Abstract
Spectra are fundamental observation data used for astronomical research, but understanding them strongly depends on theoretical models with many fundamental parameters from theoretical calculations. Different models give different insights for understanding a specific object. Hence, laboratory benchmarks for these theoretical models become necessary. An electron beam ion trap is an ideal facility for spectroscopic benchmarks due to its similar conditions of electron density and temperature compared to astrophysical plasmas in stellar coronae, supernova remnants and so on. In this paper, we will describe the performance of a small electron beam ion trap/source facility installed at National Astronomical Observatories, Chinese Academy of Sciences.We present some preliminary experimental results on X-ray emission, ion production, the ionization process of trapped ions as well as the effects of charge exchange on the ionization.
Export citation and abstract BibTeX RIS
1. Introduction
Recently, a large amount of X-ray and extreme ultraviolet (EUV) spectra with high resolution have been obtained by many space missions, such as EUVE, Chandra, XMM-Newton, Hinode and Interface Region Imaging Spectrograph (IRIS). These spectral data will give deep insights on different objects, such as plasma heating, accretion of compact objects, energy storage and release, emission structure, supernova feedback, as well as kinetics and dynamics in X-ray/EUV emitters. However, understanding these spectral data significantly depends on theoretical modeling based upon various assumptions. For example, assumptions of being optically thin with thermal electrons and in equilibrium are extensively used in the Chianti model (Dere et al. 1997) for observations in the solar transition region, chromosphere and corona. Recently, Dudík et al. (2014) further investigated the signature of a non-Maxwellian κ distribution and incorporated it into the Chianti model. The APEC/AtomDB model also adopted the optically thin and thermal electron assumptions (Smith et al. 2001), and is extensively used for X-ray observations from the Chandra and XMM-Newton satellites. Recently, Smith et al. (2012) further incorporated the charge-exchange process and non-equilibrium assumption in the APEC model, which significantly supports our understanding of X-ray spectra fromcomets and planets as well as shocked regions in supernova remnants. In these kinds of astrophysical plasmas, the charge-exchange process, between solar wind ions or hot outflows in supernova remnants and neutral atoms or molecules of cometary/planetary atmospheres as well as neutrals in interstellar mediums, is regarded as an important process for X-ray and EUV photons observed by space missions (Cravens 1997). However, the hydrogenic model adopted by them for the collision cross-section between heavy ions and neutral particles cannot reveal the energy dependence of emitted photon-flux with collisional velocity, where the emitted photon-flux is proportional to the cross-section in the collisions.
All these theoretical models strongly depend on the accuracy and completeness of fundamental atomic parameters. The recent data upgrade of the Chianti model (v8, Del Zanna et al. (2015)) significantly improves its prediction of synthetic spectra compared to its earlier version, as demonstrated by Del Zanna (2012a); Landi et al. (2013). The diagnostics of plasma temperature and density are also affected by the data upgrade in models, for example, Foster et al. (2012) indicated that the G-ratio of He-like ions can change by 60% at a given temperature of 106 K, so the diagnosed temperature can be altered by a factor of 2. Hence laboratory measurement is necessary for spectral modeling, and can help us to benchmark the accuracy and completeness of theoretical models that are used by the astronomical community.
An electron beam ion trap (EBIT) can generate coronal plasmas with temperatures of tens- and kilo-eV with electron densities of 108 − 1011 cm−3 and is therefore an excellent platform for testing spectral models and line catalogs. The earlier laboratory measurements performed by Brown et al. (1998) at the Livermore EBIT facility demonstrated that the earlier spectral model (i.e. MEKAL, Kaastra 1993) missed a lot of weak lines from n = 5 → 2 transitions in its global fitting to the spectrum of Capella obtained with ASCA (Brickhouse et al. 2000). Later, a series of laboratory measurements was performed at the Livermore EBIT by this group for different elements to compile the line list in various spectral models. For example, Lepson et al. (2002) showed the EBIT measurement for Fe VII–Fe X in the wavelength range 60–140 Å. At a shorter wavelength range of 20–75 Å, Lepson et al. (2002, 2005) presented the EBIT measurements for Ar IX–Ar XVI and S VII–S XIV, respectively. Beiersdorfer & Lepson (2012); Beiersdorfer et al. (2012) demonstrated that there are many more emissions for Fe VIII–Fe XVI ions in plasma than those predicted by the Chianti model (v7, Landi et al. 2012). Recently, many more weak unknown lines in the wavelength range of 198–218 Å were revealed by the EBIT measurement in the work Beiersdorfer et al. (2014b). Nakamura et al. (2011) examined some diagnostic line ratios in laboratory measurements of Fe xiii, Fe xiv and Fe xv on their small EBIT (also called CoBIT) developed by them. Density-sensitive line ratios of boronlike Ar xiv and Fe xxii were evaluated by Chen et al. (2004) with the well-understood spectroscopy of heliumlike Nvi.
