Abstract
We show that a Fermi surface in underdoped YBa2Cu3O6+x yielding the distribution of quantum oscillation frequencies observed over a broad range of magnetic field can be reconciled with the wavevectors of charge modulations found in nuclear magnetic resonance and x-ray scattering experiments within a model of biaxial charge ordering occurring in a bilayer CuO2 planar system. Bilayer coupling introduces the possibility of different period modulations and quantum oscillation frequencies corresponding to each of the bonding and antibonding bands, which can be reconciled with recent experimental observations.
Export citation and abstract BibTeX RIS

Content from this work may be used under the terms of the Creative Commons Attribution-NonCommercial-ShareAlike 3.0 licence. Any further distribution of this work must maintain attribution to the author(s) and the title of the work, journal citation and DOI.
Our level of understanding of metals typically depends on the extent to which Fermi surface cross-sections observed in quantum oscillation experiments can be explained by comparison with band structure calculations, and the degree to which Fermi liquid behavior prevails [1–4]. Additional factors, such as a periodic modulation of the electronic states—caused, for example, by a spin- or charge-density wave—can introduce additional gaps, in which case the Fermi surface needs to be understood in terms of the original band structure translated by ordering wavevectors [4–7].
In the case of high temperature superconductors, a determination of the electronic structure is essential for understanding the origin of pairing. Yet, the small Fermi surface pocket observed in the underdoped cuprates YBa2Cu3O6+x [8] and YBa2Cu4O8 [9] cannot readily be explained in terms of the unmodified band structure [9–17]. The momentum-space cross-section of the experimentally observed pocket is roughly thirty times smaller than the size of the large hole Fermi surface sections expected for the CuO2 planes from band structure calculations [18–20], with the cyclotron motion having apparently the opposite sense of rotation to that expected [10, 12]. A small pocket has been proposed to originate from band structure involving the mixing of the chain states with BaO orbitals in YBa2Cu3O6+x [19, 20], but the relevant band is reported to lie significantly (≈0.6 eV) below the Fermi energy in photoemission experiments [11].
The emergence of long range charge order reported in underdoped YBa2Cu3O6+x by nuclear magnetic resonance (NMR) [21] and x-ray scattering [22–24] may contribute to the observed difference between the small Fermi surface pocket measured and the large Fermi surface expected from band structure. A direct link, however, has yet to be established between the structure of the measured order and reconstruction of the large Fermi surface to yield the observed small pocket. Furthermore, some differences are reported in the ordering wavevectors seen in different experiments [21–24], and it remains to be settled whether the observed charge order in YBa2Cu3O6+x is stripe-like [25–27] as opposed to biaxial [17, 28] in nature.
A satisfactory electronic structure model of underdoped YBa2Cu3O6+x would be expected to explain the ordering wavevectors found in both NMR and x-ray experiments, while accounting for the measured distribution of bilayer-split frequencies in quantum oscillation experiments [16, 29], as well as other experimental observations relating to the electronic structure [10–12, 17, 30, 32]. A single layer scheme of Fermi surface reconstruction by biaxial charge order has previously been shown to yield nodal electron pockets [15, 17, 28, 34] consistent with experimental observations of the small size of the electronic heat capacity at high magnetic fields [30]4, the negative electrical transport coefficients [10, 12] and the predominantly nodal Fermi surface location inferred from photoemission, Fermi velocity and chemical potential oscillation measurements [11, 17, 32]. In this paper, we show that the introduction of bilayer coupling [33] to the single layer scheme [15, 17, 28, 34] leads to the possibility of a modulation period and Fermi surface pocket of different size corresponding to each of the bonding and antibonding bands, consistent both with experimental measurements of the charge ordering [21–24] and quantum oscillations [16, 29].
