Abstract
There is no ideal atomic propellant for ion thrusters. Xenon commonly used as propellant becomes resource-critical in light of electric propulsion commercialization. Combining these considerations leads to seeking alternatives to xenon as propellant. In this review, we summarize the current literature on molecular propellants. We define two classes of molecules, group I and II, comprising diatomic molecules and more complex molecules, respectively. We identify basic properties which a candidate molecule belonging to either group, I or II, should possess in order to be suitable as molecular propellant. We discuss the pits and traps in testing such candidate molecules inside a thruster on the basis of our experiences with iodine (a member of group I) and adamantane (a member of group II). The thruster system needs to be individually adopted for each propellant candidate in order to enable a thorough testing inside the thruster. The same holds for optimizing the thruster's performance when fed with a new propellant because the microscopic processes occurring inside the plasma will differ from molecule to molecule. These circumstances make such testing time-consuming and costly. To accelerate systematic screening of the vast number of molecular species in terms of suitability as propellant, we propose a screening and evolution procedure based on combining chemical engineering and fundamental physical measurements.
Export citation and abstract BibTeX RIS

Original content from this work may be used under the terms of the Creative Commons Attribution 3.0 licence. Any further distribution of this work must maintain attribution to the author(s) and the title of the work, journal citation and DOI.
1. Introduction
Electric propulsion (EP) devices for space applications are very efficient engines providing high specific impulses (Isp) by converting electric energy into kinetic energy of the expelled microscopic particles. Isp is defined as thrust generated by the EP device divided by the propellant mass flow rate used. The exhaust velocities readily achievable for propellant ions of ion thrusters are about ten times larger than the average velocity of atoms being expelled from their chemical counterparts, i.e. about 30 km s−1 compared to 3 km s−1. EP systems such as ion thrusters are now routinely used on various scientific and commercial satellites for orbital maintenance [1, 2]. Besides the classical attitude control task, EP systems have been employed now for several years for orbit raising. In particular, since the introduction of all-electric satellites by Boeing in 2012, orbit raising has boosted further EP development driven by commercial viability [3, 4].
The most mass-efficient EP systems are gridded ion engines and Hall effect thrusters (HETs). The two types of thrusters are the prevailing EP systems on today's commercial market [3, 5]. Both systems provide high Isp values due to the ionization of a propellant and subsequent acceleration of the propellant ions by means of electric fields. The noble gas xenon is commonly used as propellant in ion thrusters. Xenon is a byproduct of air liquefaction. Due to its low mass fraction of 400 ppb in the atmosphere, only large air liquefaction plants can harvest an appreciable amount of xenon. Thus, the demand for xenon does not necessarily determine its supply as the latter is strongly correlated with the demand for liquid air. These circumstances make xenon rare and rather expensive. To provide some numbers, 2000 h of continuous lifetime testing of a larger ion thruster suitable for orbit raising at a Xe gas flow of about 70 sccm requires about 50 kg of Xe. Assuming a Xe price of about US $ 2000 per kg, such a campaign would cost about US $ 100 000 just in terms of propellant costs. The development of such high power EP systems, which require large amounts of propellant, will increase the demand for xenon considerably. Thus, the price is expected to rise strongly in the future. Therefore, the search for alternative propellants is a mandatory task in order to overcome the challenge set by xenon scarcity in the transition of EP from a wallflower to a major player in satellite propulsion.
A perfect propellant combines high atomic or molecular mass, efficient ionization, and high storage density at standard conditions. It is neither corrosive nor hazardous and can easily be brought into the gas phase. Unfortunately, screening the periodic table immediately shows that no atomic propellant possesses all the properties requested. Already considering only the requirements mass, ionization energy, and boiling point given in table 1, all listed chemical elements can be excluded as perfect propellants. The inert gases xenon and krypton need to be stored in pressurized tanks or under cryogenic conditions and, furthermore, possess high ionization potentials. Additionally, krypton reduces the power-to-thrust ratio by around 20% compared to Xe due to its lower atomic mass. On the one hand, lithium, cesium, and bismuth may possess auspicious discharge properties because of their low ionization threshold and high ionization efficiency. On the other hand, they are solids at standard conditions and have to be heated up to fairly high temperatures in order to obtain sufficiently high vapor pressures for operating a thruster. Lithium reduces the power-to-thrust ratio by about 80% due to its low atomic mass. Mercury is poisonous and has a high impact on laboratory safety issues. Furthermore, it may form metallic layers on satellite surfaces. Such unintended metallizations may cause malfunctions on satellite devices. As there is no ideal propellant, the best choice of propellant depends on the demands of the mission and requires compromises. For example, if a very high specific impulse is required, lithium may be a viable alternative to xenon despite its rather poor power-to-thrust ratio [6]. Approaches of choosing the propellant best suited for a specific mission have been described recently by Fazio et al and Tisdall et al [7, 8].
Table 1. Properties of a selection of atomic propellants [9].
Element | Mass (amu) | Ionization energy (eV) | Boiling point (K) |
---|---|---|---|
Xenon | 131.30 | 12.13 | 165.05 |
Krypton | 83.80 | 14.0 | 119.95 |
Lithium | 6.940 | 5.39 | 1590.15 |
Cesium | 132.91 | 3.89 | 958.15 |
Mercury | 200.59 | 10.43 | 629.88 |
Bismuth | 208.98 | 7.29 | 1832.15 |
Considering the lack of suitable atomic propellants, one may strike a bonanza in the field of molecules. In contrast to atomic propellants, an essentially unlimited number of molecules exists. These species range from simple diatomic to highly complex molecules, which easily exceed the known chemical elements in terms of mass per unit, i.e. atom or molecule. First attempts in the field of diatomic molecules have been made when considering the concept of air-breathing EP for low earth orbit (LEO) missions, where a mixture of nitrogen and oxygen is present as residual atmosphere. Recently, it was demonstrated that a radio-frequency ion-thruster (RIT) may in principle be operated using mixtures of nitrogen and oxygen [10]. However, the concept is only of commercial interest in the LEO and defines, therefore, a niche application. Somewhat similar considerations have been made considering CO2 for refueling EP systems in the Mars' atmosphere [11]. Several authors discussed the use of water H2O as alternative propellant [12–15]. While H2O will reduce the power-to-thrust ratio, its handling is simple and safety, especially in case of a manned missions, is not an issue. The suitability of Buckminsterfullerenes (C60) as propellant was investigated by Scharlemann et al and Nakayama et al [16, 17]. Scharlemann et al reported issues concerning the stability of thruster operation. Nevertheless, Anderson and Fitzgerald emphasized that a few groups reported the successful operation of an ion thruster operating with fullerenes [18]. In 2015 a research program for investigating subliming molecular materials has been started at Giessen University. The main focus of the work currently concentrates on diamondoids and iodine [19–21]. Subliming materials offer the advantage of non-pressurized storage. It is attractive for small satellites launched as secondary payload due to the reduced risk for the primary load. However, a general strategy of finding and classifying molecules in terms of their suitability as propellant for EP systems is still lacking.