Some laboratory measurements were done at the EBIT facility to explain the long debated line ratio of 3C vs. 3D from two 3d → 2p transitions of Fe XVII. By changing the electron beam energy, Brown et al. (2001) showed that the low ratios of 3C/3D observed in solar and stellar coronae were attributable to contamination from the inner-shell satellite line of Fe XVI, which coincides with the intercombination 3D line. Its diagnostic utility for plasma temperature was also explored in this work. Later, a detailed measurement of Fe XVI emission was performed again to explore the contribution frominner-shell satellite lines (Graf et al. 2009).Gillaspy et al. (2011) investigated the beam energy dependence of the 3C/3D ratio from different EBIT measurements and found a good agreement between different measured values and theory with excitation data from R-matrix calculations. The widespread feature among available EBIT measurements is due to the energy dependence of excitation cross-section and their monoenergetic measurements. One milestone in research on this subject is the work of Bernitt et al. (2012). By irradiating the trapped ions in EBIT with femtosecond X-ray pulses from a freeelectron laser, the poor agreement between astrophysical observations and theoretical calculations was attributed to the poor quality of underlying atomic wavefunctions rather than insufficientmodeling of collisional processes. However, Loch et al. (2015) demonstrated that the laboratory measurement (2.61 ± 0.23) can be described by theory with the presently calculated gf-value by taking the non-equilibrium effect into account at a given experimental value of radiation field density. Although this is still an issue, these experimental measurements are very helpful for understanding such line ratios.
Solar wind charge-exchange emissions are regarded as a common feature in X-ray observations of comets, planets in the solar system, local hot bubbles, etc. Since its first suggestion by Cravens (1997) for the soft X-ray spectrum of comet Linear C/1999 S4, observed with the Chandra observatory, many theoretical chargeexchange models have been developed to explain the cometary observation. Beiersdorfer et al. (2003) simulated the charge-exchange emissions that occur in comets by operating the EBIT in magnetic mode, where trapped ions interact with selected gases. Recently, such chargeexchange X-ray emission was suggested to explain an unidentified line feature at ∼3.5 keV in bright galaxy clusters and the Andromeda galaxy (Gu et al. 2015). This feature has been regarded as the signal from 'dark matter' in a galaxy. By EBIT measurements, Shah et al. (2016) suggest that this emission at 3.5 keV is possibly due to n ≥ 9 to ground transitions of hydrogen-like sulfur ions. Its upper level population results from chargeexchange processes of trapped bare sulfur with neutral gases. However, there are still many unidentified emissions that need to be cataloged for astrophysical observations (Del Zanna 2012b,a, Beiersdorfer et al. 2014a).
As introduced above, the EBIT is a powerful facility for soft X-ray and EUV spectroscopy in astronomy. This was the motivation for setting up the small EBIT/source facility at National Astronomical Observatories, Chinese Academy of Sciences (NAOC). We firstly describe the operating principle and performance of key parameters associated with this small EBIT/source facility in Section 2. In Section 3, some preliminary experimental results will be presented, including X-ray emissions, ion production, the ionization process of trapped ions as well as the effects of charge exchange on ionization. Finally, we will outline the summary and outlook of our research aim with this small facility in Section 4.
2. A Small EBIT and source
Worldwide, there are a few EBIT facilities, but most of them concentrate on research related to atomic physics. Only a few groups work on astrophysical problems, for example, the EBIT-I and EBIT-II facilities at Lawrence Livermore National Laboratory (LLNL, Beiersdorfer et al. 2001), FLASH-EBIT at Max-Planck Institute for Kernphysics (MPI-K, Crespo López-Urrutia et al. 2003), as well as the CoBIT facility at University of Electro-Communications in Japan (Nakamura et al. 2008).Hence we set up a small EBIT/source (Dresden EBIS-A designed by Dreebit GmbH) facility specifically for astrophysical problems including line catalog in the X-ray and EUV wavelength range, evaluation of diagnostic line ratio, as well as exploration of the line formation mechanism in astrophysical plasmas including the cases of solar wind charge-exchange emissions in planetary and cometary atmospheres, in shocked regions of supernova remnants, as well as in central regions of starburst galaxies.
