Abstract
Naturally contaminated basil seeds were treated by a surface dielectric barrier discharge driven in the humid air by an amplitude modulated AC high voltage to avoid heat shock. In order to avoid direct contact of seeds with microdischarge filaments, the seeds to be treated were placed at sufficient distance from the surface discharge. After treatment, the seeds were analyzed in comparison with control samples for their microbial contamination as well as for the capability of germination and seedling growth. Moreover, chemical modification of seed surface was observed through the elemental energy dispersive x-ray analysis and wettability tests. We found that treatment applied at 20% duty cycle (effective discharge duration up to 20 s) significantly decreases microbial load without reducing the viability of the seeds. On the other side, seedling growth was considerably accelerated after the treatment, and biometric growth parameters of seedlings (total length, weight, leaf extension) considerably increased compared to the controls. Interestingly, scanning electron microscopy images taken for the different duration of treatment revealed that seed radicle micropylar regions underwent significant morphological changes while the coat was substantially undamaged. Inside the seed, the embryo seemed to be well preserved while the endosperm body was detached from the epithelial tegument. A total of 9 different genera of fungi were recovered from the analyzed seeds. Scanning electron microscopy images revealed that conidia were localized especially in the micropylar region, and after plasma treatment, most of them showed substantial damages. Therefore, the overall effect of the treatment of naturally contaminated seeds by reactive oxygen and nitrogen species produced by plasma and the consequent changes in surface chemistry and microbial load can significantly improve seed vigor.
Export citation and abstract BibTeX RIS
1. Introduction
Seed-borne pathogens represent one of the main problems affecting the biological quality of seeds and crop production. Seed quality is determined by their capacity of germination followed by vigorous growth of seedlings, as well as by their resistance when exposed to any environmental stress. Stress imposed by biotic factors, such as bacterial and fungal infections of seeds, constitutes a threat to their regular development and growth. Particularly, seed-borne microorganisms, infecting or contaminating the seed either in the field or during storage, can greatly affect seed quality and safety and cause severe crop yield losses. They include plant pathogens, which can be responsible for seed rot, reduced seed germination, seedling damping-off and plant diseases on several economically important crops [1].
Chemical treatment of seeds by applying dressing with antimicrobials can significantly contribute to the containment of seed-borne pathogens [2] with moderate costs and relatively low environment impact because of the small amount of active substance. However, effective chemicals are not available for all seed-borne pathogens (e.g. the oomycete Peronospora belbahrii Thines, agent of downy mildew of basil [3] and various bacteria, such as Xanthomonas campestris pv campestris on seeds of Brassica spp.). Furthermore, synthetic chemicals cannot be used in organic farming (i.e. Council Regulation (EC) No 834/2007), hampering the achievement of high-quality standards for organic seeds and hence alternative control approaches are necessary [4].
Over the last few years, physical treatments of seeds, including both traditional (i.e. hot water treatments) or innovative methods (i.e. seed irradiation), and the use of biocontrol agents, natural compounds and plant extracts have been proposed or re-evaluated as alternative approaches to treatments with conventional chemicals [5]. Simple physical approaches, such as the heat treatment (essentially using hot water), can significantly reduce infections by seed-borne pathogens but the establishment of precise treatment parameters is crucial to avoid adverse effects on the germinability and emergence of seeds [6, 7].
There have been several attempts in the past to use the electrical field in order to improve seed germinability and vigor of plants. A pioneer in this field was Bindo Riccioni back in the 1930s. He designed and patented a device able to generate fast varying electrical fields [8] able to produce atmospheric pressure plasmas and conducted a detailed study of electrical seed treatment with field-testing in 23 provinces of Italy [9]. Evidence that low-pressure plasma treatment can improve the rate of water uptake as well as germination of hard seeds was provided during investigations conducted in the USA and reported in several papers [10–13], but no details about the microbial load of seeds were reported.
Recently, innovative tools such as atmospheric pressure dielectric barrier discharges (DBD's) were tested as a viable route in order to reduce the microbial contamination of seeds and to enhance the growth of plants [14–25]. The low-temperature non-equilibrium plasma produced in air at atmospheric pressure has attracted the attention of different research groups as an effective source for the production of reactive oxygen species (ROS) and reactive nitrogen species (RNS) and their application to biological systems [26, 27].
