Abstract
This paper reports a dual resonant rectilinear-to-rotary oscillation converter (RROC) for low frequency broadband electromagnetic energy harvesting from ambient vibrations. An approximate theoretical model has been established to integrate the electromechanical coupling into a comprehensive electromagnetic-dynamic model of the dual resonant RROC. Numerical simulation has proved the nature of dual resonances by revealing that both the rectilinear resonance and the rotary resonance could be achieved when the stand-alone rectilinear oscillator (RLO) and the stand-alone rotary oscillator (RTO) were excited independently. Simulation on the magnetically coupled RROC has also shown that the rectilinear resonance and the rotary resonance could be obtained simultaneously in the low-frequency region (2–14 Hz) with well-defined restoring torque (Mr) and the initial rotation angle of the RLO (ψ). The magnetic interaction patterns between the rectilinear and the RTOs have been categorized based on aforementioned simulation results. Both simulation and experimental results have demonstrated broadband output attributing from the dual resonances. Experimental results have also indicated that the RROC could have wide bandwidth in a much lower frequency region (2–8 Hz) even without the rotary resonance as long as the system parameters are carefully tuned. Parameter analysis on different values of Mr and ψ are experimentally carried out to provide a quantitative guidance of designing the RROC to achieve an optimal power density.
Export citation and abstract BibTeX RIS
1. Introduction
With the advancement of low power consumption wireless electronic devices, the emerging field on harvesting energy from ambient vibrations for such devices using piezoelectric [1–3] and electromagnetic transducers [4–7] has been attracting numerous research interests. Study on circuit design for energy harvesters using switching techniques to increase harvesting efficiency has also been done [8, 9]. However, conventional linear energy harvesters (LEHs) can only work efficiently in the narrow frequency band matching their fundamental resonant frequencies, and a slight frequency-mismatch would significantly reduce their power density. However, ambient vibrations are usually rich in broadband, under which the conventional LEHs have notoriously limited efficiency. In order to widen the output bandwidth, active tuning mechanisms have been developed in the literature to adjust the natural frequency of the harvesters in response to the excitation frequency [10, 11]. To reduce the power consumption required for frequency tuning and obtain high net power output, advanced tuning methods have also emerged. It was shown that switching the piezoelectric element on an electrical network for a brief time period each time the deflection crosses a zero value could increase the effective bandwidth by a factor of four [12, 13]. Frequency tuning in an energy-autonomous way enabled the control unit to be optimized to an ultralow power consumption and up to 90% of the harvested energy could be fed to the powered electrical load [14]. Other techniques including passive self-tuning [15], adaptive frequency tuning [16], electrical resonant frequency tuning [17] and parametrically tuning through an active electrical load [18] have been studied. Another popular approach is to enable nonlinearity of the energy harvesters to obtain high-energy orbits by introducing external magnetic forces. The so-called nonlinear energy harvesters (NEHs) could be designed as softening or hardening configurations to widen output bandwidth under excitations with slowly varying frequencies [19]. Depending on the positions of these external magnets, NEHs can also be configured as monostable, bistable and tristable in terms of the number of their static stable states [3, 20–25]. Study shows that in comparison to bistable NEHs, the tristable harvester could easily achieve inter-well oscillations for generating higher energy output over a wider range of operating frequencies and the depth of the potential well is revealed to have essential influence on the harvesting performance [21]. Multiple cantilever beams with different but close natural frequencies have been integrated to achieve broad bandwidth [26–28] but there is no interaction between these cantilever beams. In order to explore the interaction effect on the broadband output, two degree-of-freedom harvesters have been constructed [29–32] to have double resonant peaks and increased frequency bandwidth. The two aforementioned approaches could also be combined, such as using magnetic forces to couple two cantilever beams, and to form a nonlinear two degree-of-freedom energy harvester [33, 34]. Experimental investigation has shown a nearly 100% increase in the operating bandwidth and a 41% increase in the magnitude of the power output at an excitation level of 2 m s−2 [34]. Such NEHs could make the resonant peaks commensurable with suitable parameters, which results in more resonant peaks and wider bandwidth. Experiments have demonstrated a 130% increase in the bandwidth in comparison to their linear counterparts at an excitation level of 2 m s−2 [35].