Figure 1 (left) shows a photograph of the EBIT/source (Dresden EBIS-A) facility at NAOC, as well as a 3D model of the setup (right) including the collision chamber where extracted ions interact with matter. The EBIS-A facility mainly consists of three parts, namely, the main machine, power supply with hardware control units and computer with control software. The main machine runs at room temperature without cryogenic conditions as in classical EBIT devices, and there are also three segments according to their physical properties, namely, ion production, ion extraction and ion collisions between extracted ions with targets. Besides the standard gas injection method used in our laboratory, volatile compounds of metal can also be injected by the Metal Ions fromVOlatile Compounds (MIVOC)method.
Fig. 1 The in-situ picture (left) for the EBIT/source (EBIS-A) facility at NAOC, with its schematic diagram (right) including the collision chamber.
Download figure:
Standard image2.1. Operating Principle of the EBIS
Figure 2 illustrates the operation of EBIS and the configuration of potentials for different electrodes. The electron beam is emitted from a special metal alloy cathode with high-emissivity and a diameter of 1.0 mm. This beam will be accelerated by an electric field between the cathode (Ucath) and first drift tube (U0), and compressed simultaneously by an axial magnetic field of about 0.6T to electron current densities of better than 230 Acm−2. The magnetic field is generated by a permanent magnet configuration. The compressed electron beampasses through an ensemble of three drift tubes where it sequentially ionizes neutral gas to higher charge states via successive electron impact ionizations. These ions are confined with different confinement modes by an axial potential trap of the drift tubes as illustrated by the bottom panel of Figure 2 (closed, leaky, open and pulsed trap with different voltage configurations of the collector at the side of the drift tube). The radial confinement is generated by the space charge potential of the electron beam as shown in the middle part (marked by potential profile) of top panel in Figure 2. After passing through the drift tubes, the electron beam terminates in a water-cooled collector by the negative potential of the repeller electrode relative to the cathode, see the bottom panel in Figure 2. The produced ions can be extracted from the trap by leaky or periodical pulse modes. The extracted ions are focused and position-correctedwith a downstreameinzel lens and two pairs of deflectors. Then the ion beampasses through a crossed electric- and magnetic-field (200 or 500 mT, −1 keV ≤ E ≤ +1 keV), namely a Wien filter. At an exit aperture (1.0 mm diameter), ions with different masses and charge states will be separated. The resolution can be adjusted via selection of the magnets (200 or 500 mT) and by installing different sizes of apertures (0.5, 1.0 and 1.5 mm). Behind the aperture, the ion current will be measured by a Faraday cup connected to a highly sensitive electrometer and mounted in a collision chamber or a diagnostic chamber.
Fig. 2 Top: Schematic diagram illustrating the operation of EBIS-A and its potential configuration with trapped ions in the radial and beam directions. Different colored balls represent different ions with different temperatures, where darker color refers to cooler ions. Bottom: Electrical schematic diagram illustrating the operation of the EBIS: Ucath, cathode potential; U0, trap potential at the e-gun side; UA, the central trap potential; UB1 or B2 the trap potential at collector side.
Download figure:
Standard imageIn this trap, many different atomic processes take place, including electron impact ionization, recombination (radiative and resonant), charge-exchange between charged ions and neutral gas, and excitation and radiative decay via photons emitted at different wavelengths. Various high-resolution detectors and/or spectrometers are mounted in the perpendicular direction to detect these photons. The trapped ions will be heated by the electron beam via Coulomb collision, and exchange energies between different charge states. Ions with enough energy will escape the ion trap in the radial and axial directions and take away kinetic energy, cooling the higher charge states in the trap. Then the charge state distribution and ion temperatures can reach a certain balance at given conditions. Different charged ions have different temperatures as illustrated in Figure 2 by different colored balls. These physical processes can be written as
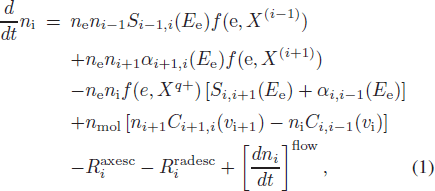
where Si−1,i and αi,i−1 correspond to the electron impact ionization and radiative recombination (RR) rate coefficients, respectively. f(e,Xi) is the overlap factor between the electron beam and the ions. denotes the axial escape rate. ne represents 'geometric' electron density derived from
, where electron velocity
and re refers to radius of the electron beam. Ie and Ee are electron beam current and energy, respectively. C(vi) is the charge exchange rate coefficient, which is a function of the relative velocity (vi) between the trapped ions and neutral particles.