NMR experiments provide evidence for long range charge ordering in a magnetic field B⪆15 T [21] in underdoped YBa2Cu3O6+x, while recent x-ray scattering experiments reveal ordering vectors that are two-dimensional (2D) [22–24]. The x-ray results are significant for two reasons. Firstly, they reveal the scattering intensity and correlation lengths to be equivalent along the a and b lattice directions of the orthorhombic unit cell [18], with the orthorhombicity and CuO chain oxygen ordering having no apparent effect in causing stripe domains to form preferentially along either a or b. Secondly, they reveal the charge ordering to be incommensurate with wavevectors and
(of in-plane period λa = λb ≈ 3.2 lattice constants) consistent with a wavevector nesting the bilayer-split bonding band [22–24] (where Qz refers to the c-axis component of the ordering vector). The periods reported in x-ray scattering experiments, however, differ from that (λa = 4.0) reported in NMR studies [21] of similar YBa2Cu3O6+x samples and that (λ ≈ 4.2) [35] typically found by x-ray diffraction in the same region of the phase diagram in single layer spin-stripe ordered cuprates [36]. Furthermore, no obvious relationship is found between the charge ordering wave vectors found in x-ray experiments on YBa2Cu3O6+x and low energy spin excitation wave vectors [37].
Given the unambiguous planar origin of the 2D charge ordering found in x-ray scattering experiments in YBa2Cu3O6+x [24], we focus here on a model dispersion solely for the CuO2 bilayers. While photoemission experiments have yet to identify signatures of band folding consistent with charge ordering, the wave vectors found using x-rays in YBa2Cu3O6+x [22, 23], as well as those found earlier in doping-dependent scanning tunneling microscopy measurements in other cuprates [38, 39], suggest Fermi surface nesting at the antinodes [34, 38]. The opening of an antinodal gap (shaded light grey in figure 1) that is expected to accompany ordering at such wave vectors is consistent with a reconstructed Fermi surface that involves relative translations of the remaining arc-like segments of Fermi surface (represented by thick black lines in figure 1 [17, 28, 34]). These translated segments of Fermi surface would occupy the same regions in momentum space where 'Fermi arcs' are observed in photoemission experiments in underdoped cuprates [11, 39–41].
Figure 1. Single layer schematic showing how gapping of the electronic density of states (DOS) at the antinodes (depicted in grey) leaves remaining arc-like segments of Fermi surface [17, 28, 34] (depicted in black), whose reconnection upon translation by Qa and Qb leads to the possibility of a nodal pocket for which the sense of cyclotron motion in a magnetic field is opposite to that of the original hole sheets. Thick black lines represent ε(k), thick magenta lines represent ε(k + Qa) and ε(k + Qb), while thick cyan lines represent ε(k + Qa + Qb). No direct coupling occurs between ε(k) and ε(k + Qa + Qb) in the model, however, ε(k) and ε(k + Qa + Qb) are both coupled to ε(k + Qa) and ε(k + Qb), thus leading to a closed pocket.
Download figure:
Standard imageThe single layer unreconstructed Fermi surface produced using ε(k) = ε0 + 2t10[cos akx + cos bky] + 2t11[cos(akx + bky) + cos(akx − bky)] + 2t20[cos 2akx + cos 2bky] has a single nesting wavevector spanning the antinodal regions (i.e. |Qa| = |Qb| in figure 2(a)). On introducing bilayer splitting, two nesting vectors now span the bonding and antibonding bands such that |QBa| = |QBb| and |QABa| = |QABb| are inequivalent (shown in figure 2(b)). For the single-layer dispersion5, we choose t11/t10 = −0.32 and t20/t10 = 0.16 after Andersen et al [18] and Millis and Norman [25]. The bonding and antibonding bands are obtained by diagonalizing

after Garcia-Aldea and Chakravarty [33], where

and tc represent intrabilayer and interbilayer couplings, respectively. On evaluating equation (1) one obtains

for the bonding and antibonding bands (shown for cos ckz = 0 in figure 2(b), where c refers to the c-axis lattice parameter. Setting t⊥0/t10 ≈ 0.5 and tc/t⊥0 = 0.25 produces a bilayer splitting at the antinodes ( and
) resembling that in bandstructure calculations [19, 20] in YBa2Cu3O6+x, but with a reduced nodal gap (εg ≈ 2tc) that falls within the experimental upper bound from photoemission [41] and magnetic quantum oscillation measurements [16].