We previously reported an electromagnetic energy harvester (EMEH), featuring conversion from rectilinear vibrations into rotary oscillations through non-contact magnetic coupling and intrinsic frequency up-conversion. The proposed EMEH was demonstrated to be capable of harvesting energy from broadband low-frequency vibrations efficiently [36]. This paper reports a dual resonant rectilinear-to-rotary oscillation converter (RROC) that builds off our previously reported EMEH platform. Both rectilinear resonance and rotary resonance could be achieved simultaneously in the low-frequency region (2–14 Hz) by the well-defined parametric and configuration tuning, further broadening the bandwidth. A theoretical model of the electromechanical coupling originated from mathematical deduction and experimental verification has been established to derive a comprehensive electromagnetic-dynamic model of the dual resonant RROC. Numerical simulation based on the model provides insights of the magnetic interaction between the rectilinear and the rotary oscillators (RTOs), and interaction patterns are categorized. Broadband output in the low-frequency region attributing from the dual resonances is demonstrated by modeling, simulation and experimental results.
2. Dual resonant RROC
The RROC builds off our previously reported rectilinear-to rotary oscillating platform [36] (figure 1(a)), where circularly distributed potential wells and restoring torques were generated with eight angular equilibrium positions (0, π/4, 2π/4, 3π/4, π, 5π/4, 6π/4, 7π/4). The RTO has a rotor with four arc magnets and a stator with two repulsive rectangular magnets and six coils (2000 turns, 50 Ω). For each coil, a steel screw is inserted inside to increase the magnetic flux cross the coil and generated magnetic interaction with the magnets on the rotor, which along with the two repulsive magnets create the desired potential well distribution. The rectilinear oscillator (RLO) also has four arc magnets distributed centro-symmetrically. A schematic diagram of the magnetic coupling of the dual resonant RROC is illustrated in figure 1(b), where and
refer to the magnetic moment vectors of the RLO magnet and the rotor magnet, R is distance from the rotor magnet to the rotor center,
is the vector from the RLO magnet to the rotor magnet, h is the relative translation displacement of the RLO (along z axis), θ is the rotation angle of the rotor magnet,
is the pre-defined initial angle of the RLO magnet (the angle between
and x axis).
Figure 1. (a) Photo representation of the dual resonant RROC (120 mm OD × 170 mm H), which consists of a magnetically coupled RLO (top) and a RTO (bottom), and (b) schematic diagram of magnetic coupling of the dual resonant RROC.
Download figure:
Standard image High-resolution imageThe dual resonances could be easily realized by adjusting the relative positions of the two repulsive magnets (d1 and d2), which in turn, tuning the depths of the potential wells and therefore the restoring torques Mr (figure 2). More specifically, positions of the two repulsive magnets play a critical role in tuning the structural stiffness in the small-angle rotation region, i.e. Mr1 represents the stiffer RROC than that of Mr4 within a small range of θ. The positions of two repulsive magnets investigated are: Mr1: (11.9 mm, 14.4 mm), Mr2: (11.9 mm, 13.2 mm), Mr3: (11.1 mm, 13.2 mm) and Mr4: (11.9 mm, 12.8 mm). The inserted figure shows the potential energies. Only the results from −π/4 to π/4 are shown but they could be extended to a range of 2π because of the centrosymmetric nature of the ROCC. Figure 2 also shows that the potential wells are positioned at 0, π/2, π and 3π/2, which indicates these four angular equilibrium positions are maintained but the other four undesired positions at π/4, 3π/4, 5π/4 and 7π/4 are removed since no potential wells reside at those positions [36]. The measured restoring torques are curve fitted using a sum of sine functions as