The electron heating process can be described by

where the Coulomb cross section is . Here Zi and lnΛi are ionic charge and the electron-ion Coulomb logarithm, respectively. Energy transfer between the ith and jth ionic species is described as

where νij is the Coulomb collision rate between the ith and jth ionic species. The energy loss due to escaping ions derived from the Fokker-Planck equation (Penetrante et al. 1991) is

where and νi is the total Coulomb collision rate for the ith ionic species. The trapped ions can escape radially and axially, which is governed by the radial and axial potential (Vw) as shown in Figure 2. Many more details on the related physics were described in the work Kalagin et al. (1998).
2.2. Performance of the EBIS
This EBIS facility is an inexpensive compact device, and runs at room temperature with permanent magnets (0.6 T) instead of superconducting coils. At the NAOC laboratory, the vacuum pressure of the EBIS can be decreased to 3 × 10−10 mbar without gas injection. The potentials of various electrodes have been tested up to −5.0 kV for the cathode, 15 kV for the drift tube, −10 kV for the repeller and ±5 kV for the einzel lens. The heating current has been tested up to 4.24 A with a beam current of 172 mA at acceleration voltage of 17.5 kV (namely, U0 = 14.5 kV and Ucath = −3.0 kV). Various charge states of astrophysically abundant elements (e.g. argon and iron in our test) can be extracted and resolved in the collision chamber by applying appropriate electric- and magnetic-fields on the Wien filter. The ion current can be up to nearly 450 pA with ion area-density of ∼1010 cm−2 (i.e. charge of q = 10).
3. Preliminary Results
Since the commissioning of the EBIS machine in Oct. 2015 and its first upgrade of the ion extraction and measurement in July 2016, we have performed some testing measurements, and obtained some preliminary results on X-ray spectra, ion spectra of extracted ions, ionization of ions as well as the effects of charge-exchange on ionization.
3.1. X-ray Spectrum
By using a silicon drift detector (SDD, AmpTek XR-100SDD) with an area of 25mm2 and an energy resolution of 130 eV at 1.8 keV photon energy, we observed X-ray spectra of argon ions due to electron excitations, as shown in Figure 3. Many dominant emission lines of H- and He-like argon are observed obviously, including triplet, Kβ of Ar16+ and Lyα of Ar17+. The triplet lines of Ar16+ are blended together due to lowresolution (∼130 eV) of the SDD. Additionally, we also observed Kα, Kβ lines of iron, which are important features for plasma diagnostics in various objects, e.g. supernova remnants, X-ray binaries and galaxy clusters. In this case, such Kα and Kβ lines of iron are from electrode materials. Wherever those lines of Ir and Cr are from the cathode material composition, another important feature is a very weak RR line at ∼13 keV, which is equivalent to the sum of beam energy and L-shell transition energy. In photoionized plasma of X-ray binaries and active galactic nuclei, for example in Cygnus X-3 (Paerels et al. 2000), RR continuumaround 4.64 keV was used to diagnose the temperature. A sophisticated data acquisition system can dissociate those lines from excitation resonance, dielectronic recombination and RR as described in the works Zou et al. (2003) and Biedermann et al. (2002). Such detailed investigation is very useful for improving spectral models, including diagnostic lines of He-like ions.
Fig. 3 The measured X-ray spectra via SDD for argon at the EBIS facility at a beam energy of 12.13 keV without considering space charge potential. Ion trapping times of 100, 500 and 1000 ms are plotted together.
Download figure:
Standard imageAs stated in the introduction section, high-resolution spectra in laboratory measurements can significantly help modelers to benchmark theoreticalmodels for astrophysical observations. However, the efficiency of photon detection is decreased significantly by using spectrometers such as a crystal or flat-field grazing grating and the detection of weak recombination emissions becomes difficult. For collisional emissions by electrons, the EBISA plasma can generate enough X-ray photons to be detected by using spectrometers with high-resolution due to its high repetition ability and stability. The measured X-ray and EUV spectra will improve our understanding of He-like triplet spectroscopy that can be used for diagnostics of plasma density and temperature, as well as un-identified emissions in soft X-ray and EUV regions. Hence we plan to set up a crystal spectrometer this summer and a flat-field grazing grating spectrometer later this year. The SDD X-ray detector has high efficiency with the loss of resolution and is a good choice for weak recombination emission lines including resonance dielectronic, radiative and charge-exchange recombination lines.
