB exempt protocol (10–0201).
2.4. Scaffold seeding
Fabrics were punched into 9/16 inch diameter circular scaffolds and placed in 24 well non-tissue culture treated polystyrene plates and sterilized in 70% ethanol for 4 h and subsequently washed with phosphate buffered saline (PBS) three times. Scaffolds were then soaked in complete growth medium (CGM; Eagle α-Minimum Essential Medium, 10% fetal bovine serum (FBS), 2 mM L-glutamine, 100 units ml−1 penicillin, and 100 μg ml−1 streptomycin) for 12 h prior to cell seeding. hASC superlot (second passage) cells were seeded in 75 cm2 tissue culture treated flasks and expanded to 75% confluency in CGM. hASC were trypsinized and resuspended in CGM at a concentration of 200 000 cells ml−1. Scaffolds (n = 3/condition) were then seeded with 20 000 cells cm−2 and allowed to attach for 1 h in a humidified incubator at 37 °C and 5% CO2 prior to flooding the well with CGM. Cells were allowed to attach for an additional 12 h prior to turning the scaffolds over and seeding the other side with an additional 20 000 cells cm−2. After an additional 12 h, scaffolds were transferred to new tissue culture plates, and the remaining cells on the plate surfaces were trypsinized and counted on a hemocytometer to determine cell seeding efficiency. Scaffolds were then cultured in CGM for 7 d, with media changes performed every 3 d. On day seven, culture medium was changed to osteogenic differentiation medium (ODM; Eagle α-Minimum Essential Medium, 10% FBS, 2 mM L-glutamine, 100 units ml−1 penicillin, 100 μg ml−1 streptomycin, 50 μM ascorbic acid, 0.1 μM dexamethasone, and 10 mM β-glycerophosphate), or adipogenic differentiation medium (ADM; Eagle α-Minimum Essential Medium, 10% FBS, 2 mM L-glutamine, 100 units ml−1 penicillin, 1 μM dexamethasone, 10 μg ml−1 human insulin, 100 μM indomethacin, and 500 μM isobutylmethlxanthine), or continued in CGM for an additional 14 d with media changes every 3 d.
2.5. Analysis of scaffold performance
On days 1, 3, 5, 7, and 21 cellular viability was evaluated with a mammalian cell Live/Dead viability/cytotoxicity kit (Invitrogen Molecular Probes, Eugene, OR) and cellular proliferation using the AlamarBlue™ assay (BioRad AbD Serotec, Oxford, UK). Live/Dead scaffolds (n = 3/condition/time point) were washed twice in PBS and dead cells stained with 4 μM ethidium homodimer-1 and live cells with 2 μM calcein AM in PBS for 15 min in a humidified incubator at 37 °C and 5% CO2. Stained scaffolds were then mounted on glass microscope slides and imaged under 10 × magnification on a Leica DM5500B fluorescence microscope (Leica Microsystems, Wetzlar, Germany). Calcein AM produces a strong green fluorescence in the cytoplasm of live cells while ethidium homodimer-1 produces a strong red fluorescence in the nuclei of dead cells. At each experimental time point, AlamarBlue™ scaffolds (n = 3/condition) were washed twice with PBS and wells flooded with media containing 10% (v/v) of AlamarBlue™. Acellular blank scaffolds treated with appropriate medium were included at each time point. Scaffolds were incubated for 3 h in a humidified incubator at 37 °C and 5% CO2, at which time 200 μl of treated media was removed and absorbance read at 570 and 600 nm on a GENios microplate reader (Tecan, Männedorf, Switzerland). Scaffolds were then washed three times with PBS and wells flooded with media. Metabolic activity results in the reduction of AlamarBlue™, resulting in a detectable colour change. The percent reduction is related to metabolic activity and thus an indicator of cellular proliferation. Because AlamarBlue™ is nontoxic to cells, the same scaffolds can be evaluated for cellular proliferation for the duration of the experiment. AlamarBlue™ assays were conducted in the culture wells for each time point analyzed to minimize disturbing hASC attached to the scaffolds.