In this paper, we start off with general considerations in order to classify molecular propellants (section 2). We then describe experimental results obtained from the operation of an RIT with iodine, representative of group I, and adamantane, representative of group II. Considering these two representative molecules we will outline typical challenges in operating electric thrusters with molecular propellants (sections 3–5). Finally, we will propose a fast way of systematically screening molecular species in terms of their suitability as propellant for electric thrusters based on these experiences (section 6). The paper concludes with a brief summary and an outlook (section 7).
2. Classification of propellant molecules
Generally, a crude classification of propellant molecules into two groups can be made as a starting point of such investigations:
- Group I: diatomic molecules, consisting of two atoms, either of the same chemical species or different chemical species.
- Group II: polyatomic molecules, consisting of more than two atoms and various chemical elements.
The following main ionization channels may occur due to electron–molecule collisions in case of a member of group I:
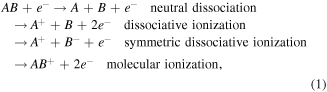
where AB denotes a molecule composed out of chemical element A and B, e.g. molecular nitrogen N2 or carbon monoxide CO, e− denotes an electron, and superfixes + and − characterize the charge state of the atom, if not neutral.
It should be emphasized that the ionization channels given are only a restricted selection of all possible processes which may occur in the collision of an electron with a diatomic molecule. However, this selection comprises, in our opinion, the dominant channels for forming ionized species originating from the molecular state. In case of group I molecules, the dissociation energy is typically lower than the ionization threshold, e.g. in the cases of iodine, oxygen or nitrogen [22]. Hence, a high fraction of ionized species will be a product of a dissociative collision process. For example, Szabo and coworkers reported on the use of iodine I2 as propellant in a 200W HET [23]. They observed that the ionized species in the plume consisted of 97% I+ and a molecular fraction of about 3%. The likely reason for the rare appearance of
is the low dissociation energy of the iodine molecule of approximately 1.57 eV. This value is significantly lower than that corresponding to the typical electron temperature of the thruster's plasma. Furthermore, neutral dissociation requires a subsequent ionizing collision. Thus, this dissociation process has to be classified as an energetic loss channel as electric power is not utilized for ionization. To minimize this kind of loss, an appropriate strategy is seeking diatomic molecules with a neutral dissociation energy as low as possible. It should be much lower than the ionization energy of its atomic fragments, which, of course, should be low in the first place, i.e. Edissociation ≪ Eionization. Already this brief discussion shows that the plasma processes and the underlying plasma chemistry in plasmas consisting of diatomic molecules are much more complex than for plasmas of atomic species. The complex interplay of the processes involved has to be analysed in detail for each molecule in order to judge its suitability as propellant. Performing such experiments using real thrusters is essential for reaching the technology readiness level (TRL) necessary for employing the propellant in space. On the other hand, diagnostics access is rather limited in such thrusters. Thus experiments have to be accompanied by theoretical investigations. For example, a modeling of a gridded ion thruster running with iodine has been conducted by Grondein et al [24].
In case of larger molecules assigned to group II the number of relevant ionization and dissociation channels will be even larger. A multitude of different fragments may occur in the plasma. These fragments henceforth become available for further collision processes. Therefore, it appears desirable to focus on such molecules where dissociation is avoided or at least significantly suppressed, i.e. Edissociation ≫ Eionization. In real systems, dissociation will be inevitable and, thus, such propellants will always suffer from a reduction of the power-to-thrust ratio due to the lower mass of the fragments and the losses resulting from the corresponding fragmentation processes [25]. However, again there is a lack of systematic experimental studies investigating the operation of ion thrusters with such complex molecules as propellant. In contrast to ion thrusters, complex molecules are already in use for jet-propulsion, e.g. JP-10 (Abbreviation for Jet propellant 10) C10H16. While the sum formula is the same as for the diamondoid adamantane, the properties differ strongly, e.g. in contrast to solid adamantane JP-10 is a liquid at room temperature [26].
It would be careless to declare all members of group II as unsuitable alternative propellants, solely on general grounds. The experimental database is too small to draw this conclusion to date. The class of diamondoids as these molecules can be chemically engineered in controlled way [27] will serve as a model system. A strategy for diamondoids is to use a molecule as large and compact as possible. Measurements of the mass spectra using electron impact ionization of adamantane and the heavier diamantane by Waltman and Ling [28] indicate that the stability of this class of molecules may rise by adding more carbon units. Furthermore, the ionization potential is generally reduced with increasing size of the diamondoids as shown by Lenzke et al [29] and may be further decreased by functionalizing the molecule.
3. Test facility and equipment
The test facility used for operating the electric thrusters with molecular propellants is shown schematically in figure 3. A cylindrical tank of 1 m diameter and a height of 0.7 m made of stainless steel was used as vacuum recipient. In case of iodine, corrosive-resistant models of a rotary vane pump of 32 m3 h−1 and a turbo molecular pump of 1400 l s−1 nominal pumping speed were employed in the evacuation process. The pumping system yielded a base pressure of approximately 1 × 10−4 Pa. In case of adamantane, conventional pumps of similar pumping speed were used instead. The vacuum during operation of the thruster was maintained by cryogenic pumping in both cases. The cryogenic surface had an area of 0.24 m2. The pumping speed has been estimated to be roughly 13 000 l s−1 for xenon, adamantane, and atomic iodine. The estimate for molecular iodine has been significantly lower due to the lower thermal velocity caused by the higher mass and was a pumping speed of about 9500 l s−1. Thus, the base pressure with cryogenic pumps was in the lower 10−5 Pa range. The pressure in the recipient was measured by a ionization gauge of the Penning type, typically specified for nitrogen. While the adjustment factors for xenon and iodine are well known [30], the real pressure for adamantane as the dominant species in the recipient could not be determined. Nevertheless, for all measurements with iodine or xenon, the operating pressure was lower than 2 × 10−3 Pa. Therefore, pressure dependent effects on the performance of the thruster should be negligible also in case of adamantane due to the comparable quantities of the propellants used.