where Ai, Bi and Ci are the curve fitting coefficients. According to the magnetic pattern shown in figure 1(b), the magnetic forces between the RLO magnet and the rotor magnet could be determined by the relative translation displacement h and the angular displacement [36]

In equation (2), is the vacuum permeability, r is the norm of
R is distance from the rotor magnet to the rotor center and
is the norm of
and
Since both the RLO and the rotor have four magnets, the comprehensive force F and torque
are [36]

where k is 0.906, a correction factor identified from experiment to consider the size effect of the magnets. Equation (3) will be integrated into the governing equations for the numerical simulation.
Figure 2. Measured and curve fitted restoring torque Mr corresponding to different positions (d1, d2) of two repulsive magnets.
Download figure:
Standard image High-resolution image3. Electromechanical coupling
The electromagnetic voltage of a coil subject to magnetic flux variation is expressed as

where N is the number of coil turns, H is the magnetic field strength. A is the area of the coil cross-section and is the relative permeability of the magnetic core inside the coil. During the modeling process of the RROC, the difficulty to include the electrical configuration arises from the nonlinear B–H curve of the steel screws inside the coils, i.e.,
in equation (4) is not constant but dependent on H. In order to avoid introducing the complex permeability of the steel screws,
is considered as a constant, and thus the magnetic field density across the center of the coil could be obtained from the magnetic dipole model [37] as

where is the normalized direction vector in y axis,
is the vector from the rotor magnet center to the coil center and r is norm of
According to the diagram of the RTO structure shown in figure 1(b), they are expressed as
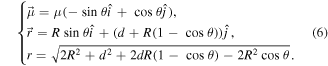
In equation (6), d is the radial distance from the coil center to the trajectory of the rotor magnet center and is the normalized direction vector in x axis, therefore, equation (5) becomes
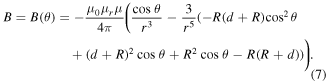
The total magnetic flux density across one coil (combination of four magnets) becomes

Both equations (7) and (8) are complex. The total magnetic flux density in one coil is neither a linear function nor a sinusoidal function of which makes it inconvenient to develop a systematic model and hard to get an insight of the magnetic flux's dependence on
However, figure 3(a) indicates that the total magnetic flux density in one coil can be approximately modeled as a sinusoidal function of
and the period is
(totally four magnets). Since
has not been determined yet, the constant part
in equation (7) was not included in the simulation, but it would not influence the sinusoidal dependence.
Figure 3. Numerical simulation of equation (8) when R = 24.2 mm and (a) d = 24.1 mm, (b) d = 50 mm, (c) d = 10 mm. 24.1 mm is the value of d in the design.
Download figure:
Standard image High-resolution imageNote that, the larger d/R is, the closer to a sinusoidal function Btot is, which could also be concluded from equations (7) and (8). When R/d ≪ 1, equation (7) is approximated as

Substituting equations (9) into (8) yields

Equation (10) indicates that Btot is a sinusoidal function of when d ≫ R. The overall magnetic flux density in one coil is then taken as a sinusoidal function of
in the study, that is,

where k1 is a constant coefficient. Therefore, the electromagnetic voltage is

ku is also a constant coefficient. When the rotor is having small-angle harmonic oscillations, that is, where A and
are the oscillation amplitude and frequency, equation (12) becomes

Equation (13) indicates that the frequency of the output voltage is two times of the rotation frequency when the θ is small, which coincides with our previous findings [36]. The RTO has six coils at 0,
and
The angular differences between them are integral multiples of
(half period of the voltage), therefore, the output voltages from the six coils have either same phases or opposite phases. By connecting the six coils serially, the total output voltage is still a sinusoidal function and equation (12) is therefore used to represent the total output voltage.
In order to verify equation (12) and identify ku, the output voltage is measured at different angular velocities of the rotor and different load resistances, as shown in figure 4, where A DC geared motor is connected to the rotor and the angular velocity is controlled by the power supply and measured indirectly by a laser sensor. All the data are acquired by an oscilloscope.
Figure 4. Experimental setup for measuring the output voltage of the RTO at different angular velocities of the rotor and different load resistances.
Download figure:
Standard image High-resolution imageThe waveforms in figure 5 indicate that the output voltage has approximate sinusoidal dependence on the rotor rotation angle, which agrees with the aforementioned assumption as shown in figure 3(a).
Figure 5. Output voltage waveforms of the RTO at a load resistance of (a) 1 MΩ and (b) 430 Ω, when R = 24.2 mm and d = 24.1 mm. The rotor angular velocity is 29.7 rad s−1.
Download figure:
Standard image High-resolution imageFigure 6. The output voltage Vpp at different rotor angular velocities ωr.
Download figure:
Standard image High-resolution imageThe output voltage across the load resistor becomes:

where Rint is the internal resistance of the coils (internal inductance is negligible). To obtain the value of ku experimentally, the output voltage (Vpp) is measured at different rotor angular velocities, as shown in figure 6. Curve fitting (at 1 MΩ) yields a value of 0.5335 for ku, which is then used for calculation based on equation (14) at (430 Ω). Good agreement between calculation and experimental results occurs at lower rotor angular velocities ( < 30 rad s−1). The discrepancy becomes larger at
> 30 rad s−1 as the inductive reactance of the coils becomes more evident. However, the discrepancy even at larger
is not significant in our study, indicating that neglecting the inductive reactance of the coils is acceptable.
The equation for the electrical circuit is [38]