Cell mediated calcium and lipid accretion was evaluated on day 21 qualitatively using Alizarin Red S and Oil Red O stains, respectively (both reagents from Acros Organics, Geel, Belgium), and quantitatively using total calcium (StanBio Laboratory, Boerne, TX) and adipogenesis colourimetric assay kits (BioVision, Milpitas, CA). Scaffolds for staining were washed three times in PBS and fixed in 10% buffered formalin for 30 min (Thermo Scientific, Waltham, MA). Scaffolds were subsequently rinsed three times in PBS and stained with 40 mM Alizarin Red S or 0.4 mM Oil Red O for 5 min. Scaffolds were rinsed several times with distilled water to remove excess stain and imaged on an Olympus CKX41 inverted microscope at 10 × magnification (Olympus, Center Valley, PA). Scaffolds for quantitative analysis (n = 3/condition) were rinsed twice in PBS and subsequently carefully cut in half with a razor. For calcium quantification one half was transferred to 1 ml of 0.5 N HCl and placed on an orbital shaker overnight at 4 °C. The remaining half was transferred to 200 μl of RIPA buffer (Thermo Scientific, Waltham, MA) and incubated on ice for 10 min, centrifuged at 15 000 rpm and the supernatant collected. Similarly, scaffolds for triglyceride quantification were cut in half and one half transferred to 200 μl of adipogenesis assay buffer and the remaining half in RIPA buffer. Calcium and triglyceride samples were assayed according to manufacturer's instructions and absorbance read on a GENios microplate reader at 550 and 570 nm, respectively. Protein samples for normalization were analyzed using a BCA total protein assay (Thermo Scientific, Waltham, MA) according to manufacturer's instructions and absorbance read at 562 nm.
2.6. Statistical analysis
Statistical analysis was performed using Microsoft Excel for Mac OS X, Version 10.10.2 (Microsoft, Redmond, WA). An unpaired Student's t-test was used to analyze statistical difference and to distinguish statistical significance between test conditions. For each quantitative assay n = 3 biological replicates and n = 3 technical replicates were utilized (n = 9 per condition). Error bars represent standard error of the mean. Statistical significance is indicated as p < 0.05.
3. Results
3.1. Scaffold characterization
The nonwoven fabrics employed in this study as tissue engineering scaffolds were chosen as representative 'typical' nonwovens with 'typical' fabric and fibre properties of their respective manufactuing method. Scaffolds exhibited a wide range of fibre and fabric properties (table 2). Representative macroscopic images of scaffolds are presented in figure 1, and SEM micrographs of scaffold surfaces at 500 × magnification are presented in figure 2. Scaffolds varied from thin fabrics with fine micron sized fibres and small pore size to very open structures with very high porosity and larger fibres. Fibre diameter varied from 1.1–26.9 μm, while thickness and pore size ranged from 160.8–965.7 μm and 2.6–655 μm, respectively. Similarly, mechanical properties, measured as peak load, varied from 1.0–102.2 N. As expected, sample size of electrospun scaffolds was insufficient for mechanical testing in the MD and CD, and the width of meltblown samples was insufficient for CD testing.
Table 2. Fiber and fabric properties of nonwovens evaluated as tissue engineering scaffolds using hASC.
Electrospun | Meltblown | Spunbond | Carded | |
---|---|---|---|---|
Fiber diameter (μm) | 1.1 ± 0.3 | 4.8 ± 4.6 | 12.8 ± 2.4 | 26.9 ± 3.0 |
Fabric thickness (μm) | 160.8 ± 1.4 | 343.2 ± 12.3 | 562.3 ± 21.2 | 965.7 ± 52.2 |
Mean flow pore size (μm) | 2.6 ± 0.7 | 27.2 ± 12.5 | 24.0 ± 14.9 | 655.0 ± 373.4 |
Basis weight (g m−2) | 25 | 50 | 100 | 55 |
SVF | 0.125 | 0.117 | 0.143 | 0.046 |
Porosity (%) | 87.5 | 88.3 | 85.7 | 95.4 |
MD strip test peak load (N) | — | 25.5 ± 7.4 | 102.2 ± 12.8 | 1.0 ± 0.6 |
CD strip test peak load (N) | — | — | 55.7 ± 5.5 | 1.0 ± 0.6 |
Note: MD and CD refer to machine and cross directions, respectively. Fabric size was insufficient for peak load testing of electrospun scaffolds and fabric width was insufficient for meltblown CD peak load testing.