Radical ROS (such as , OH, HO2, etc) and non-radical ROS (such as H2O2, O3, 1O2, ONOOH, etc) are involved in the regulation of several biological processes (growth, development, and responses to biotic and/or abiotic stimuli) and their possible role as oxidant agents in the inactivation of microorganisms has been shown [26, 28]. Moreover, ROS and RNS are known to activate the hypersensitive response (HR) and other events related to the plant pathogen response system [29–32]. Therefore, the DBD plasma treatment of seeds represents an interesting solution for organic farming as well as an alternative control method to classical chemical applications. The described effects of non-thermal gaseous plasmas to microbial decontamination on seeds together with the consequent increase of vigor and growth of the derived seedlings compared to controls have been already reported [21, 23, 33]. However, due to the possible synergy of reactive species, UV photons and electric field, more systematic studies are necessary to reveal the full potential of low-temperature non-equilibrium plasmas at atmospheric pressure in the field of plant sustainable protection and growth.
Recently, we studied surface DBD (SDBD) to produce ozone and excited species [34, 35]. In the case of amplitude modulated High Voltage (HV) systems, the SDBD produced ozone at a quite low cost in the dry synthetic air in the energy density range from 10−4 to 10−1 Wh l−1. In addition, the O3 concentration under such conditions exceeded the NyOx concentration by more than one order of magnitude, implying that the system was controlled by the ROS dominated chemistry. In this work, we used SDBD configuration driven by an amplitude modulated AC high voltage at atmospheric pressure to produce various reactive species in humid air. This device has been applied to treat sweet basil seeds (Ocimum basilicum L.) through various exposure conditions avoiding however direct contact of seeds with plasma filaments. After treatment, we observed the response of seeds through the overall microbial content, surface chemical modification, and seedling growth behavior.
2. Experimental section
2.1. Discharge system
The discharge system included the modified SDBD reactor used to study the production of active species [34–36], gas feeding unit, discharge energization system, electrical and optical diagnostics and sample holder. The SDBD reactor shown in panel (a) of figure 1, consisted of a planar SDBD electrode system placed in a PVC chamber equipped with gas feed input/output ports and a high voltage (HV) interface. In comparison with our previous SDBD reactor design [36], this reactor chamber allowed the insertion of a sample holder (figure 1(d)) next to the SDBD surface. The electrodes were deposited on both sides of a thin alumina substrate using the screen printing technique [34].
Figure 1. Experimental setup for the treatment of seeds: (a) sketch of modified SBDB reactor with sample holder; (b) sketch of the SDSB electrode geometry with metallic ground (GND) and high voltage (HV) electrodes deposited on opposite sides of the alumina plate; (c) bottom view of the discharge without sample holder inserted; (d) picture of the sample holder with seeds distributed over an alumina disc pad and glass tube spacer for fixing the distance between the SDBD and seeds; (e) snapshot of the basil seeds exposed to the SDBD in air taken through the side optical viewport.
Download figure:
Standard image High-resolution imageThe nickel-based electrode exposed to the discharge consisted of 17 parallel strips (1 mm wide, 75 mm long and separated by 3 mm). A silver-based induction electrode (74 mm × 74 mm) covered the opposite surface of the alumina plate. The SDBD was powered by an AC power supply composed of the TG1010A Function Generator (TTi), Powertron Model 1000A RF Amplifier and a high-voltage step-up transformer. Applied AC HV waveform (5 kHz) was amplitude-modulated by a square-wave modulation waveform (fM = 500 Hz) producing 5 kHz sine-wave TON and TOFF periods with a fixed duty cycle D = TON/(TON + TOFF) = 20% and a precise number of sine-wave bursts was selected through an external trigger realized by means of a TG 5011 Function Generator (TTi). A LeCroy LC534A digitizing oscilloscope (bandwidth 1 GHz, up to 2 GS/s) was used to record the voltage-charge, voltage–current characteristics of the discharge. The discharge voltage waveforms were sampled using a Tektronix P6013A high voltage probe (1000:1@1 MΩ, bandwidth 25 MHz). A voltage probe (10:1@10 MΩ, bandwidth 1 GHz) was used to measure the voltage drop on a current sensing shunt resistor (R = 10 Ω) or on a transferred charge measuring capacitor (C = 0.5 µF), both inserted alternately between the induction electrode and ground. The reactor was fed with humid air at a fixed flow rate of 7 slm.