The thrusters employed in the experiments were modified RIT-10 possessing cylindrical discharge vessels with a diameter of 10 cm and a height of 5.5 cm. A drawing of the used thruster is shown in figure 1. It resembles the original design for operation with xenon. For both iodine and adamantane the gas resistance was replaced by a model of larger dimensions. The resistance is used to avoid a discharge in the propellant feeding line. The conductivity of the original gas resistance was too low in combination with the vapor pressure of adamantane and iodine of a few kPa to provide sufficient mass flow and, therefore, sufficient neutral gas pressure in the discharge vessel. Two slightly different modifications of the thruster were used for iodine and adamantane, respectively; the main differences are summarized in table 2. Thrusters of the RIT type are further well described in the literature [21, 31, 32]. The working principle comprises two almost independent processes, first, ionization of the propellant gas using a RF-driven coil wrapped around a discharge vessel and, second, electrostatic extraction of ions from the plasma by a system of two or three grids.
Figure 1. CAD-drawing of the major parts of a radio frequency ion thruster.
Download figure:
Standard image High-resolution imageTable 2. Configuration of the thruster assembly for adamantane and iodine.
Propellant | Beamlets | Maximum flow (mg s−1) | Windings of the coil |
---|---|---|---|
Adamantane | 37 | 0.2 | 8 |
Iodine | 241 | 1.2 | 6 |
The RF-coil is powered by a radio-frequency generator (RFG). Modern RFGs, as used in our experiments, are composed of a half-bridge topology operating in the near-resonant mode. They are controlled by a phase-locked-loop/frequency-locked-loop pattern. This approach facilitates zero-current switching of the transistors for high power transfer efficiencies towards the plasma (also known as impedance bridging). The frequency is essentially fixed by the inductance of the thruster and the capacitance of the capacitor-board installed in the generator. A more detailed explanation of the working principle of the RFG can be found in [33].
The extraction system consisted of three grids with a thickness and spacing of 1 mm each. The apertures of screen-grid and grounded grid had a diameters of 4 mm. The apertures of the acceleration grid had a smaller diameter of 3 mm for focusing the ions. Furthermore, the smaller apertures of the acceleration grid reduce the neutral gas loss via the grid system. Further details of the modifications of the RIT-10 for operation with iodine and adamantane are given in table 2.
The propellant supply systems for iodine and adamantane followed the same operating principle, but required different components due to differences in their material properties. The propellant supply systems also have to fulfill the task of evaporating the solid propellant. A heatable MKS 1152c mass flow controller (MFC) provided the iodine flow. The flow was calibrated by a weighing experiment. Changes of weight of the iodine-reservoir over certain periods of time were determined for several fixed set points of the controller. The resulting calibration line is shown in figure 2(a).
Figure 2. (a) Calibration of the mass flow controller for iodine. (b) Verification of the predicted mass flow for adamantane.
Download figure:
Standard image High-resolution imageIn case of adamantane, another heatable MFC of type MKS 1150c was used. The operating principles of both MFCs differ. In case of the former, it is based on a conditioned laminar flow through a pipe inside the MFC and, in the latter, on the choked flow condition. In case of MKS 1150c, one has to make sure that gas lines of sufficient diameter (at least 6 mm or a quarter inch) are used, otherwise the back pressure is too high to fulfill the choked flow condition. The MFC was calibrated by the manufacturer using nitrogen, the predicted flow for adamantane was also provided by the supplier. Again, we verified the correctness of the predicted flow by a weighing experiment. The experimental data were found to be in agreement with the manufacturer's predictions within the error bars of the experiment of ±20% as shown in figure 2(b) together with the theoretical estimates of the manufacturer.
Figure 3. Schematic of the setup for performance analysis and diagnostic of an RIT-10 fed by iodine and adamantane.
Download figure:
Standard image High-resolution imageThe fragments of dissociated iodine and adamantane leaving the plasma vessel of the RIT-10 thruster were measured with a sector mass-spectrometer as shown in figure 3. The spectrometer consists of a double focusing dipole magnet. Slits are located at the focal points of the magnet for cutting out transverse components of the beam. A Faraday-cup is used for detecting the ion-beam current. The readout of the ion currents is performed by a Keithley picoampèremeter 6485. The typical noise of the current measurement is in the order of a few tens fA.
To obtain additional information about the species inside the plasma, in particular, the neutral species, the light emission of the discharge was recorded in the wavelength range from 200 to 1100 nm with a step width of 0.45 nm using a spectrometer of the type HR2000+ from Ocean Optics with a fiber-based light collection. The collector was positioned at the backside of the discharge vessel made of quartz glass. A response correction for the entire optical system was made using a calibrated tungsten lamp.
4. Iodine
Iodine as an alternative to xenon, at least in some mission scenarios such as cubesat applications [34, 35], is currently widely discussed. First attempts to operate a HET [23, 36] as well as an RIT [21] with iodine have been reported. Furthermore, theoretical predictions of the behavior of iodine as propellant for gridded ion thrusters are available [24]. However, still many unknowns remain. Thorough diagnostics of plasma and plume are required in order to determine reliable parameters such as the mass efficiency, which are essential for assessing the performance of the propellant.
Iodine is a diatomic molecule I2 (253.8 amu). It can be stored as a solid at standard conditions. Its density of 4.9 g cm−3 allows compact designs of the propellant storage tank. For example, the amount of propellant required for operating a thruster consuming 0.5 mg s−1 for 104 h can be stored in a spherical reservoir of less than 20 cm in diameter. Iodine can be easily sublimed. At a temperature of 85 °C the vapor pressure already exceeds 2.5 kPa [37]. In principle, heat generated by the thruster may be used to further decrease the demands on heating power for subliming the propellant, i.e. offering a way of minimizing this drawback of iodine being a solid propellant. Moreover, iodine can easily be bound by a cryopump in terrestrial tests of thrusters, temperatures of ≈180 K are cold enough for yielding a sticking coefficient close to 1. Therefore, cryopumping can be realized cheaply by employing liquid nitrogen cooled surfaces. The latter will not work with xenon which requires temperatures below 50 K for achieving sticking coefficients close to 1. Unfortunately, other components of the vacuum system such as turbo molecular or rotary vane pumps need to be protected due to the corrosive nature of the halogen. These circumstances in turn increase costs of testing. Nevertheless, the overall costs for testing thrusters with iodine should be lower than in case of xenon, in particular, when the costs of the propellant itself are also taken into account.