Figure 1. Optical images of nonwoven fabrics evaluated as tissue engineering scaffolds for hASC (Canon EOS Rebel T3i 18-55 mm automatic lens). (a) Electrospun, (b) meltblown, (c) spunbond, and (d) carded. CD and MD indicate cross and machine directions, respectively. MD and CD do not apply to electrospun scaffolds, as they were collected on a static plate.
Download figure:
Standard image High-resolution imageFigure 2. Scanning electron micrographs of nonwoven fabric surfaces evaluated as tissue engineering scaffolds for hASC at 500× magnification. (a) Electrospun, (b) meltblown, (c) spunbond, and (d) carded. Fiber diameters ranged from 1.1, 4.8, 12.8, and 26.9 μm while average pore size ranged from 2.6, 27.2, 24.0, and 655 μm for electrospun, meltblown, spunbond, and carded fabrics, respectively. Scale bars represent 100 μm.
Download figure:
Standard image High-resolution image3.2. Seeding efficiency
hASC were able to adhere to all scaffolds examined with seeding efficiencies exceeding 60% for all nonwoven fabric types (figure 3). Scaffolds exhibited seeding efficiencies of 84, 82, 78, and 64% for electrospun, meltblown, spunbond, and carded fabrics, respectively. There was no significant difference in seeding efficiency between electrospun, meltblown, and spunbond scaffolds. However, carded nonwovens exhibited a significant decrease in seeding efficiency compared to the other scaffold types (figure 3). After transferring the scaffolds to new culture plates, the plates used for the seeding process were visually inspected under an optical microscope prior to calculation of seeding efficiency. For each scaffold type, the majority of cells attached to the culture plate were concentrated on the edge of the culture well, in the small space between the scaffold and the plate wall where the cells did not have to travel through the scaffold to reach the plate. The electrospun scaffold plates showed little to no cells in the area covered by the scaffolds. The meltblown and spunbond scaffold plates exhibited a small number of cells in the area covered by the scaffolds, while the carded plates exhibited the greatest number of cells in the area under the scaffolds.
Figure 3. Seeding efficiency of nonwoven scaffolds evaluated as tissue engineering scaffolds for hASC. After the seeding procedure, scaffolds were transferred to new tissue culture plates and the remaining cells were trypsinized and counted on a hemocytometer (n = 3). No statistical differences were observed among electrospun, meltblown, and spunbond scaffolds, while carded scaffolds exhibited a significant reduction in seeding efficiency. This was attributed to much larger fibre and fabric pore sizes in carded scaffolds, reducing overall surface area and probability of cells interacting with scaffold fibres. Error bars represent standard error of the mean. * indicates p < 0.05.
Download figure:
Standard image High-resolution image3.3. Proliferation and viability
hASC proliferation and viability was analyzed qualitatively over the course of the experiment using a Live/Dead staining assay. All scaffolds exhibited an increase in the number of viable cells over the first 7 days of culture in CGM (figure 4). hASC on electrospun, meltblown, and spunbond scaffolds exhibited a typical hASC morphology and attached across multiple PLA fibres. However, hASC cultured on carded samples initially attached to single PLA fibres and exhibited a more elongated morphology along the fibre direction (figures 4(d1) and (d2)). After 5 days, cells appeared to be concentrated in regions where fibres overlap, with far fewer cells in adjacent open pore volumes (figures 4(d3) and (d4)). At the end of the 21 day experiment, electrospun, meltblown, and carded scaffolds were uniformly covered in viable cells for all culture media (CGM, ODM, and ADM), whereas carded samples showed regions of concentrated cells in regions of fibre overlap, with ingrowth into adjacent pore volumes (figure 5).
Figure 4. Live/dead staining of hASC over time for hASC seeded scaffolds cultured in CGM at 10× magnification. All fabric types supported viable cell growth over the first 7 days of the experiment. Meltblown and spunbond samples exhibited a greater number of viable cells, attributed to cell attachment and spreading across multiple fibres throughout the thickness of the scaffolds. Electrospun scaffolds exhibited cell growth in a monolayer on the fabric surface. Cells initially attached to single fibres on carded scaffolds and gradually filled in at fibre–fibre crossover points. Meltblown and spunbond scaffolds also exhibited a greater number of dead cells compared to electrospun and carded fabrics. This is attributed to mass transport limitations in cells that have infiltrated the fabric thickness. Scale bars represent 100 μm.