Figure 2. (a) Single layer Fermi surface corresponding to ε(k) as defined in the text. (b) Bilayer Fermi surface after equation (2) for kz = 0, where the red and blue lines correspond to bonding and antibonding bands respectively. The cross-sectional area of the Fermi surface represents an effective hole doping of p = 8% relative to the half-filled band, which we estimate from the total width of the chain Fermi surface seen in photoemission experiments on ortho-II ordered YBa2Cu3O6+x [11]. QB and QAB refer to nesting wavevectors of the bonding and antibonding bands respectively, while Qa and Qb pertain to the different lattice directions in the orthorhombic unit cell [18]. εgap refers to the nodal energy gap.
Download figure:
Standard imageThe corrugation of the unreconstructed bilayer Fermi surface with respect to the interlayer momentum kz (shown in figure 3) caused by t⊥0 and tc implies that Fermi surface nesting is optimized by acquiring a Qz = π component to the wave vector. Such a component produces a reconstructed Fermi surface in which the fundamental component of the corrugation is suppressed, leaving a small residual second harmonic corrugation of order t2c/t⊥0 (originating from the square root in equation (2)). Hard x-ray scattering experiments find direct evidence for an interlayer component to the ordering vector [12]. Furthermore, quantum oscillation experiments performed over a broad range of magnetic field and angles are found to be consistent with a bilayer-split Fermi surface that exhibits a comparatively weak corrugation [15, 16]. The strong suppression of the interlayer optical conductivity within the underdoped regime [42] could be a further signature of Fermi surface corrugation suppressed by nesting. The small size of the residual corrugation term (which we estimate later in the manuscript) compared to the other energy scales enables us to consider a simplified 2D approximation to the Fermi surface, which we obtain by neglecting the kz-dependence in equation (2).
Figure 3. Schematic of the interlayer Fermi surface corrugation at bky = ± π according to equation (2). Owing to a Qz = π component, translation (dotted lines) of the bonding and antibonding Fermi surfaces by QB and QAB, respectively, leads to an almost perfect interlayer Fermi surface nesting at the antinodes.
Download figure:
Standard imageTo obtain the full set of reconstructed bands, the relevant couplings (VB,ABa,b) between bands with relative translations by ±QBa, ±QBb, ±QABa and ±QABb need to be considered (including both the bonding and antibonding bands given by equation (2)), requiring the construction of a Hamiltonian in the form of a matrix (see e.g., [25, 33]). Orthogonality between the Bloch wavefunctions of the bonding and antibonding bands ensures that charge-density modulations form independently for those two bands, with the coupling between them being a second order effect6. The strongest coupling Va,b(k') is therefore expected to occur between states within each of the bands translated by wavevectors intrinsic to that band—i.e. between ε(k)B and ε(k ± QBa,b)B and between ε(k)AB and ε(k ± QABa,b)AB.
While the complete set of bands for strictly incommensurate order (in which λB and λAB are irrational) requires an infinite number of translations and a matrix of infinite rank, which cannot be diagonalized, it was shown in [34] that one can approximate incommensurate behavior by considering rational values of λ (in which λ is the ratio of two integers)—yielding large but manageable matrices. Provided 0 ≪ |Va,b(k')| < |t10|, the Fermi surface consists of multiple repetitions of the same electron pocket throughout the Brillouin zone [34]. Here we go a step further in simplification by considering only the minimal number of terms necessary to produce a single instance of the electron pocket for each band (e.g. those illustrated in figure 1). Hence,
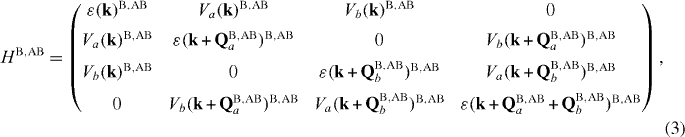
which must be diagonalized for both bonding and antibonding bands. With these simplifications, we can estimate the size of the pockets, their effective masses and contributions to the electronic DOS for arbitrary values of λB or λAB—bearing in mind that the complete Fermi surface will have these same pockets repeated by ±QBa, ±QBb, ±QABa and ±QABb and combinations thereof throughout the Brillouin zone.