where the electromechanical coupling coefficient becomes

The conclusive governing equations of the coupled oscillations are then derived as
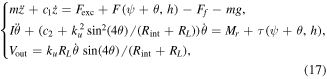
where m is the mass of the RLO, c1 is the damping coefficient of the RLO, z is the absolute translation displacement of the RLO, Fexc is the input excitation force, Ff is the friction force, I is the angular mass of the rotor, c2 is damping coefficient of the rotary oscillation. The electromechanical coupling is included in equation (17). Different from many works that have done in the literature [21, 23, 25, 38], the electromechanical coupling coefficient in equation (17) is not only dependent on the load resistance, but also on the dynamic variable, The strategy here opens a useful way to simplify and identify complex systems in terms of both modeling and experiment. Preliminary guidelines could also be concluded from equation (17). On one hand, when the load resistance is large (close to open-circuit condition), the electrical damping is negligible, which demonstrates that the modeling in [36] is acceptable for a load resistance of 1 MΩ. On the other hand, when the rotor is trapped in the potential wells,
becomes very small, and so does the electrical damping, however, the output voltage is also small. Therefore, inter-well rotation with high-angular velocity is favorable while small-angle intra-well oscillation is unfavorable.
4. Numerical simulations of the dual resonant RROC
Characteristic parameters and their values of the RROC are listed in table 1.
Table 1. Parameters of the RROC.
Parameters | Definition | Value |
---|---|---|
m | Mass of the RLO | 662 g |
c1 | Rectilinear oscillation damping | 3 kg s−1 |
c2 | Rotary oscillation damping | 1 × 10−6 kg m2 s−1 |
Fexc | External excitation | 0.6 g |
Ff | Friction force on the RLO from the steel shafts | 1.48 N |
g | Gravitational acceleration | 9.8 m s−2 |
I | Angular mass of the rotor | 3.02 × 10−5 kg m2 |
R | Distance from rotor center to magnets center | 24.2 mm |
μ | Norm of the magnetic moment of the magnets | 2 × 10−6 A m2 |
μ0 | Vacuum permeability | 1.257 × 10−6 N A−2 |
ku | Electromagnetic voltage coefficient in equation (12) | 0.5335 V s rad−1 |
Rint | Internal resistance | 460 Ω |
4.1. Study of the stand-alone RTO and the stand-alone RLO
To verify the dual resonances and high-energy orbits of the RROC, simulations of the stand-alone rotary oscillation and the stand-alone rectilinear oscillation are performed, respectively. According to equation (17), the rotary oscillation is governed by

Here, nonlinear excitation torque is set as Mr = cMr3. c = 0.6, 1, 1.4 is chosen (figure 7) to study the stiffness effects, while a linear excitation torque is set as Mr = −0.3θ to demonstrate that the nonlinearity of rotary oscillaiton results from the nonlinearity of restoring torque. As shown in figure 7(a), the jump-down frequency increases with the increasing of c, which coincides with the relationship between the natural frequency of a linear resonator and its stiffness, and when the restoring torque is linear, the oscillation is almost linear as well (black line), where the high-energy orbit is not achieved. Figure 7(b) suggests that electrical damping (corresponding to RL) has significant influence on the bandwidth and peak rotary displacement. At optimal load resistance of 465 Ω, the electrical damping is significant and detrimental to the rotary oscillation if broad bandwidth is desired. This should be avoided with careful design of the external circuit. Note that the rotor has intra-well oscillations in this part of simulation, instead of inter-well oscillations.
Figure 7. (a) Open-circuit numerical simulation of equation (18) at Mexc = 0.2 mN m rotary chirp excitation, Mr = cMr3 (c = 0.6, 1 and 1.4) and Mr = −0.3θ. (b) Numerical simulation of equation (18) at Mr = Mr3 and different load resistances RL.
Download figure:
Standard image High-resolution imageThe governing equation of the rectilinear oscillation is