The main graph of figure 4 shows typical performance-mappings of an RIT employing iodine as propellant. Corresponding performance mappings of the same thruster, when xenon is employed instead, are shown for comparison. Further examples can be found in [21]. The performance of the thruster with iodine and xenon is quite similar. The flow unit mg s−1 was used to obtain a better idea about mass-efficiency and specific impulse. In the important low flow regime iodine slightly outperforms xenon, whereas xenon shows better performance at higher flows. The almost parabolic shape of the iodine performance curve is in accordance with the electrical efficiency for a gridded ion thruster modeled by Grondein et al [24]. The same holds true for that of xenon. For a better comparison to other plasma sources, the neutral gas pressure was estimated. The geometric transparency of the screen grid is 38%. An approximation for molecular flow yields a pressure between 0.038 Pa at 0.3 mg s−1 and 0.107 Pa at a flow of 0.6 mg s−1 for the performance curve of iodine at a beam current of 80 mA. The pressure can be linearly interpolated for other flows. For the calculation it was assumed that 70% of the extracted current is caused by I+ ions. Furthermore, it was assumed that 60% of the mass flow leaving the grid system is neutral atomic iodine. While the former fraction is motivated by the results of our mass spectrometry measurement, the latter has to be considered as more or less arbitrary. For xenon a pressure between 0.049 Pa at a flow of 0.3 mg s−1 and 0.126 Pa at doubled flow has been calculated. For iodine as well as for xenon a gas temperature of 400 K was used in the calculation. The RFG power on the ordinate is the DC-power provided to the generator. Measurements of the power coupled into the coil were attempted employing a commercial device Octiv Poly from the company Impedans. However, this measurement is only reliable for a discharge resistance of at least 1 Ω. This condition is fulfilled only at flows exceeding approximately 0.45 mg s−1. In this regime it is found that at least 80% of the input power of the generator is coupled into the coil. The fraction of power which is actually coupled into the plasma remains however unknown. A global model from Volkmar [38] for a smaller ion source of the same type using xenon as propellant yielded a coupling efficiency between 35% and 45% from coil to plasma. Assuming a comparable coupling factor for the thruster used yields that approximately 25%–40% of the applied DC-power may be coupled into the plasma.
Figure 4. Comparision of iodine and xenon performance using an RIT-10 at two different extraction currents [21]. 1500 V are applied to the screen grid and −150 V to the acceleration grid. The chosen RF-frequency is 1.1 MHz. The mass spectra of the iodine beam have been measured with the spectrometer shown in figure 3.
Download figure:
Standard image High-resolution imageThe two smaller graphs of figure 4 depict mass spectra obtained at different operation points of the RIT-10 fed by iodine. It is found that molecular iodine ions contribute strongly to the beam current. More than 30% of the detected ions are molecular iodine ions for a beam current of 80 mA and a flow of 0.3 mg s−1. Thus, the ratio of ionized molecules
to ionized atoms I+ in case of the RIT is much higher than that reported for a 200 W HET of only about 3% [39]. Assuming a homogeneous beam, the considerable fraction of ionized molecules in the beam results in a higher average mass-to-charge ratio of the emitted ions. Thus, the contribution of the ionized molecules leads to a by about 11% higher thrust compared to xenon at the same mass flow and beam-power. Figure 4 also indicates that the amount of molecular iodine is smaller at a higher beam current of 95 mA. Double-charged iodine ions I2+ contribute much less to the beam than the other two ionic species. Only a few per mill of all positive ions of the beam are double charged. Since the spectrometer only detects ions of with a divergence of less than 1° from the beam axis, the results need to be treated somewhat cautiously because the divergence behavior of the different ionic species may differ. However, such a difference of the beam divergence of
and I+ should be rather small. This assumption is based on experiments where the beams extracted from the ion source used was characterized with a array of Faraday cups for xenon and krypton. The beam profiles for both propellants were found to be almost indistinguishable. A possible inhomogeneous distribution of the different species across the beam due to a spatial distribution of the plasma parameters, i.e. electron temperature and density of neutrals, inside the thruster cannot be entirely ruled out. However, the fluctuations are typically considered small in this type of thruster. At least, in most global models describing such thrusters successfully a constant electron temperature and a constant plasma density are assumed inside the plasma vessel [24, 38]. The total uncertainty of the abundance measurement, without considering this possible inhomogeneity, is assessed to be a few percent only.
Because of the importance of the average mass-to-charge ratio for assessing the thrust, we set the focus of our mass spectrometry studies on determining the abundance of the different ionic iodine species and
as function of mass flow and beam current, i.e. of the operational parameters of the thruster. The thrust is proportional to the square-root of the mass to charge ratio. Therefore, a high ratio of
to I2+ is desirable in terms of thrust efficiency. Figures 5(a) and (b) show the variation of the relative amounts of the three ion species, i.e. I+,
, and I2+, in the beam as a function of RFG power and mass flow, respectively. The ordinate axis is plotted on a logarithmic scale. Singly charged I+ and
are the dominant species. At low RFG powers,
is even more abundant than I+, which changes at higher RFG powers where I+ becomes the dominant ionic species. In case of constant RFG power of 50 W, the dominance of I+ prevails over the entire range of mass flows studied. The amount of double charged I2+ is significantly below 1%. However, it shows a strong dependence on both operational parameters. It increases with decreasing mass flow as well as with increasing RFG power. I.e. the increase occurs when the electrons acquire high energies prior to collisions with the ions in case of low neutral densities and large accelerating fields. The crossover between the abundances of
and I+ with increasing RFG power reflects the simultaneously occurring increase of the electron density. It may be anticipated that this variation of electron density also affects the probabilities of indirect and multi-step processes. Furthermore, variations of the RFG-power lead to a change of the electron temperature. When ions are extracted, a higher RF-power leads to a larger ion current. Consequently, the neutral gas pressure decreases. Thus, the electron temperature can be expected to rise due to the larger mean free path between collisions. This claim is confirmed by the results of Volkmar [38], whose simulation results for a smaller ion source driven with xenon showed a rise of the electron temperature at constant flow with increasing beam current. Both electron temperature and electron density influence the ratio of double-charged ions I2+ to singly-charged ions I+. The decrease of the relative rate of molecular iodine ions
with higher RFG-power seems plausible and is in agreement with the results of Grondein et al [24]. For completeness, we state that the total ionic current only exhibits a weak dependence on RFG power. Nevertheless, it shows a decrease by about 20% with increasing mass flow over the range studied. The latter may be explained by a higher neutral gas density in the vacuum recipient with rising flow. This leads to more charge exchange processes or a broadening of the ion beam or both.