We proceed to obtain the Fermi surface for a given strength (V0) of the potential by determining the minimum in the total electronic DOS for the bonding and antibonding bands as a function of QBa,b and QABa,b. We utilize the fact that the DOS is further minimized by considering momentum-dependent couplings of the form [34]

In real-space, r corresponds to a modulation of bond strength, for which there is evidence in recent experiments [24]. The value of r is tuned so as to suppress the energetically unfavourable coupling between translated states whose group velocities point in the same direction. By adjusting V0, t⊥0, rB and rAB (where B and AB refer to the bonding and antibonding bands, respectively), we are then able to tune the locations in λB and λAB of the minima in the DOS.
We find that the wavevectors QBa,b and QABa,b at which the electronic DOS is minimized when V0/t10 = 0.7, t⊥0/t10 = 0.54, rB ≈ 1.65 and rAB ≈ 1.35 correspond closely to the wavevectors reported in x-ray scattering and NMR experiments. These same wavevectors also produce nodal pockets (see figure 4) with areas consistent with those found in recent quantum oscillation experiments uncovering a bilayer-split Fermi surface (see figure 5(a)). Figure 5(b) shows the electronic DOS (solid lines) for V0/t10 = 0.7 plotted as a function of 1/λ for both the bonding and antibonding bands in units of the effective mass. The deep minima (where the free energy is minimized) are located at λBa,b = 3.30 and λABa,b = 4.05, which are very similar to the values λa,b = 3.28 and λa = 4.00 reported in x-ray scattering [22–24] and NMR [21] experiments, respectively, performed on underdoped YBa2Cu3O6+x.7
Figure 4. Bonding (red) and antibonding (blue) nodal electron Fermi surface pockets obtained using equation (3) for V0/t = 0.7, depicted in the extended Brillouin zone representation. Black lines indicate the unreconstructed Fermi surface shown in figure 2(b). Grey lines represent the remaining unnested portions of Fermi surface resulting from the inclusion of only the lowest order terms in equation (3). The grey lines become reconstructed into multiple repetitions of the pockets throughout the extended Brillouin zone on including more terms in the Hamiltonian [34]. A schematic representation of the complete reconstructed Fermi surface is produced by translating the closed pockets by ±QBa, ±QBb, ±QABa and ±QABb and all multiples and combinations thereof (not shown for clarity).
Download figure:
Standard imageFigure 5. (a) The solid lines (left-hand axis) show the optimal values of λB and λAB at which the total electronic DOS is minimized, which are found to depend on the coupling strength V0. Both the bonding (B) and antibonding (AB) bands are shown. At V0/t10 = 0.7, the values of λ agree with those obtained from x-ray scattering [22] and NMR [21] experiments (filled circles). The dotted line (right-hand axis) shows the corresponding pocket frequencies calculated for the bonding and antibonding bands (related to the pocket area via Onsager's relation F = (ℏ/2πe)Ak), which also depend on V0. At V0/t10 = 0.7, the frequencies also coincide with the bilayer-split extremal orbits F0 ± 2ΔF (filled squares) inferred from quantum oscillations in which the frequencies F0 and F0 ± ΔF are observed [16]. (b) Electronic DOS for V0/t10 = 0.7 (plotted in units of the band mass mb per band in free electron masses me) at the Fermi energy as a function of 1/λ, revealing a clear gap for each band with a minimum that is used to infer the optimal value of λ. Red and blue lines correspond to the DOS of the reconstructed bonding and antibonding bands obtained from equation (3). Dots indicate the minimum DOS per band within the reconstructed Brillouin zone when all multiples of QB,ABa,b are included in equation (3) (see footnote 7).