2.2. Discharge products
The SDBD discharge products were analyzed by means of non-dispersive UV absorption ozone monitors API 450 and API 450 M, chemiluminescence NO/NOX analyzer API 200EM and infrared N2O analyzer API 320E (all Teledyne Instruments). Stable discharge products were quantified without biological materials inserted in the chamber.
2.3. Seed management, water imbibition, germination and seedling growth tests
Commercially available dry seeds of sweet basil, not previously treated with any chemicals, were uniformly distributed on the alumina disc pad, inserted into the SDBD reactor and exposed while maintaining a fixed flow of air through the chamber (figure 1). Exposure times varied from 10 s up to 600 s. Both treated (exposed with the discharge ON) and controls (exposed with discharge OFF) seeds were then sowed in three different environments: soil, sterile wet paper, and agarized medium for in vitro culture. For statistical elaboration at the least 4 batches of 25 seeds were used for each experiment.
Time-lapse photography was performed to estimate the water holding capacity of treated versus control seeds. Variation of seed dimensions was used as an evidence of the enhancement of water imbibition.
Autoclave-sterilized Whatman 3MM filter paper discs were layered in each of a 6-well cell culture plate (5 cm diameter) and thereafter wet with sterile distilled water. Seeds, after each plasma treatment and relative control, were placed on the paper under aseptic conditions in a laminar flow hood. Plates were kept as above. A time lapse picture recording (every 1 min) was taken with a digital camera (13 Mpx) for up to 8 d to compare emergence and growth of treated and control seeds.
Germination was evaluated by the mean time to germination (MTG) defined as:
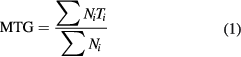
where Ni is the number of newly germinated seeds at time Ti recorded as days after imbibition. Germination rate (GR) was calculated as the inverse of MTG and expressed as seeds d−1 [37].
Plastic pots filled with a mixture of sterilized soil (50%) and commercial peat moss (50%) were watered and kept after sowing in a growth chamber at 24 °C, with a 1000 lux light intensity for 16 h d−1. The average time of cotyledons emergence was timely recorded and, after an observation period of 40 d, seedlings were submitted to biometric measures (total length, weight, root length, the longitudinal extension of first true leaves set).
2.4. Scanning electron microscopy (SEM) and energy dispersive x-ray (EDX) analysis
The microscopic observations were taken by a Hitachi TM3000 SEM (at 10−4 Pa pressure and 15 kV acceleration voltage) in either Observation or Analysis mode. For the best image results, the samples were previously gold-palladium sputter coated for 120 s (at 10−1 Pa pressure, 10 kV acceleration voltage at 15 mA) by an Edwards Sputter Coater. The microanalysis data were gathered by the Oxford Instruments NanoAnalysis SwiftED3000 X-Ray MicroAnalysis System included in the TM3000 at the conditions of microscopic observations, both by energy dispersive x-ray (EDX) Spectrum View and ID acquisition area on rectangle areas ranging between 0.032 and 1.20 mm2.
2.5. Microbial contamination and statistical analysis
Treated and control seeds were placed on water agar medium (WA; 7 g of agar Oxoid No. 3 per liter of distilled water) under aseptic conditions in a laminar flow hood for the characterization and quantification of naturally-occurring seed-borne fungi. Sealed dishes were put in a growth chamber (same conditions as above). In some cases, if a longer growth period was needed to observe seedling development, agar was supplemented with sucrose and micro- and macro-elements to improve photosynthesis and seedling elongation [38].