Figure 5. Dependence of the relative amounts of the ionic iodine species I+, I2+, and in the ion beam (a) on RFG-power at constant massflow and (b) on propellant massflow at a constant RFG-power. The voltages applied to the grids are constant in both cases.
Download figure:
Standard image High-resolution imageWe also scanned for negative ions in the mass spectroscopic experiment. The rate coefficients given by Grondein et al indicate that several percent of the ions should be negatively charged at electron temperatures lower than 4 eV. However, we could not detect any negative ions I−. A likely reason is that negative ions will be reflected by the floating potential and thus hindered to leave the plasma vessel via the grid system. Therefore, it still may be the case that negative ions contribute to degrading the performance of iodine compared to xenon in the high-flow regime. While our setup for mass spectrometry with the dipole magnet gives precious information about ions, it cannot yield any information about the neutral species I0 and . A residual gas analyzer is no alternative as it requires an additional ionization in the detection process. This ionization process leads to additional dissociation and, thus, the results will not be representative for the plasma inside the discharge chamber. Some information about the neutral species may be obtained by optical emission spectroscopy.
An example of an emission spectrum of the iodine plasma inside the thruster is given in figure 6. The spectrum consists of many sharp lines corresponding to electronic transitions of I0 (spectroscopic notation I I) and I+ (spectroscopic notation I II) on a broad vibronical background originating from . The spectral features lie in the spectral range between UV and near IR. We do not attempt to fully analyse the spectrum. Instead we will focus on the dependence of a few prominent spectral features characteristic for the three iodine species I0, I+, and
. Based on the assumption that the optical transition matrix elements of these features are independent of the operational parameters, we analyse the intensity ratios of pairs of transitions corresponding to two different species in order to assess the evolution of their relative abundance with the operational parameters. The lines and the broader band used in the analysis described are given in table 3.
Figure 6. Emission spectrum of an RIT-10 like ion source with iodine as sole propellant.
Download figure:
Standard image High-resolution imageTable 3. Wavelength λ of the lines and bands used for the analysis of the iodine discharge. The wavelengths as well as the transition states were taken from the NIST database.
Species | λ (nm) | Transition |
---|---|---|
I0 (I I) | 905.83 | 5p4 6p2[3]7/2 ![]() |
I0 (I I) | 973.17 | 5p4 6p2[2]5/2 ![]() |
I+ (I II) | 466.65 |
![]() ![]() ![]() |
I+ (I II) | 467.55 |
![]() ![]() ![]() ![]() |
![]() |
640–650 |
![]() ![]() |
The spectral features chosen are well separated from features of other species. The two chosen lines of I+ cannot be spectrally resolved with the spectrometer used and the sum of their intensities was used in the analysis. The intensities of the two I0 line at 905.83 nm and at at 973.17 nm were determined separately. The integral value of the intensity of the spectral range between 640 and 650 nm was chosen as indicative for because no significant sharp features of the other two species are present in this range.
Figure 7 shows the evolution of the ratios of the intensities of the characteristic spectral features. The data were obtained at the operational points of the performance mapping measured with an extraction current of 80 mA. The open squares denote the ratio of the intensities of the two emission lines assigned to neutral atomic iodine I0. The ratio for all mass flows is constant. This is expected and somewhat justifies the assumption that the transition matrix elements are to a first approximation independent of the operational parameters of the thruster in the range of interest. Furthermore, we find, that the relative amount of I+ decreases with respect to those of the two neutral species I0 and . This finding somewhat indicates that the ionization process is less efficient at higher mass flows, i.e. higher density of neutral iodine, which is expected. Interestingly, the relative amount of neutral molecular iodine
increases compared to that of neutral atomic iodine I0 with increasing mass flow. This curve also follows the trend observed in the performance mapping. There, the performance curve rises again at higher mass flows meaning that more RFG power is required to keep up the same beam current. Thus, the rise of the performance curve denotes that more power is coupled into the plasma and leads to strong vibronic excitation of the molecules.
Figure 7. Ratios of ionized I+, neutral I0, and the bands of (right axis) derived at the same operational points of the thruster as in the given performance curve (left axis). The extraction current was 80 mA and the RF frequency 1.1 MHz.
Download figure:
Standard image High-resolution imageIn conclusion, although iodine is a simple diatomic molecule, obtaining a detailed understanding of the processes going on within an iodine plasma inside a thruster is very challenging. Further thorough mass spectrometric and optical spectroscopic investigations of plasma and plume are required to shed more light on the processes involved. A full understanding will require a careful comparison with theoretical global models of the plasma. Establishing this knowledge is essential for understanding and optimizing the performance of a thruster fed with iodine as propellant at different points of operation. Due to the rather small dimensions of the discharge vessel, invasive diagnostics such as Langmuir-diagnostics for a precise analysis of the plasma parameters are not applicable as they would perturb the plasma. Furthermore, any modification of the thruster has to be avoided to receive data comparable to earlier experiments and to comply with the TRL achieved by previous testing. Supporting experiments need to be designed, but in the long run cannot replace testing with the actual thruster.
5. Adamantane
Diamondoids are a class of molecules CmHm+6 for m ≤ 5, deviations from that sum formula may occur for m > 5. The arrangement of carbon-atoms of a diamondoid molecule resembles a fragment of the diamond crystal lattice [27, 40]. Hydrogen terminates the diamond lattice fragment. The large number of known diamondoids and corresponding derivates in conjuction with the established ways of controlling chemical modifications of such molecules makes this class of rather stable molecules an ideal model system for defining ways of optimizing molecular propellants. Results on the progress of tests using adamantane, which is the smallest diamondoid C10H16 (136 amu), will be discussed.