3.2. Ion Spectra of Extracted Ions
In order to investigate charge-exchange spectra that have been discovered fromcometary andMartian atmospheres (Lisse et al. 1996; Dennerl et al. 2006), the extraction of such highly charged ions from EBIT is important. In EBIT measurements reported from Beiersdorfer et al. (2003) and Shah et al. (2016), the discrimination of charge-exchange emission lines was realized by a sophisticated data acquisition system and a fast high-frequency switching on/off unit for the electron beam at their EBIT facilities This was used to diagnose the components of solar winds and investigate the possible origin for the decay emission of 'dark matter' at 3.5 keV in the Perseus cluster (Bulbul et al. 2014). At the NAOC EBIS-A facility, an ultra-high vacuum collision chamber has been installed for such charge-exchange spectra with a solid target. In this context we firstly tested the performance of the extraction system that is part of this facility.
We measured the ion spectra of iron extracted from the EBIS, which was separated by theWien filter, and detected by a Faraday cup, as shown in Figure 4 for leaky and pulse modes. In leaky mode, the potential (40V) is kept constant. In these measurements, the electron beam energy was 11.09 keV (without including space charge potential) and the electron current was 83mA at a vacuum pressure of 6 × 10−9 cm−3. The ion spectroscopy illustrates two different spectral characteristics for the two different extraction modes. In the leaky mode, ions with different charge states (lower and higher) will be extracted, but only highly charged ions are extracted in pulse mode. In the leaky mode, only ions with enough kinetic energy can overflow the potential barrier. In the trap, neutral atoms will be ionized to higher charged ions as determined by the ion ionization potential and the electron beam energy, and the higher charged ions will first obtain thermal energy, as demonstrated in the work of Kalagin et al. (1998) and our recent simulation work on argon, see Figure 5. The physical details for this argon simulation are out of the scope of the paper, and similar to the work of Kalagin et al. (1998), and will be described in the work of Liang et al. (2017). If the trapping time is long enough, the Coulomb collision between ions becomes apparent, and will transfer thermal energy from higher charged ions to lower charged ions. Then the lower charged ions will obtain enough kinetic energy to overcome the potential barrier. This is the reason that more lower charged ions will be extracted in the leaky mode, but not for the pulse mode. Such obvious separation for different charge states will significantly benefit our future experiments on collisions between highly charged ions and neutral particles as they occur between solar wind ions and a planetary or cometary atmosphere.
Fig. 4 Ion spectra of charged iron ions in leaky and pulse modes at a trapping time of 500 ms. X-axis refers to the voltage (V) on the electrodes of the Wien filter. Y-axis refers to the measured ion current.
Download figure:
Standard imageFig. 5 Evolution of ion temperature for argon in the EBIT at electron energy of 9.0 keV and current of 70 mA.
Download figure:
Standard image3.3. Ionization Process of Gas
In a laboratory, a lot of testing measurements need to be done to figure out which conditions and operation parameters are the best choices for ion production in collisional and charge-exchange spectroscopic research. Understanding ion production is also an important test for X-ray and EUV spectroscopic research for a newly customized research facility. The different settings for ionization time will significantly affect the fraction of a certain highly charged ion species as well as its corresponding X-ray and EUV photon generation.
Argon gas is injected into the EBIT via the gas injection system, and the ionization process starts instantly at a vacuum pressure of 5 × 10−9 cm−3. In this experiment, the beam energy is 5.34 keV with a current of 31.5 mA. The central drift is negatively biased to 190 V relative to U0, while UB1 is negatively biased to 0 V to trap/extract or is negatively biased to 210 V to empty. The trap is periodically opened to extract ions, and emitted photons are detected by the SDD as mentioned in the previous subsection. The trapping time is ramped up from 20 ms up to 250 ms with a step size of 5 ms, while the data acquisition time is kept constant in each measurement. The measured X-ray spectra are plotted in Figure 6 (right). From this figure, we can see that the photon energy of the argon Kα line steadily increases with longer trapping time. Such increase of photon energy is due to increasing charge of trapped ions. It is well known that the Kα line energy is higher for ions with more charge. At the photon energy of 3.6 keV, a weak Kβ line is also observed at longer trapping time.