Download figure:
Standard imageUsing Onsager's relation, F = (ℏ/2πe)Ak (where Ak is the Fermi surface orbit cross-section in momentum space), we obtain FB ≈ 337 T and FAB ≈ 709 T quantum oscillation frequencies for the model Fermi surface pockets in figure 4 when V0/t10 = 0.7 (see figure 5). The size of the closed orbits is not found to be significantly impacted by the inclusion of higher order terms in the Hamiltonian in the limit |Va,b(k')| < t10, justifying our neglect of such terms for estimating the orbit areas and their effective masses. The observed frequencies would be further modified due to magnetic breakdown tunnelling, which is expected due to the suppressed gap εgap ≈ 10 meV between bonding and antibonding bands at the nodes in underdoped YBa2Cu3O6+x, as seen in photoemission experiments [41]. The low effective mass of m0 ≈ 0.34 me for the prominent frequency F0 from band structure calculations [18, 25] due to the hopping parameter t10 = 380 meV implies a renormalization of the bands (and subsequently V0) by a factor of ≈4.7 in the vicinity of the Fermi energy for consistency with the experimentally measured value m* ≈ 1.6 me. Such a value of the renormalization yields for the residual corrugation (neglected in the 2D Fermi surface approximation) (t2c/t⊥0)/4.7 ≈ 2.5 meV, which is comparable in magnitude to the small interlayer coherence temperature (≈27 K) reported in interlayer transport experiments [43]. Finally, we obtain V0 = 0.7 × 380/4.7 ≈ 57 meV for the strength of the charge order parameter, which, on taking T0 ≈ 135 K [23] for the ordering temperature, yields 2V0/kBT0 ≈ 10. While this ratio is large, it lies within the range of values, 6 <2V0/kBT0 < 17, found experimentally in typical charge-density wave systems [44–48]. V0 is also of sufficient strength to overcome incoherence of the bands reported at the anodes [52].
The magnetic breakdown resulting from the small gap εgap between bonding and antibonding bands at the nodes (see figure 6) is expected to occur at four points around each pocket (in the repeated Brillouin zone), giving rise to a sequence of five frequencies F0 + mΔF (where m = −2, −1, 0, 1 and 2) in which the original bilayer-split frequencies correspond to FB = F0 − 2ΔF and FAB = F0 + 2ΔF [16] (see figure 4). A small magnetic breakdown gap (εgap) would further cause the central frequency F0 (depicted in figure 6) to dominate [16] with weaker adjacent features at F0 − ΔF and F0 + ΔF [16], while the spectral features at F0 ± 2ΔF are expected to be further suppressed in amplitude [16]. The calculated values F0 ≈ 519 T and ΔF ≈ 95 T compare favorably with those F0 ≈ 532 T and ΔF ≈ 90 T found experimentally [16].
Figure 6. (a) Schematic showing how magnetic breakdown tunneling (black dotted line) leads to the observation of the F0 frequency, which dominates the quantum oscillation Fourier spectrum, considering the hypothetical case where the bonding and antibonding charge ordering vectors are the same (i.e. QB = QAB) after [16]. On the left-hand-side, magnetic breakdown tunneling of probability [2] (where ωc = eB/m* is the cyclotron frequency) across the nodal gap εgap separating bonding and antibonding Fermi surfaces (indicated in red and blue respectively) is seen to occur at four equivalent points by symmetry around each of the reconstructed pockets. The resulting magnetic breakdown orbit is indicated on the right-hand-side. (b) On considering the case relevant to the present paper in which QB ≠ QAB, several pockets with relative momentum space translations of ±(QB − QAB) are incorporated into the magnetic breakdown path (left-hand-side schematic). For clarity, only those pockets directly involved in magnetic breakdown tunneling are shown (the complete Fermi surface would consist of the repeated translation of these pockets by all possible combinations of QBa, QBb, QABa and QABb). The magnetic breakdown orbit arising from the four equivalent symmetry-related points of magnetic breakdown tunneling (neglecting smaller gaps) in this case has the same frequency F0 (right-hand-side) as that in (a).