Download figure:
Standard image High-resolution imageFigure 5. Live/dead staining of hASC at the end of the 21 d experiment in CGM, ODM, and ADM at 10× magnification. hASC remained viable on all fabric types for the duration of the experiment. By day 21, electrospun, meltblown, and spunbond fabrics exhibited similar cell spreading, covering the entire surface of the fabrics. hASC on carded scaffolds showed ingrowth into pore volumes, originating from fiber–fiber crossover points, but did not entirely occupy scaffold pores. hASC treated with CGM or ODM exhibited typical spread hASC morphology while ADM treated scaffolds assumed a rounded shape. Electrospun, meltblown, and spunbond scaffolds exhibited a similar number of dead cells, while carded scaffolds exhibited relatively few dead cells. Scale bars represent 100 μm.
Download figure:
Standard image High-resolution imageProliferation of hASC was quantified using the AlamarBlue™ assay. All scaffolds exhibited an increase in hASC proliferation over time, however the meltblown and spunbond scaffolds led to a significant increase in cellular proliferation compared to electrospun and carded scaffolds over the first 7 days in culture (figure 6(a)). These results were in agreement with the qualitative Live/Dead imaging. At the end of the 21 day experiment in each culture media evaluated, there were no significant differences in proliferation between scaffold types (figure 6(b)).
Figure 6. AlamarBlue™ cellular proliferation of hASC across different nonwoven fabric types (n = 3). (a) hASC proliferated across all fabric types over the first 7 days of the experiment, however meltblown and spunbond fabrics showed a significant increase compared to electrospun and carded fabrics. (b) All scaffold types showed similar proliferation at the end of the 21 day experiment across each media type evaluated. Error bars represent standard error of the mean. * indicates statistical difference between scaffold types connected by bars (p < 0.05).
Download figure:
Standard image High-resolution image3.4. Differentiation capacity
In order to examine the effect of scaffold type on the adipogenic and osteogenic differentiation capacity of hASC, lipid and calcium accretion was visualized and quantified on day 21. Oil Red O staining revealed the presence of lipid vacuoles in hASC on all scaffold types when cultured in ADM (figure 7(a3), (b3), (c3) and (d3)). Similarly, Alizarin Red S staining indicated the presence of calcium accretion for all scaffolds cultured in ODM (figure 8(a2), (b2), (c2) and (d2)). These results were confirmed quantitatively and indicated a significant increase in lipid and calcium accretion for all scaffolds compared to controls (figure 9). Electrospun, meltblown, spunbond, and carded scaffolds resulted in normalized triglyceride concentrations of 0.048 ± 0.007, 0.074 ± 0.013, 0.069 ± 0.010, and 0.077 ± 0.005 nmol lipids μg−1 protein respectively, and calcium mass of 0.0095 ± 0.0006, 0.0099 ± 0.0009, 0.0074 ± 0.0006, and 0.0108 ± 0.0006 μg calcium μg−1 protein respectively. As expected, lipid accretion was significantly lower for CGM and ODM treated scaffolds, and calcium accretion was significantly lower for CGM and ADM treated scaffolds.
Figure 7. Oil Red O lipid staining for all nonwoven fabric types evaluated as tissue engineering scaffolds for hASC at 10× magnification. All scaffold types exhibited the presence of lipid vacuoles when treated with ADM (lipid vacuoles appear stained as cherry red spheres). Electrospun scaffolds exhibited a monolayer of lipid vacuoles in a single focal plane, whereas lipid vacuoles where observed in multiple planes throughout the fabric thickness for meltblown, spunbond, and carded scaffolds. CGM controls and ODM treated scaffolds did not exhibit any lipid vacuoles, indicating cells were not undergoing random differentiation. Scale bars represent 100 μm.
Download figure:
Standard image High-resolution imageFigure 8. Alizarin Red S calcium staining for all nonwoven fabrics types evaluated as tissue engineering scaffolds for hASC at 10× magnification. Calcium deposits appear dark red after staining. All fabric types exhibited the presence of intense calcium staining when treated with ODM. Calcium staining was concentrated in regions of greatest fibre density for meltblown, spunbond, and carded scaffolds, while electrospun scaffolds exhibited uniform staining over the entire fabric surface. CGM controls did not exhibit any calcium staining, however relatively small amounts of calcium were observed for ADM treated scaffolds. Scale bars represent 100 μm.