Adamantane is a solid at standard conditions. It possesses a mass density of 1.08 g cm−3 at 300 K. For comparison, at this temperature xenon would have to be pressurized to about 7 MPa to achieve the same storage density [41]. Adamantane can be brought easily into the gas phase by sublimation. A temperature of about 100 °C is sufficient for obtaining a vapor pressure of 2 kPa [42, 43]. Thus, the demands on heating power can be kept inside acceptable limits.
The threshold energy for electron impact ionization of adamantane is 9.23 eV [29], almost 3 eV lower than that for xenon (12.13 eV). Potentially, the lower ionization potential can lead to a lower power consumption of the thruster. This positive effect is counteracted by energy loss channels arising due to fragmentation of the molecule or its excitation in collisions with electrons. Fragments of adamantane appear when electrons with an energy exceeding 10.6 eV are present in the plasma.
The degree of fragmentation depends on the fragmentation cross sections and the electron energy distribution. Assuming a Maxwellian electron energy distribution function and typical electron temperatures, the amount of molecular fragments can be kept low only, if the fragmentation cross sections of the molecule in ground and excited state are much smaller than its ionization cross section. For example, at an electron temperature of 7 eV only about 6.3% of the total number of electrons possess enough energy to cause ionization without fragmentation of adamantane. However, already about 32% of the electrons possess enough energy to either cause ionization or fragmentation. This typical scenario emphasizes the need for a propellant with a large energy gap between ionization and fragmentation threshold.
The behavior of adamantane as propellant also was investigated. Corresponding performance mappings using an RIT-10 in the configuration described in section 3 are shown in figure 8. The frequency of the RF-generator was set to 1.6 MHz. Performance mappings acquired using the same operational parameters of the thruster, but xenon instead of adamantane, are depicted in the same graph for comparison. The extraction currents used in this comparison were rather low, i.e. 3 and 5 mA. Measurements at higher currents were not possible using adamantane as propellant because of limitations of the equipment. The shape of the adamantane performance curves in figure 8 are comparable to those of xenon. However, the gas consumption as well as the needed RFG-input power are both significantly higher for the same extraction current. In contrast to iodine not even a rough estimate of the discharge pressure can be made reliably in case of adamantane. The thermal velocities of the neutral species expected to appear in the discharge, such as adamantane and hydrogen, differ by more than one order of magnitude. Therefore, without precise knowledge of the relative abundances of the neutral species, an estimate of the pressure suffers from large uncertainties.
Figure 8. Performance-mapping of an RIT-10 for two extraction currents with adamantane and xenon. The RF-frequency is 1.6 MHz [25].
Download figure:
Standard image High-resolution imageThe composition of the ion beam extracted (grid voltages applied) or emitted (grid voltages set to zero) by the RIT-10 fed with adamantane as propellant was studied as function of the operational RFG power and gas flow. A typical mass spectrum of the ion beam is depicted in figure 9(a). It indicates that the ion beam contains a rich amount of fragments of adamantane. As expected, the energy gap between ionization energy of the molecule and dissociation energy of the molecule is not large enough to avoid fragmentation. Furthermore, it indicates that the cross sections for fragmentation to occur in collisions with electrons are fairly high. Fragmentation to a large extent is the explanation of the poorer performance of adamantane as propellant in comparison to xenon (figure 8). Such mass spectra have been analysed in terms of the abundance of the different ionic species. Figure 9(b) shows the relative abundance of the various fragments as a function of gas flow. An increase of gas flow at constant RFG power should lead to a lower mean energy of the electrons and assuming a Maxwellian distribution to a lower electron temperature. A lower electron temperature in turn should decrease the fraction of electrons possessing energies above the dissociation threshold. Thus, one would expect a decrease of relative abundance of ionic fragments with increasing gas flow. We do not observe a clear trend in the relative abundances of the different ionic species in the range of gas flows studied. It appears that the change of electron temperature is too small in this range to cause a clear trend. Furthermore, we varied the RFG power coupled into the adamantane plasma at constant gas flow. The measurement was performed without applied grid voltages in order to avoid instabilities of the ion extraction. The currents are in the order of some 100 pA. In this configuration, the ions are solely accelerated by the potential drop in the sheath, typically a few ten volts. This leads to a rather bad resolution of the mass spectra. As a consequence, not all peaks of the ion species present can be distinguished. Hence, we focused on the behavior of the adamantane ion peak which is well separated from those of the ionic fragments. Figure 9(c) shows the results obtained as a function of the RFG power. A strong drop of the relative abundance of the adamantane ions with increasing RFG power is observed up to 30 W. For RFG powers higher than 35 W, the curve seems to saturate before dropping again slightly at powers above 50 W. A higher RFG-power leads to a higher collision rate of the electrons with neutral gas. Therefore the probability for multiple impact rises. A larger degree of fragmentation is observed as a consequence. The electron temperature Te of the adamantane plasma was assessed to be about (7 ± 1) eV under the operating conditions yielding the data shown in figure 9(c).The electron temperature was deduced from a measurement of the sheath potential, using the mass spectrometer. The flux density of the dipole magnet is measured and used to calculate the momentum of the extracted ions, which in turn can be correlated to the sheath potential Φ. The latter is given by the sum of plasma potential and floating potential

where m is the average mass of the ions and me the electron mass. The density averaged mass of the ions is about 90 amu. A value of Te = (7 ± 1) eV is rather typical for low-temperature plasmas in RITs. Xenon-plasmas in this kind of thruster exhibit Te values between 3 and 5 eV.
Figure 9. (a) Mass spectrum of the ions extracted from an RIT-10 using adamantane as propellant. The RFG power was 33.5 W, and the applied screen and acceleration grid voltages were 750 V and −75 V, respectively. (b) Relative amounts of the different ion species present in the extracted beam as a function of gas flow. RFG power and the applied grid voltages were the same than given above. (c) Relative abundance of ionized adamantane molecules in the ion beam current as function of the RFG-input power. The flow was kept constant at 0.122 mg s−1 and no grid voltages were applied.