The simulation results are shown in figure 8. One indicated finding from figure 8 is that neither or m has evident impact on the rectilinear resonant frequency although they both determine the equilibrium positions of the RLO. On one hand, the nonlinear stiffness of the restoring force is large when
is small, however, the equilibrium position shifts upward and thus the stiffness at the equilibrium position does not change much. On the other hand, the equilibrium position shifts downward when m increases, and the nonlinear stiffness increases, but since the resonant frequency is the square root of the ratio of stiffness to mass, the resonant frequency does not have significant dependence on
or m. The advantage of such a feature is that the RROC is tolerant to manufacture imperfections. However, significant changes of the magnetic interaction (i.e. changes of the magnets size) will lead to the significant changes of the resonant frequency. A point worth noting is that the friction force Ff is largely dependent on m as the steel shafts and the plastic parts of the prototype have deformations under excitations when the mass of the RLO is not perfectly centered. The measured Ff is 1.48 N at m = 662 g, and Ff is chosen as 1 N and 0.5 N for simulation of m = 500 g and 400 g, respectively.
Figure 8. The simulated results of equation (19) at θ = 0°, and (a) different ψ and (b) different m under 0.6 g rectilinear excitation and at a load resistance of 1 MΩ.
Download figure:
Standard image High-resolution image4.2. Magnetic interaction patterns between the RLO and rotor of the RTO
The essential novelty of the dual resonant RROC is using the contactless magnetic force to couple the rectilinear oscillations and the rotary oscillations, hence it is of great interest to unveil the interaction patterns between the RLO and the RTO, especially at the resonance of the RLO when the RLO has the largest displacement and strongest interaction with the rotor. The numerical simulation of equation (17) is performed for 200 s with a 1 ms interval and the results from 59.5 to 62 s are presented in figure 9.
Figure 9. Selected simulation results of equation (17) under harmonic excitation of 0.6 g and 5 Hz for illustrating the magnetic interaction patterns (pattern 1–4) between the RLO and rotor of the RTO. Here, m = 662 g, ψ = 10°, Mr = Mr3 and RL = 1 MΩ. dθ/dt refers to the angular velocity of the rotor.
Download figure:
Standard image High-resolution imageAccording to the simulation results, four magnetic interaction patterns are categorized. In pattern 1, the restoring torque has an opposite direction to the angular velocity when the RLO is approaching the lowest point and therefore the rotor rotation is reversed to the opposite direction. Although the torque changes the direction before the interaction ends, as we could find a small peak of the driving torque with an opposite direction next to the first peak, the small peak is not able to reverse the rotation again. Therefore, after the interaction of pattern 1, the rotor has reversed high velocity inter-well rotation. Like pattern 1, the driving torque firstly reverses the rotation in pattern 2 because it has an opposite direction to the angular velocity, however, the torque changes its direction and the peak of the second torque is larger than that of the first one, therefore the rotor rotation is reversed back to its former rotation direction. After the interaction of pattern 2, the rotor has high velocity inter-well rotation with the same direction. In pattern 3, the torque has the same direction as the angular velocity and hence the rotation is not reversed but accelerated in the same direction. Even though the torque has several small opposite peaks, they are much smaller than the predominant one and are unable to reverse the rotation but slightly alter the angular velocity amplitude. In this pattern, the angular velocity goes up step by step until the interaction works in other patterns. Pattern 4 is a compromise of pattern 1 and pattern 2 where the torque changes its direction after the rotation is reversed. However, the amplitude of the opposite torque peak is not small enough as that in pattern 1 so that the reversed rotation could maintain, or large enough as that in pattern 2 so that the rotation could be reversed again. It merely decelerates the rotor rotation so that the rotor falls into a potential well and yields large-angle intra-well oscillation. In addition to the four basic patterns of the interaction, there is another case where the interaction is weak, which means the driving torque is small and the rotor angular velocity does not have significant changes after the interaction. The results indicate that the amplitude and the frequency of the output voltage also increase with the increase of the angular velocity of the rotor, as predicted from equation (14). Although the driving torque changes its direction all the time, the restoring force on the RLO always maintains positive and periodic but the amplitude changes. Therefore, the frequency of the rectilinear oscillation is always the same as the excitation frequency (the period is 0.2 s corresponding to the 5 Hz excitation) but the oscillation amplitude changes with time.