Download figure:
Standard image High-resolution imageFigure 9. End product expression of hASC after 21 days in culture on all nonwoven fabric types evaluated (n = 3). (a) Total triglyceride content normalized to total protein across all media types evaluated. All fabric types exhibited a significant increase in triglyceride content when treated with ADM, indicating the presence of an adipogenic cell phenotype. No statistical differences were observed amongst ADM treated scaffolds across all fabric types. (b) Calcium content normalized to total protein. All fabric types exhibited a significant increase in calcium content when treated with ODM, indicating the presence of an osteogenic cell phenotype. No statistical differences were observed amongst ODM treated scaffolds between electrospun, meltblown, and carded scaffolds, however a small but significant decrease was observed on the spunbond scaffolds. CGM = complete growth medium. Error bars represent standard error of the mean. * indicates statistical difference compared to control mediums (p < 0.05). NS indicates not statistically significant between scaffold types connected by bars.
Download figure:
Standard image High-resolution image4. Discussion
The goal of this study was to evaluate commercially relevant, repeatable, and economical high throughput nonwoven manufacturing techniques as tissue engineering scaffolds. Specifically, we examined the viability, proliferation, and adipogenic or osteogenic differentiation of hASC on meltblown, spunbond, and carded PLA nonwovens compared to the nonwoven gold standard of electrospinning for tissue engineering. Our group and many others have demonstrated the potential utility of electrospun nonwovens as tissue engineering scaffolds for a wide range of cell sources and target tissues [13–17, 20–22]. Electrospun fibres are similar in size scale to native ECM and provide an ideal environment for cellular attachment, proliferation, and differentiation. However, electrospinning large quantities is time consuming, may require harsh organic solvents, and typically results in structures with inferior mechanical properties to the tissue being treated [23–25]. We hypothesized that industry standard nonwoven manufacturing techniques such as meltblowing, spunbond, and carding may provide economical, repeatable, and scalable methods for the fabrication of large quantities of nonwovens for use as tissue engineering scaffolds with precise control of fabric and fibre properties.
All scaffolds used in this study were able to support attachment, proliferation, and differentiation of hASC. Seeding efficiency data indicated there was no significant difference between seeding efficiency for electrospun, meltblown, and spunbond scaffolds, however a significant decrease was observed on the carded scaffolds (figure 3). This is likely due to differences in fabric properties, namely pore size and fibre size. The carded scaffold had a very open and porous structure; with an average pore size of 655 μm, compared to pore sizes in the range of 1–25 μm for the other fabric types. The fibre diameter of 26.9 μm was also significantly larger for the carded scaffolds, compared to fibre diameters ranging from 1.1–12.8 μm for the other scaffolds. This open structure and larger fibre size may have decreased the opportunity for cellular attachment to the scaffold fibres during the seeding process as hASC seeded in static culture could instead collect and adhere to the culture plate.