Download figure:
Standard imageWe turn to the question of why the wavevectors for the bonding and antibonding bands might be detected in different experiments [21, 22] and consider whether details of the Fermi surface reconstruction could provide potential clues. X-ray scattering experiments are expected to be sensitive to the momentum-dependent susceptibility [22–24], which is largest for the sections of the Fermi surface that are better nested and most completely gapped once Fermi surface reconstruction takes place. We find the electronic DOS to be lower for the bonding band (reflecting its flatter topology), which could potentially account for the observation of λB in x-ray scattering experiments [22–24]. Further experiments are required to determine whether a secondary feature can be seen with x-rays corresponding to the antibonding band at λABa,b ≈ 4; possibly induced by the application of a magnetic field at low temperatures [49]. NMR, by contrast, is expected to yield a splitting when the charge modulations become commensurate with (or locks-in to) the underlying crystalline lattice [21]; owing to its proximity to an integer value, such a possibility is more likely for λAB.
We also note that whereas the formation of a CuO2 plane-centered charge modulation involving the bonding band [23] is mostly decoupled from oxygen ordering within the chains, as indicated by recent x-ray scattering experiments [24], the opposite may be true for a charge modulation involving the antibonding band owing to a greater overlap of its wave function with the chains (the chains themselves being prone to different forms of order [50, 51]). Local interactions with the oxygen order could cause the latter to become more susceptible to a lock-in transition or to differences in the strength of the modulation along the a and b lattice directions, potentially revealed in NMR experiments [21].
In summary, we have shown that the different wavevectors reported in x-ray scattering and NMR experiments and the frequencies observed in quantum oscillation experiments [16] can be consistently explained by biaxial charge ordering involving different nesting vectors for the bonding and antibonding bands in the bilayer cuprate YBa2Cu3O6+x [16, 18, 33]. We note that the negative electrical transport coefficients, small size of the high magnetic field electronic heat capacity and chemical potential oscillations are similar to those expected in previously considered biaxial charge ordering models in which the effects of bilayer coupling were neglected [15, 28, 34]. The high purity of YBa2Cu3O6+x samples together with their suitability for both quantum oscillation and x-ray scattering experiments make these materials a model system for understanding the interplay between charge ordering and superconductivity in the cuprates.
Acknowledgments
This work is supported by the Royal Society, King's College (Cambridge University), US Department of Energy BES 'Science at 100 T,' the National Science Foundation and the State of Florida.
Footnotes
- 4
Assuming that the electronic contribution to the heat capacity approaches the normal state value asymptotically as the upper critical field Hc2 is approached [31] where μ0Hc2 ≲ 100 T in underdoped YBa2Cu3O6+x.
- 5
This choice approximately reproduces the form of the large planar Fermi surface sections found in several different calculations of the electronic band structure [18–20] that is broadly consistent with photoemission experiments [11, 41]. Variations in the hopping parameters will affect the values of λ and F0 in the model.
- 6
Shifts in the atomic positions associated with a lattice distortion will introduce mixing between the bonding and antibonding modulations, such that each band becomes subject to the others modulation, introducing a second order coupling between ε(k)B and ε(k + QABa,b)B and between ε(k)AB and ε(k + QBa,b)AB.
- 7
Since only a limited number of terms are included in equation (3), the DOS does not reach as low a value as it would were all multiples of QA,ABa,b included (i.e. there remain contributions from the unnested portions of Fermi surface (grey lines) in figure 4). On including all terms in the Hamiltonian when λ is commensurate with the underlying lattice [34] the total DOS per band falls to a lower value corresponding to that of a single closed pocket for both the bonding and antibonding bands (indicated by circles in figure 5(b)).