Taxonomic identification to the genus level of fungal colonies from treated and control seeds was carried out by microscopic observations (Leica MZ 12.5 and Leica DM2500) after 3–10 d of incubation. Percentages of contaminated seeds were determined. Data, transformed into angular values (arcsin √ x), were submitted to the analysis of variance (ANOVA) and mean values were separated with the Duncan's Multiple Range Test [39].
3. Results and discussion
The SDBD discharge was operated at atmospheric pressure in humid ambient air. In order to avoid thermal shock which might be destructive, the driving AC voltage (fAC = 5 kHz) was kept as low as possible to ensure homogenous distribution of microdischarges over the whole SDBD surface. Figure 2 shows typical charge-voltage characteristics and energy delivered during the HV burst composed of two AC cycles at a working voltage of 8.6 kVpp (peak-to-peak). The repetition frequency of applied HV bursts was 500 Hz implying a duty cycle of 0.2 and an average power of 6.5 W (corresponding to a surface power density of 80 mW cm−2, or energy density of 1.5 10−2 Wh l−1). At given applied energy density, the SBDB fed with air works in the ozone mode, i.e. concentration of O3 produced by the discharge (typically 200–300 ppm at reasonable production yield of 70–80 g kWh−1) exceeded significantly concentrations of nitrogen oxide products (typically 30–40 ppm of NO2 and 2–3 ppm of N2O) [34].
Figure 2. Typical electrical characteristics of the SDBD: (a) voltage waveform; (b) voltage-charge Lissajous figures for VAC = 8.6 kVpp; (c) energy dissipated during single AC burst composed of two AC cycles. The discharge produced in humid air at atmospheric pressure and fixed flow rate of 7 slm.
Download figure:
Standard image High-resolution imageIt is also worth pointing out that the real discharge 'ON' period was significantly shorter compared with the duration of a single AC cycle (about 50% of its duration). Therefore, considering a driving HV duty cycle of 20%, an effective discharge ON time was only a fraction (0.1) of the whole total exposure time.
In order to fix optimum conditions for the treatment of seeds, we initially performed a parametric test varying both the treatment time and distance of seeds from SBDB surface, maintaining other parameters unchanged. In figure 3, the picture shows samples obtained when fixing different exposure conditions and illustrates the effects of the treatment on in vitro seed cultures (photographs were taken 24 d after sowing plasma treated/control seeds in WA). Each vial contained an equal number of exposed seeds placed on WA. The appearance of fungal colonies, often associated with changes in colors (light to dark brown) and clearness of the culture medium, evidenced the presence of still vital microbial contaminants on seeds after treatment. In panel (a) of figure 3 samples exposed to the SDBD products, for various combinations of treatment time (vertical axis) and distances (horizontal axis) from plasma, are reported. The most efficient treatment in term of microbial contamination reduction was the combination of the plasma-to-seed distance d = 3 mm and treatment time greater than 1 min. This is clear in panel (b) that reports typical results of treatment at a distance of 3 mm and 1 min total exposure time. In panel (c) typical results for control seeds evidence the wide development of fungal colonies covering all the agar surface and inhibiting the seed germination and consequent growth of seedlings.
Figure 3. Effects of treatment on in vitro seed cultures for different distances and treatment time in SBDB reactor: (a) samples exposed to SDBD products for various combinations of treatment times (1, 3, 5 min) and distances (3, 4, 5 mm); (b) sample treated at a 3 mm distance from SDBD surface for 3 minutes; (c) control sample.
Download figure:
Standard image High-resolution imageWe used scanning electron microscopy (SEM) to analyze possible morphological changes in treated and control basil seeds. Typical images obtained are reported in figure 4. In the first column, the whole seed structure is reported. The external seed coat does not show evident morphological changes with the remarkable exception of the micropylar region (where the radicle emerges).
Figure 4. SEM Images of the seed coat surface (left column), detail of radicle micropylar region (middle column) and cross section (right column) of basil seeds after different conditions: (a)–(c) control, (d)–(f) treatment time 20 s, (g)–(i) treatment time 200 s.