Live/Dead staining revealed that cells remained viable on all nonwoven scaffold types over the course of 3 weeks (figures 4 and 5). Further, by the end of the experiment all scaffolds across all media types appeared to exhibit similar hASC coverage on all nonwoven types (figure 5). However, at early time points, meltblown and spunbond samples appeared to have more viable cells compared to electrospun and carded fabrics (figure 4(b1)–(b4) and (c1)–(c4)). This was consistent with AlamarBlue™ cellular proliferation assays, which showed a statistically significant increase in hASC proliferation on meltblown and spunbond scaffolds for days 1–7 compared to electrospun and carded nonwovens (figure 6(a)). We speculate that this increase is likely attributed to cellular infiltration into the thickness of meltblown and spunbond scaffolds. Cellular growth is restricted to the surface of electrospun fabrics due to very small pore sizes, effectively leading to less total growth area for cells. Carded samples also indicated cell spreading throughout the thickness of the scaffolds, however cellular attachment was focused on the surface of single fibres, whereas cells appeared to attach across multiple fibres on the other fabric types. Cell growth on carded samples began with attachment of individual cells to single fibres, and over time cells populated fibre–fibre cross over points (figures 4(d1)–(d4) and 5(d1)–(d3)). These findings are consistent with work by Edwards et al examining tissue growth on carded PET nonwovens using mouse fibroblasts [6]. Meltblown and spunbond scaffolds also exhibited a greater number of dead cells over the course of the experiment compared to electrospun and carded fabrics (figures 4(b1)–(b4), (c1)–(c4) and 5(b1)–(b3), (c1)–(c3)). This was likely attributed to mass transport limitations of nutrients throughout the thickness of the scaffold constructs. Several groups have shown that cell seeded scaffolds quickly become covered in a dense layer of ECM and cells on the periphery, effectively blocking mass transport of nutrients to cells in the interior of the scaffold and removal of cellular waste products [7, 8, 41, 42]. In the case of electrospun scaffolds, cell growth is restricted to the surface of the fabric due to the small pore size restricting migration through the fabric thickness, however at the end of the 21 day experiment electrospun scaffolds showed an increase in the number of dead cells compared to earlier time points. This effect was not observed with the carded scaffolds and is likely a result of the very porous nature of the material. hASC are not observed to occupy the entirety of the pore volume, likely resulting in less ECM deposition on the surface, effectively retaining a degree of porosity and route of mass transport.
AlamarBlue™ proliferation data at day 21 indicated minimal differences in the number of cells across all fabric and media types (figure 6(b)). Although the initial proliferation rate of the meltblown and spunbond samples was higher compared to those of the electrospun and carded samples (figure 6(a)), by the end of the experiment cell growth equalized across the different scaffold types (figure 6(b)). Taken together, this data suggests that there is an important relationship between fibre size, pore size, and fabric thickness that governs the ability of cells to attach and proliferate throughout the scaffold construct over time. Although AlamarBlue™ is widely accepted as an indicator for proliferation [43–47], it is more accurately a measure of overall metabolic activity [43]. Therefore, direct comparison of different phenotypes presents inherent limitations of this technique. Adipocytes exhibit a lower level of overall metabolic activity compared to that of osteoblasts, which would result in a lower percent reduction of AlamarBlue™ in adipocytes compared to the same number of osteoblasts, suggesting a lower level of proliferation. Experiments to calculate the actual number of cells at each time point, such as measuring total deoxyribonucleic acid (DNA), would be needed to conclusively determine the rate of proliferation.
Analysis of the differentiation capacity of hASC cultured on high throughput nonwoven fabrics indicated that all fabrics were capable of supporting adipogenesis and osteogenesis when treated with appropriate growth factors (figures 7–9). Oil Red O staining revealed the presence of lipid vacuoles for hASC seeded on all scaffold types cultured in ADM (figure 7(a3), (b3) and (c3)). Visual inspection of Oil Red O micrographs would suggest that electrospun scaffolds exhibit the largest number of lipids compared to the other scaffold types. However, all of the lipid vacuoles on the electrospun scaffolds were observed in a single plane, suggesting cell growth was restricted to the surface of the scaffold with little to no ingrowth throughout the fabric thickness (figure 7(a3)). Conversely, all other scaffolds exhibited the presence of lipid vacuoles in multiple planes, throughout the thickness of the fabrics. Although not a significant measure of cellular infiltration, this suggests that cells are capable of penetrating the thickness of meltblown, spunbond, and carded scaffolds to some extent. Additional experiments would be required to verify cellular infiltration and distribution throughout the thickness of the scaffolds. Additionally, lipid vacuoles on the carded samples appeared concentrated in the space between fibre–fibre crossover points (figure 7(d3)), further suggesting cells are able to bridge these points after 3 weeks, similar to work by Edwards et al on carded PET [6]. Oil Red O staining results were confirmed with quantitative evaluation of total triglycerides present on ADM treated scaffolds. Figure 9(a) revealed that all hASC seeded scaffolds cultured in ADM show a striking increase in total triglycerides compared to CGM controls and ODM treated scaffolds, indicating the presence of an adipogenic phenotype. Further, there was no statistical difference in normalized triglyceride content for ADM treated scaffolds between nonwoven fabrication method, indicating all three high throughput fabrication methods are suitable for hASC adipogenesis.