Download figure:
Standard image High-resolution imageWe also performed optical spectroscopic studies. An example of an emission spectrum of the adamantane plasma is given in figure 10. In contrast to the optical emission spectra taken from plasma of the simpler molecule iodine, the spectrum of the adamantane plasma shows only a few atomic transitions in the visible and near infrared spectrum on a very broad background. Two lines could be assigned to transitions of atomic hydrogen, furthermore, neutral carbon and traces of neutral xenon are observed. The xenon lines are a consequence of the crossed feeding lines of xenon and adamantane and a minor leakage rate of the xenon valve. However, the intensities of the xenon lines are at least two orders of magnitude smaller compared to the operation with pure xenon. Therefore, we assume that the influence of the minor xenon leakage is negligible. The emission spectrum corresponds to the visual impression. The plasma emission is of a relatively low intensity compared to that of iodine or xenon plasmas and exhibits a whitish color. This appearance is understandable considering the presence of the various molecular fragments in the plasma which all contribute differently to the emission observed.
Figure 10. Optical emission spectrum of an RIT-10 using adamantane as propellant.
Download figure:
Standard image High-resolution imageSummarizing our experiences obtained by studying adamantane as a typical representative of group II candidates for alternative molecular propellants, we have to state that the degree of complexity of the plasma in terms of species present and processes ongoing is considerably larger than in case of diatomic iodine representating group I candidates for alternative propellants. As a consequence, the discharge behavior inside the thruster as well as the properties of the ion beam are extraordinary hard to understand. Further means of characterization are required, in particular, because optical emission spectroscopy is apparently unsuitable for a deeper plasma analysis in case of plasmas of complex molecules with a large degree of fragmentation such as adamantane. If fragmentation occurs to a significantly smaller degree, optical emission spectroscopy will still prove useful for characterization of molecules of group II. Furthermore, the settings of the propellant feed system, the electronics, as well as the grid system of thruster are not necessarily simply transferable when changing to another candidate molecule. This holds for molecular propellants in general. Thus, the efforts required in terms of optimizing the thruster environment in order to enable testing of properties of the molecular propellant are very demanding, implying high costs and a high time budget. This means that a reliable preselection of molecular substances in terms of suitability as propellant is highly desirable and essential for making progress in this research field.
6. Future strategy for finding a suitable molecular propellant for ion thrusters
There are only very few molecules consisting solely of heavy (similar to xenon or heavier) atoms. Hence, fragmentation has to be avoided in case of all molecules of group II, such as adamantane, in contrast to those of group I, such as diatomic iodine. For the assessment of the suitability of a certain molecule as propellant, it would be very advantageous to know its thresholds and, at least, relative cross sections of ionization and fragmentation prior to testing its suitability as propellant inside a thruster. These fundamental parameters are not known for most molecules, especially not for newly synthesized molecules. Furthermore, it should be kept in mind that the synthesis of a novel molecule, which comprises an essential step in an iterative design of molecular propellants, will often yield only small amounts of substance. Thus, not enough material is available for testing the propellant's properties inside a thruster.
We have designed an experimental time-of-flight (ToF) set-up dedicated to determining ionization and dissociation thresholds of molecules by electron collision as a function of electron energy. This set-up only requires a few milligrams of substance to carry out the measurements. Cross sections are measured as a function of the electron energy in the regime of single collisions between electrons and molecules. The measuring principle is the same as that described by Straub et al [44]. Measuring the cross sections for a large range of electron energies is still quite time-consuming, but less time consuming and costly than the machine set-up procedure typically required for adopting the thruster set-up for testing another molecule as propellant. The results obtained for adamantane using the novel set up are compared with data obtained using adamantane as propellant inside the RIT thruster below. We show that the agreement is rather good giving hope that using this novel set-up will increase the speed of systematically screening candidate molecules in terms of their suitability as propellant in order to replace xenon in the near future.
The iterative screening procedure is schematically depicted in figure 11 in the form of a decision tree. The experimental traces shown are ToF mass spectra obtained with the new set up recording the fragments occurring in collisions of adamantane molecules and electrons of defined energy, here, exemplarily shown for electron energies of 15, 20, 25, and 100 eV. Based on such data, we can decide how to modify the adamantane molecule chemically in a controlled way in order to iteratively optimize its properties to serve as propellant. This faster screening is also applicable to a wide range of candidate molecules extending beyond diamondoids and their derivatives.
Figure 11. Strategy for finding a suitable molecular propellant, exemplary shown for adamantane. The experimental time-of-flight spectra show the energy-dependence of the fragmentation of adamamantane for four different electron energies.
Download figure:
Standard image High-resolution imageExemplarily, figure 12 depicts a comparison of two series of mass spectra obtained at the same electron energies. The series on the left correponds to adamantane, C10H16, the series on the right to 1-fluoro-adamantane, a functionalized adamantane molecule, C10H15F. Two observations can readily be made. First, the dominant fragments developing in the series of mass spectra for adamantane, i.e. C6H7 and C7H9, are also prominent in the mass spectrum obtained from the plume of the RIT-10 thruster operated with adamantane shown in figure 9(a). Thus, the approach will shed light on the on processes taking place in the plasma as proposed and will reveal the dominant fragmentation channels. Second, comparing the fragmentation of adamantane and 1-fluoro-adamantane reveals that already the replacement of a hydrogen atom by a fluorine atom changes the fragmentation channels entirely. In 1-fluoro-adamantane the number of significant fragmentation channels is reduced significantly for electron energies up to 20 eV, introducing a almost iodine-like situation with a few dominant fragments only.
Figure 12. Comparison of the flight time spectra of adamantane and 1-fluoro-adamantane at four electron energies.