Download figure:
Standard image High-resolution imageIn this region, the surface was apparently affected by damages induced by the etching processes due to plasma [40] (erosion of the basic structure) which were more pronounced with increasing treatment time (figures 4(e) and (h) versus (b)). This could be evidence that the emergence of radicle should be less difficult as the effect of cracking in external tegument. Images of the cross section of the seed show also a different degree of dehydration in treated seeds (figures 4(f) and (i)) compared to the control (figure 4(c)). The loss of water or an osmotic shock could have caused the apparent discrete separation of internal and external coat layers from the endosperm.
Water uptake by treated basil seed was more efficient and faster than in the case of control seeds. In figure 5, the typical behavior of control and treated seed is shown. Control basil seed surface (figure 5(a)) demonstrated a higher hydrophobicity compared to the treated one (figure 5(b)).
Figure 5. Effect on seed surface of plasma treatment: control basil seed surface (a) shows a higher hydrophobicity compared to treated one (b) (photo taken immediately after applying a water droplet of 1 µl). Panel (c) shows the difference in seeds size before and after treatment. Photographs inserted in panel (c) were taken 2 min after sowing them on wet sterile paper.
Download figure:
Standard image High-resolution imageIt is evident that after the treatment the water droplet spread over a larger seed surface with significantly lower contact angle. The water holding capacity was followed by using time-lapse photography in order to evaluate water imbibition. We observed an increase by at the least 20%, as shown in figure 5(b), where the average dimension of statistically significant samples is reported together with the picture of the seeds (control/treated) taken at the same instant. The faster imbibition of treated seeds, with a consequent average diameter increase (figure 5(c)), can lead to a quicker seedling emergence and faster seedling growth, that could cause escaping from infection by pathogens.
Elemental microanalysis was performed through EDX measurements to reveal chemical modifications of the surface due to the treatment by the SDBD plasma. The analyzed areas are highlighted in figure 6 while EDX results are shown in table 1. The main relevant difference consisted in a variation of the oxygen to carbon ratio which becomes more evident in the region of the emergence of the radicle and a small increase in the content of potassium. These changes suggest that the DBD air plasma produced an important oxidation of the external layers of seeds. The oxidizing character of the air plasmas is an expected effect due to the presence of ROS species produced in the discharge.
Table 1. EDX elemental microanalysis (weight percentage) of treated and control seeds
Analysed area | K | C | O | O/C ratio | |
---|---|---|---|---|---|
Treated | 1 | 2.4 ± 0.1↑ | 41.9 ± 1.1 ↓ | 53.6 ± 1.1↑ | 1.27 |
Control | 1 | 2.0 ± 0.1 | 42.5 ± 1.3 | 52.5 ± 1.2 | 1.23 |
Treated | 2 | 4.2 ± 0.1↑ | 33.9 ± 1.5 ↓ | 59.6 ± 1.4↑ | 1.75 |
Control | 2 | 3.6 ± 0.1 | 36.6 ± 1.6 | 56.5 ± 1.4 | 1.54 |
Treated | 3 | 4.5 ± 0.1↑ | 31.2 ± 1.2 ↓ | 63.2 ± 1.1↑ | 2.03 |
Control | 3 | 2.7 ± 0.1 | 36.1 ± 1.6 | 56.3 ± 1.5 | 1.56 |
aAcquisition areas 1, 2 and 3 of 0.086, 1.20 and 0.032 mm2, respectively.
Figure 6. EDX analysis was performed in three different areas of the seed surface as shown in the figure.
Download figure:
Standard image High-resolution imageThe observed higher content of oxygen on the tegument surface can influence several biological processes (growth, development, and responses to biotic and/or abiotic stimuli) [41]. Moreover, the increase in potassium, maybe due to diffusion of this cation from the interior of the seed coat to the surface, can be linked also to the changes in the chemistry of the seed coat surface. It should be noted that even if not detected by EDX other product of the discharge are RNS whose roles in the germination and faster growth could be related to a combined chemistry of the potassium [42, 43].