Similar to Oil Red O results, Alizarin Red S staining revealed the presence of intense red calcium staining across all nonwoven scaffold types seeded with hASC and cultured in ODM (figure 8(a2), (b2), (c2) and (d2)). Calcium staining was not observed for CGM controls; however there was a small amount of staining for ADM treated scaffolds (figure 8(a3), (b3), (c3) and (d3)). Microscopic evaluation revealed that calcium staining was concentrated in areas of greatest fibre density, i.e. staining was not present in porous regions. This suggests that cells are also concentrated in these regions and do not fully occupy pore volume. This was particularly striking for the carded samples, where red staining was observed near fibre cross over points, leaving no stain in the large open pores. Small regions of light staining were also observed in ADM treated scaffolds with no apparent pattern discernable.
Alizarin Red S staining results were confirmed with quantitative evaluation of total calcium present on each scaffold type. Figure 9(b) revealed that all ODM treated fabric types showed an increase in total calcium compared to CGM controls and ADM treated scaffolds, indicating the presence of an osteogenic phenotype. ADM treated scaffolds showed an increase in calcium content relative to CGM controls, however to a much lesser extent than ODM treated scaffolds. While there was no difference in scaffold type for adipogenesis, osteogenesis results indicated that there was no difference in normalized calcium accretion across electrospun, meltblown, and carded samples, however there was a small but significant decrease in calcium accretion on spunbond scaffolds.
Scaffolds used in this study were sterilized using ethanol submersion and no contamination was observed throughout the duration of the culture period. While this sterilization technique is effective and easily implemented at the lab scale, it is not conducive to full scale commercial sterilization. Economical and effective sterilization of scaffolds on an industrial scale must also be considered when considering nonwoven scaffolds as commercial tissue engineering products. Ethylene oxide sterilization and γ-irradiation are the two main sterilization techniques currently employed in the medical device field [48]. γ-irradiation has become the industry standard for sterilization of nonwoven medical materials due to its effectiveness, low-cost, and ease of use [49]. Further, ethylene oxide sterilization may lead to the formation of toxic gases, posing a health risk [49]. However, when choosing a sterilization method, impact on the material and chemical properties of the device must be considered. γ-irradiation has been shown to lead to deterioration of mechanical properties of polypropylene and PLA via chain scission [48, 49]. Careful evaluation of the effects of sterilization on the properties of nonwoven scaffolds must be considered and deemed acceptable for successful implementation in a final product.
5. Conclusions
We have demonstrated that meltblown, spunbond, and carded high throughput nonwoven manufacturing methods are suitable for production of tissue engineering scaffolds for hASC. hASC viability, proliferation, adipogenesis, and osteogenesis were similar to electrospun gold standard nonwoven tissue engineering scaffolds. These results are promising in the effort to move tissue engineering strategies out of the lab and into commercial production and clinical use as they allow large quantities of material to be produced quickly, economically, and with a wide range of controlled fabric properties. However, it is important to note that further studies are needed to understand the interplay of fabric properties and their effects on cellular growth and differentiation. In order to directly state that one method is superior to another, studies normalizing specific fabric properties such as fibre size, SVF, or pore size are needed. This becomes difficult, as each method has unique benefits and disadvantages. For example, it would be nearly impossible to create electrospun and carded scaffolds with the same fibre diameter and porosity. However these results do indicate that these methods are suitable for cell growth and differentiation and merit additional study as commercially viable, high throughput tissue engineering scaffolding materials. Understanding the interplay between fibre and fabric properties on the behaviour of hASC is crucial for successful tissue engineering strategies and will vary depending on the target tissue and cell source. In future studies, we will examine the effects of processing parameters as well as fibre and fabric properties on cellular growth and differentiation across different nonwoven manufacturing methods with the goal of better understanding the relationship between scaffold properties and hASC behaviour.
Acknowledgments
The authors would like to thank Dr Mahsa Mohiti-Asli for fabrication of the electrospun scaffolds used in this study, as well as Drs Josie Bodle and Liliana Mellor for their technical expertise. They would also like to acknowledge the following funding sources: NWI 11–136 (EGL), NSF/CBET 1133427 (EGL), and NIH CTSA 550KR61325 (EGL).