As the high-angular velocity of the rotor is maintained or achieved after the interaction pattern 1, 2 and 3, these three patterns are favorable to produce high output voltage while pattern 4 is not. As can be seen from figure 10, results indicate that the interaction between the RLO and rotor of the RTO is a combination of the four basic patterns, which makes the output rms voltage much smaller than the peak-to-peak voltage. Further study to ensure the interaction works at pattern 1, 2 and 3 while avoiding pattern 4 could possibly be a promising approach to enhance the harvesting capacity.
Figure 10. Selected simulation results under harmonic excitation of 0.6 g and 5 Hz, where m = 662 g, ψ = 10°, Mr = Mr3 and RL = 1 MΩ.
Download figure:
Standard image High-resolution imageThe numerical simulation is also performed under frequency sweep and the results are presented in figure 11, which demonstrate the dual resonances from the rectilinear oscillation and the rotary oscillation.
Figure 11. Simulation results under frequency sweep indicating the dual resonances from rectilinear oscillation and rotary oscillation. m = 662 g, ψ = 10°, Mr = Mr3 and RL = 1 MΩ.
Download figure:
Standard image High-resolution image5. Experimental investigation of systematic parameter analysis
The schematic diagram of the experimental setup is presented in figure 12, where the excitation signal generated by Labview is fed into a long stroke electrodynamic shaker (APS dynamics Inc.) via a NI-DAQ. A piezo accelerometer (PCB333B32) measures the input excitation level and a laser sensor (optoNCDT1402, Micro-Epsilon Inc.) measures the rectilinear displacement of the RLO.
Figure 12. Schematic diagram of the experimental setup for the RROC testing.
Download figure:
Standard image High-resolution imageOur previous experimental study shows limited output bandwidth [36], partly because that m was small (347 g) and the interaction between the RLO and rotor of the RTO was weak (ψ = 10°). In this paper, comprehensive study was performed to find out the parametric effect on voltage output (figure 13). When Mr = Mr1, the rectilinear oscillation achieves stable high-energy orbits (the output voltage maintains high amplitudes in the whole region from 2 to 8 Hz), but rotary resonance was missing, which may be due to the fact that the high value of Mr1 shifts the rotary resonance to the higher frequency region (>14 Hz). When the stiffness is smaller, (Mr = Mr2, Mr3, Mr4), the rotary resonance appears evident but jumping up to and falling down from high-energy orbits happen to rectilinear oscillation.
Figure 13. Measured output voltage (2–14 Hz) at Mr = Mr1, Mr2, Mr3, Mr4, where m = 662 g and ψ = 10°.
Download figure:
Standard image High-resolution imageThough the potential energies of those four restoring torques do not have much difference, the output voltages are quite different, indicating diverse dynamics. This could be explained as follows. Since the rotor has small angular mass, its angular velocity and acceleration are sensitive to the restoring torque. Also, the RROC is endowed with nonlinearity, small perturbation and variation of the restoring torque are expected to have significant impact on the nonlinear dynamics. Moreover, the restoring torque determines the equilibrium position of the rotor, and based on analysis on the interaction patterns, it may be concluded that the restoring torque could influence the occurring possibility of each pattern and then the output voltage. For example, when the interaction mainly works on the pattern 1, 2 and 3, but hardly on pattern 4. However, when
the possibility of pattern 4 is high and the voltage amplitude changes sharply as the interaction switches between pattern 1, 2, 3 and 4. To delve into how the restoring torque impacts the interaction patterns, the initial equilibrium values heq and θeq of h and θ are calculated through