Germination of basil seeds on wet sterile paper (see figure 7) was recorded through time-lapse photography. The germination process of treated seeds was faster than the control: the rupture of the external tegument (figure 7(a)), the endosperm swelling and emergence of the radicle (figure 7(b)), the emergence of the cotyledons (figure 7(c)) and the complete emergence of the cotyledons (figure 7(d)) with the formation of the seedling took place on average in about 25% shorter time compared with control (figures 7(e)–(h)).
Figure 7. Time lapse photographs of treated (a)–(d) and control (e)–(h) seeds taken at the same time. Times of observation are: 18 h (a) and (e); 22 h 30 min (b) and (f); 41 h (c) and (g); 50 h (d) and (h).
Download figure:
Standard image High-resolution imageFigure 8 reports the average germination rate for plasma treated seed (calculated from four replications of 25 seeds) compared with the correspondent control samples. The seeds were considered to be germinated when radicle emerged from the seed coat. The graph shows that under aseptic conditions and using only water the germination process was about 25% faster. Germination of seeds was not affected by the plasma treatment in the time range analyzed here and is on average about 92%. Germination of control seed on wet sterile paper is slightly smaller reaching about 85%. In our experimental conditions, the GR calculated as defined by equation (1) for the control seeds was 0.8 d−1, a value comparable with the literature [44], while the calculated rate for the treated seeds is 1.1 d−1.
Figure 8. Effect of plasma treatment on seed germination of basil seeds after plasma treatment of 300 s (treated) compared to the control. Figures are the average of four replicates and bars represent the standard error. Values with different letters are statistically different at the probability levels P = 0.05 (small letters) or P = 0.01 (capital letters).
Download figure:
Standard image High-resolution imageGrowth test in soil confirmed that the overall growth of seedlings was quite enhanced and at 40 d the elongation and weight were 20% higher in treated than in the control seeds (see table 2). Nevertheless, in this case, the average germination rate decreased in this case to 64% and 49% for treated and control seeds, respectively.
Table 2. Average biometric data of seedlings
Total length | Leaf longitudinal extension | Number of leaves | Weight | |
---|---|---|---|---|
Control | (14.2 ± 0.8) | (9.9 ± 0.8) cm | 8 ± 1 | (3.4 ± 0.7) g |
Treated | (18.3 ± 2.6) cm | (11.2 ± 1.0) cm | 8 ± 1 | (5.5 ± 0.8) g |
Treated/control | +22% | +11% | +0% | +39% |
aDetermined after 40 d in a growth chamber at 24 °C, with a 1000 lux light intensity for 16 h d−1.
Results drastically change when seeds are grown in water agar where the development of fungal colonies inhibits the growth of control seeds. In the last case while treated seeds maintain a germinability rate ranging from 70 to 90% (depending on treatment times) the germinability of control seeds drops to less than 50%.
Seed tegument surface usually contains a certain microbial load. When exposed to oxidant agents, such as ROS produced by the plasma discharge, inactivation of microorganisms and the activation of plant defense responses can occur. Preliminary experiments to explore this aspect were performed. To evaluate the original sanitary status of seeds (i.e. their native microbial contamination), control samples of seeds were placed in Petri dishes containing WA. Basil seeds used in this study were naturally contaminated with many saprophytic fungi. A total of 9 different genera of fungi were recovered from the analyzed seeds. Alternaria was the most frequent (82%), followed by Rhizopus (3%), Penicillium (3%), Aspergillus (2%), Botrytis (1%) and others (1%).
Plasma treatments reduced significantly fungal contamination, especially at the longest treatment time (figure 9). It is worth noting that after a treatment of 300 s, the contamination of seeds is mainly to be ascribed to Alternaria sp. spores, that are by far the most difficult to kill.
Figure 9. Effect of plasma treatments on naturally contaminated basil seeds after different exposure time (3 s up to 300 s) as compared to the control. Values with a same letter on the figure are not statistically different at the probability level P = 0.05. Bars represent the standard error.
Download figure:
Standard image High-resolution imageIt is worth noting that the treatment apparently improves the germinability ratio with respect to the control in cases of high contamination of seeds. We generally observed that the development of seedlings was stopped by the faster growing microbial colony in the case of control samples while some of the treated ones (treatment time 3 min) could grow regularly after 7 d (about 30% of seedling survived).