Download figure:
Standard image High-resolution imageThe results shown in figures 11 and 12(a) are not directly comparable with those in figure 9(a). The latter figure shows a mass spectrum extracted from an RIT-10 using adamantane as propellant. The adamantane plasma inside the thruster originates from multiple collisions with electrons possessing a continuous distribution of electron energies. This distribution can be approximated well by a Maxwell–Boltzmann distribution with an electron temperature Te = (7 ± 1) eV (see section 3). Furthermore, multiple collisions inside the plasma lead to multi-step fragmentation, i.e. also fragmentation of fragments. In contrast, the fragments in the mass spectra shown in figures 11 and 12(a) originate from collisions with electrons of defined energy in the single impact regime, where multi-step fragmentation should not occur. To highlight these differences, we have used the mass spectra obtained with the ToF set-up at specific electron energies (like those in figure 12(a)) to crudely mimick the situation inside the thruster and to clarify the relationship between the mass spectra obtained of the RIT-10 and those measure in the ToF set-up. The mass spectra at specific electron energies were area-normalized and weighted with the corresponding probability according to a Maxwell–Boltzmann distribution of the electrons' kinetic energies with electron temperature Te = 7 eV. All weighted mass spectra were added up. The sum represents the mass spectrum of adamantane ions and fragments exiting the thruster after a single electron-adamantane collision only. No collision-induced fragmentation of the fragments was assumed. The comparison with the measured mass spectrum is shown in figure 13. The measured mass spectrum and the calculation assuming a single electron-adamantane collision only before exiting the thruster (red curve) agree in the types of fragments observed. However, the measured mass spectrum shows higher weights for the fragments at lower masses, e.g. stronger signals of lighter fragments such as CnHx+ with n = 1, ..., 4. The reason for the latter is the multi-step fragmentation occurring inside the plasma. This is further corroborated by the crude calculation assuming three electron-adamantane collisions inside the thruster and no further fragmentation of fragments (blue curve). Three possible collisions is a reasonable number considering the mean free path between electron-molecule collisions and the dimensions of the plasma vessel. This calculation shows a good agreement with the experimental curve in terms of spectral weight for the adamantane-like ions (at about 135 m/q), but overestimates the weights of the intermediate fragments CnHx+ with n = 5–8 whilst still underestimating the weights of CnHx+ fragments with n = 1–4. The reason is that our crude model does not account for fragmentation of the fragments, i.e. in reality some fraction of the heavier fragments CnHx+ with n = 5–8 will be converted into lighter fragments CnHx+ with n = 1–4 by colliding themselves with electrons inside the plasma vessel. Yinon and Bulusu [45] have shown in a detailed study of the fragmentation pathways of adamantane performed by the collisionally induced dissociation method that a fragmentation of the fragments indeed takes place and that at least a dozen different paths need to be accounted for to fully describe the multi-step fragmentation. Nevertheless, the energy-resolved measurements obtained using the ToF set-up and depicted in figures 11 and 12(a) are extremely valuable for assessing the suitability of molecules as propellants as they are indeed suitable for accurately determining the molecule's ionization threshold, its absolute or relative cross sections for ionization, and its energy-dependent fragmentation behavior. Thus, the results allow us to distinguish easily between suitable and unsuitable propellant candidates without having to adopt the experimental set-up for each candidate and requiring a few milligrams of the corresponding substance only.
Figure 13. Comparison of the experimental mass spectrum of the operating RIT-10 and two calculated mass spectra for an electron temperature of 7 eV, assuming either one or three electron-adamantane collisions (which may lead to fragmentation) prior to the ionized adamantane molecule or its fragments leaving the thruster. A possible fragmentation of fragments is neglected.
Download figure:
Standard image High-resolution imageOur objective in the long run is finding a molecule with a large energy gap, e.g. more than 10 eV, between ionization and fragmentation threshold and simultaneously a large ionization cross section. If a substance fulfills previously defined criteria to a promising degree, it will be tested as propellant inside an RIT, otherwise it will be chemically modified. The class of diamondoids and their derivatives is an ideal model system for verifying this screening and evolution procedure based on combining chemical engineering and fundamental physical measurements.
7. Conclusion and outlook
Iodine shows desirable advantages compared to xenon at some operating points of the thruster at low mass flows. While the required mass flow and RFG-power for a certain beam current are comparable, the mean mass of emitted ions is significantly higher compared to xenon. Therefore a higher thrust at the same beam-power and mass flow may be achieved using iodine. Future investigations with iodine should be performed with a thruster that allows larger extraction current densities. We found that the relative fraction of molecular iodine ions drops with increasing RFG-power and therefore electron density. Since the beam current is proportional to the electron density, the performance advantage of iodine is reduced or completely vanishes at typical extraction currents for this thruster size (e.g. 234 mA xenon in case of the Artemis mission [46]). Due to its corrosive nature, the use of iodine on satellites remains a risk. While iodine seems to be a promising candidate, its still no perfect propellant to replace xenon.
As somewhat expected, adamantane is not a suitable propellant yet. However, it can be used to generate a plasma, thus it serve may as a starting point for further studies. It can be functionalized by replacing one of the H atoms by either F or a methyl group. Both substitutions should reduce the threshold for electron impact ionization. Furthermore, increasing the size of the molecule going to the larger diamantane will increase the dissociation energy. In this fashion, the molecule can be iteratively modified and optimized for use as propellant ion ion thrusters. In this sense, the class of diamondoids serves a model system for establishing this strategy.
However, a systematic approach for addressing this challenge of finding good molecular propellants for EP systems requires a fast and cheap screening method for assessing the candidate molecule's suitability as propellant and ways of improving its properties. We propose such a procedure combining chemical engineering and fundamental physical measurements. In particular, we presented an experimental approach how the ionization and dissociation thresholds of the candidate molecule can be directly determined requiring a few milligrams of the substance only in contrast to a few tens of grams for testing it as propellant inside a thruster. Of course, if a likely candidate is identified, it will need to be tested inside a thruster. Such testing then requires a careful adaption of the thruster and its periphery tailored to the specific properties of the molecules. The requirements on the thruster system differ from molecule to molecule as demonstrated for adamantane and iodine. The same holds for optimizing the thruster's performance when fed with a new propellant. The optimization of the thruster's performance with a new propellant requires a thorough understanding of the microscopic processes occurring inside the plasma. In case of molecular propellants, the number of microscopic processes, which need to be accounted for, is much larger than in case of an atomic plasma. The complexity of the processes inside a molecular plasma demands a great deal of plasma and beam diagnostics as well as of corresponding global-model descriptions. Despite all challenges, there is a good chance of finding the 'needle in the hay stack' in the near future. It may not be a single molecular propellant for replacing xenon in all possible mission environments, but one may anticipate molecular propellants optimized for specific mission environments. Surely, efforts employing iodine on cubesats or efforts and considerations of using atmospheric gases in low Earth or Mars orbits point in this direction.
Acknowledgments
This work has been supported by the Federal Ministry for Economic Affairs and Energy under contracts 50RS1502 and 50RS1709.