Equation (20) gives that when ψ = 10°, the values of θeq are 2°, 3.55°, 3.06° and 3.75° corresponding to Mr1., Mr2., Mr3. and Mr4. By comparing the values of θeq with the results shown in figure 13, one finding is that the inverse correlation between the output stability (from 2 to 8 Hz) and θeq: θeq is small at and the output voltage is stable whereas θeq is large at
and the output voltage is not stable. This order also agrees with that of the potential well depths and restoring torque stiffness as shown in figure 2(b). The explanation is as follows. It has been discussed that there are four angular equilibrium positions of the rotor that are desirable (0, π/2, π, 3π/2) and the other four are undesirable (π/4, 3π/4, 5π/4, 7π/4). When the rotor is around the latter four positions, the driving torque has an opposite direction to the rotation angle and will restore the rotor within the well. Although two repulsive magnets are added to the RTO to remove the latter four equilibrium positions, these four positions are not actually removed but are transformed from stable equilibrium positions into unstable equilibrium positions. As the possibility of the rotor being around the unstable equilibrium positions decreases with the increasing of the potential well depth, the rotor at
is less likely to stay around the unstable equilibrium positions (π/4, 3π/4, 5π/4, 7π/4) than it is at
Therefore, the rotor at
has a higher chance to have inter-well rotations with high-angular velocity and to produce high voltage output in the whole frequency region from 2 to 8 Hz.
Output voltage was also measured at Mr = Mr1,Mr3 and ψ = 0°, 20° to further demonstrate that the RROC could achieve dual resonances in multiple parameter selections. Shown in figures 14(a) and (b), because the initial driving torque at ψ = 0° is zero and is not able to excite rotary oscillations at high frequency region (8–14 Hz), the rotary resonance does not appear but rectilinear resonance appears because the prototype is not perfectly manufactured and ψ is not exactly 0°. In this case, a restoring torque with low stiffness would make it easy to initiate rotation and provide a large driving torque to excite rotary oscillation in low frequency region (8–14 Hz). This explains that Mr3 yields broadband rectilinear resonance while Mr1 does not. Since 20° is close to the unstable equilibrium position at π/4, the rotor would have a higher chance to stay around the unstable equilibrium position, the output voltage in the low-frequency region from 2 to 8 Hz is therefore not promising when ψ = 20°. Dual resonances are observed in figures 14(c) and (d) when is 20°, but the rotary resonance at
is not evident, mainly because
is nearly flat near
as shown in figure 2(a), resulting a loss of nonlinearity of the restoring torque. In summary, to obtain dual resonances
should be chosen as 10° and the restoring torque should be chosen with relatively shallow potential wells
In order to obtain good performance in the low-frequency region from 2 to 8 Hz,
should be chosen as
and the restoring torque should be chosen with relatively shallow potential wells
or
could be chosen as 10° and the restoring torque should be chosen with relatively deep potential wells
The discussion here is made based on the performance under an acceleration level of 0.6 g. The desirable restoring torques would vary with the acceleration level.
Figure 14. Measured output voltage when Mr = Mr1,Mr3 and ψ = 0°, 20°.
Download figure:
Standard image High-resolution imageAs analyzed in the discussion of the rotary oscillations, the large electrical damping would significantly reduce the bandwidth and the peak values of the rotary oscillations. Figure 15 presents the results at a load resistance of 460 Ω. Compared with the results at open-circuit condition, the conclusion could be drawn that the electrical damping has negligible influences on the output in the frequency region from 2 to 8 Hz when the rectilinear resonance dominates, while it has significant unfavorable influence on the rotary oscillation in the frequency region from 8 to 14 Hz. To obtain wide bandwidth benefiting from the dual resonances, external circuits should be carefully designed and the balance between the output power and the bandwidth should be weighed so that desired overall performance could be achieved. The rms power output at a load resistance of 460 Ω is also presented in figure 16. Though high peak-to-peak output voltage (about 40 V at 6 Hz) is obtained, the rms voltage is relatively low (4.2 V at 6 Hz) mainly because the RROC works at a combination of the four categorized interaction patterns as discussed before, the rms output power is therefore not high.
Figure 15. Measured output voltage at a load resistance of 465 Ω when Mr = Mr1,Mr3 and ψ = 10°. The results show that electrical damping is detrimental to rotary oscillation and voltage output.
Download figure:
Standard image High-resolution imageFigure 16. Output power at a load resistance of 465 Ω when Mr = Mr1,Mr3 and ψ = 10°.
Download figure:
Standard image High-resolution image6. Conclusion
In conclusion, comprehensive modeling, numerical simulations, and experimental parameter analysis are carried out to investigate a dual resonant RROC. Simulation results explain the interaction patterns between the RLO and the rotor of the RTO. Both the simulations and the experiments have shown the dual resonances in the low frequency region from 2 to 14 Hz. Experimental analysis of the parameters provides quantitative guidance of the harvester design. Studies show that in order to obtain dual resonances in the low frequency region (2 to 14 Hz), ψ should be chosen near 10° and Mr should be chosen with relatively shallow potential wells, which could be easily achieved by adjusting the relative positions of two repulsive magnets. To obtain higher-power density and broader bandwidth in a much lower frequency region (2–8 Hz), ψ should be chosen near 0° and Mr should be chosen with relatively shallow potential wells, or ψ could be chosen near 10° and Mr should be chosen with relatively deep potential wells. Simulation and experimental results have also shown that the electrical damping has little influence on the output in the frequency region from 2 to 8 Hz where the rectilinear resonance dominates, while it has significant influence (unfavorable) on the rotary oscillation in the frequency region from 8 to 14 Hz.
Acknowledgments
This work was partially supported by the US Office of Navy Research (N000141410230), and partially supported by the Department of Energy ARPA-E (DOE-AR0000531).