Fig. 6 Schematic diagram of the experimental setup (left) and measured X-ray spectra of charged argon ions at a vacuum of 5.0 × 10−9 cm−3 (right). The trapping time is ramped up from 20 ms to 250 ms with a step size of 5 ms, while the data acquisition time is kept constant in each measurement. The trapping potential (UA – UB1) is periodically switched on/off by shifting UB1 (trapping) to UB2 (empty) and back to empty the trap and restart ion production and accumulation. The excited X-ray photons are detected by an SDD. Different colors represent the photon counts as marked by the color bar at the bottom.
Download figure:
Standard image3.4. Charge-exchange Effect on Ion Production
As discussed in Section 2.1, the charge-exchange process is a basic atomic process in the trap center. This affects the distribution of different charged ions in the trap and corresponding ionic population for a given charge at the collision chamber. We plan to explore research on charge-exchange spectroscopy in the near future. Hence we first examined such charge-exchange effects on neutral gas in our vacuum conditions.
At a lower vacuum pressure of 2 × 10−9 cm−3 (a lower neutral gas abundance) in the trap, we performed similar X-ray measurements with the same beam energy of 5.34 keV and electron beam currents of 31.3 mA, as well as the same trap potential of 190 V. By fitting each spectrum shown in the scatter plots in Figures 6 and 7 with a single Gaussian profile, we obtain the photon energy and its full-width at half-maximum (FWHM) as shown in the right panel of Figure 7.
Fig. 7 A scatter plot of X-ray spectra of highly charged argon ions at a lower vacuum pressure of 2 × 10−9 cm−3 (left), and a comparison of the Kα photon energy (in keV, left tick-label for filled symbols) and (FWHM in eV, right tick-label for open symbols) at two different vacuum pressures (5 × 10−9 mbar for open/filled circles, 2 × 10−9 mbar for open/filled triangles) (right).
Download figure:
Standard imageWe notice that the curve profile of Kα photon energy and the FWHM vs. ionization time are kept constant at different vacuum pressures. This reveals that the ion production is not affected by the vacuum pressure, that is, the charge-exchange effects with neutrals are very weak relative to the ionization at the present vacuum pressure. The slope of the curve of Kα photon energy becomes smaller with longer trapping time. This reveals that the L-shell ions appear at a trapping time of ∼150 ms, because the photon energy increases nonlinearly and slowly after this trapping time. At the trapping time of 100–150 ms, the FWHM reaches a maximum value of 160 eV which is higher than the SDD resolution of about 110 eV at 3.0 keV. This indicates that the Kα lines from different M-shell charged ions contaminate each other, and they have comparable fractions in the trap center. After the trapping time of 150 ms, the FWHM decreases and reaches the highest resolution of the SDD. This results from the fact that the L-shell ions dominate the trap at sufficient ionization/trapping time.
4. Summary and Outlook
In summary, the EBIT/source facility at NAOC is a compact and economical device for spectroscopic investigation of astrophysical plasmas. All abundant ions in the cosmos can be produced due to its wide beam energies spanning hundreds of eV to 20 keV. Test measurements of X-ray spectra with low-resolution reveal that the facility runs well and hydrogen-like iron ions can be produced. Trapping time plays an important role in ion production and their distribution in the trap. Additionally, the produced ions can be extracted in leaky or pulsemode with this facility and the ion current can be up to hundreds of pA with an ion density of 107 cm−3.
A spectrometer with high-resolution will help us to perform line catalog measurements in soft X-ray and EUV regions where many emission lines are not well known. Such a crystal spectrometer for the X-ray wavelength region is being manufactured and will be installed this summer. Additionally, an EUV spectrometer with high resolution is also being planned this year. In the EBIT/source, there are many processes taking place. A sophisticated data acquisition method will help to resolve different processes in the trap center. For example, the charge-exchange spectra can be observed with a timing procedure of the electron beam (on/off) synchronized with the data acquisition system. This will significantly improve our understanding of charge-exchange X-ray spectra observed in cometary/planetary atmospheres and in the shocked region of supernova remnants, as well as clarify unknown emission lines at ∼3.5 keV which are being investigated as originating from dark matter. Furthermore, the collision chamber will help us understand collisions between cosmic particles and the interstellar medium.
Acknowledgements
This work is supported by the National Key R&D Program of China (No. 2017YFA0402401) and the National Natural Science Foundation of China (Grant No. 11522326), as well as the National Basic Research Program of China (973 Program, Grant 2013CBA01503). LGY also acknowledges support by the Science Challenge Project (No. TZ2016005).