Figure 10(a) shows details of control basil seeds evidencing the presence of fungal conidia like those of Alternaria spp., close to the radicle micropylar region. Filamentous structures attributable to hyphae were also observed (figure 10(b)). The plasma seems to induce morphological changes of fungal structures on the seed surface as shown in figure 10(c) where it is clearly visible the residues of a destroyed conidium close to a damaged one.
Figure 10. SEM images of basil tegument seed surface in the proximity of radicle micropylar region. Fungal structures on basil seed before ((a) conidium; (b), mycelium) and after ((c), altered conidium) plasma treatment.
Download figure:
Standard image High-resolution imageThere are several possible mechanisms to explain the microbial inactivation by non-thermal plasmas [45], that can be of either biological or physical nature. Biological mechanisms include DNA damage by UV radiation, lipid peroxidation, protein modulation and ROS-induced apoptosis. Physical mechanisms are mainly related to phenomena induced by electric fields such as electrostatic disruption (due to the accumulation of charged species on spore surfaces) and electroporation (caused by the direct action of external high electric fields). Damaging of the cell's surfaces resulting in a loss of membrane integrity, leakage of intracellular components and ultimately focal dissolution of the cell surface was demonstrated in a floating electrode DBD discharge [46, 47]. More recently, it has been demonstrated that chemical etching process is the main mechanism leading to the removal of organic materials from surfaces treated by air DBD [40]. The reported etching rates of about of 5 nm s−1 of the plasma treatment, even though the chemistry of etched surface can strongly influence this rate [40]. This means that complete removal of spores via etching will depend on the thickness of the cell walls of the conidial species. Studies of the fine structure of conidia by electron microscopy reported that the fungal spore walls thickness can vary from species to species and depends also on the age of the conidia. In particular, it can vary from few tens of nanometers (as in the case of Penicillum spp. [48]) up to more the 500 nm as in the case of Alternaria spp. [49] (melanized and multicellular spores) and Rhizopus spp. [50], with intermediate values of some hundreds of nm for Botrytis cinerea [51] and Aspergillus [52]. The physical characteristic of the Alternaria spp. can explain why we found it to be one of the more resisting spores to plasma treatment.
4. Conclusion
Basil seeds were exposed to ROS and RNS produced by an SDBD at low mean energy density (1.5 × 10−2 Wh l−1) and without direct contact of seeds with plasma filaments. The exposed seeds were analyzed for their microbial load, capability of germination and seedling growth, morphologic and chemical modifications of the seed coat.
SEM images showed that the morphology of the seed coat is almost completely preserved with the remarkable exception of the radicle micropylar region, where also a change in chemical composition was recorded by EDX technique. The ratio of C/O content increased by 40% for a total treatment time of 5 min. A small increase of potassium was also observed after plasma treatment. Furthermore, plasma treatment significantly modified wettability of the surface which is evidenced by an increased diameter of seeds immersed in sterile water (20% more after 10 min).
Moreover, the used plasma treatment does not influence the germinability of seeds due to the fact that the discharge is working in power modulated mode and therefore seeds are not exposed to any thermal stress. On the other hand, the seedlings have more chance to survive and improve their growth because the microbial charge is significantly reduced and a faster growth is exhibited.
We conclude that exposure of contaminated seeds performed without direct contact with microdischarge filaments can significantly improve seed viability. A faster development of seedlings can be of importance and might improve their survival in soil by escaping infection by pathogens.
Acknowledgment
This research was carried out within the framework of the project 'Epidemiology, genetics of phytopathogenic microorganisms and development of molecular diagnostic methods' granted by the University of Bari. M S and V P supported by the Czech Science Foundation (GAČR contract no. GA15-04023S).
Authors wish to thank Rete Regionale di Laboratori SELGE (Regione Puglia, Accordo di Programma Quadro 'Ricerca Scientifica'—II Atto integrativo. Del. CIPE n. 35/05.) for the application of the scanning electron microscope platform, and De Corato Sementi (Andria, Italy) for the availability of seed materials.