Abstract
We study the rescattering dynamics of a one-dimensional hydrogen molecule with the nuclei fixed at various internuclear distances. By analysing the laser-intensity dependence of the yields of the ions, we show that the rescattering process strongly depends on the internuclear distance. The dynamics of the ionic excitation is found to reflect the interplay of the rescattering dynamics and the charge-resonant states of the
ion. In addition, we propose an internuclear collisional excitation mechanism for interpreting the enhanced excitation of the
ion at large nuclear distances.
Export citation and abstract BibTeX RIS
1. Introduction
When the strength of a laser field becomes strong enough to distort the binding Coulomb field inside an atom, the interaction of the ultrafast field with atoms and molecules produces many highly nonlinear phenomena, such as high-harmonic generation (HHG), above-threshold ionization and nonsequential double ionization [1–3]. Through the exposure of a helium atom to an ultrafast laser field, the dynamics of the two correlated electrons began to be revealed [4]. However, the hydrogen molecule, with its two flexible cores, make the ultrafast dynamics of the correlated electrons more interesting and complex than for the helium system.
In the presence of an intense laser field, a hydrogen molecule exhibits an abundance of nonlinear phenomena. Considering the variation of the internuclear distance R, the ionization of the hydrogen molecules can be enhanced at some critical distance R, known as charge-resonance-enhanced ionization (CREI) [5–8], and the system is able to absorb more than one photon contributing to the resonance-enhanced multiphoton ionization [7, 9]. The different multiphoton absorption pathways to and
final states can lead to interference, the relative phase of which is controlled by the laser carrier-envelope phase (CEP) [10, 11]. The spatial asymmetry resulting from CEP-dependent interference has been observed experimentally [12–15].
When the laser field intensity increases further, being close to , the Keldysh parameter for the hydrogen molecules will approach to or be smaller than 1 [16], and the dynamics of the interaction between the laser field and the molecules will transform from the multiphoton to the tunnelling regime. In the tunnelling regime, the hydrogen system levels are distorted strongly by the laser field, and the laser-induced dynamics can be well described by the rescattering picture [17]. In this case, one tunnelled electron is accelerated in the laser field and then driven back to the nuclear core, resulting in nonlinear phenomena [18]. For the hydrogen molecule, the rescattering of the returning electron can induce photodissociation and Coulomb explosion of the molecule [19–22]. The rescattering process can also generate HHG, which provides an efficient tool for investigating attosecond dynamics [23, 24]. The rescattering dynamics might also be related to population inversion for the molecular ions, as recently observed through air lasing [25, 26] and superradiant emission [27, 28]. On the other hand, the remaining electron will localize in either core after the ionization of the first electron at large internuclear distances [29, 30] and the localization can be controlled by the laser field [31, 32]. Depending on the localization phase of the remaining electron [24], different channels of rescattering, e.g. rescattering by either atoms, can be significantly different in terms of both amplitude and phase. The motion along the molecular axis and the molecular rotation are important for obtaining accurate angular distributions [33]. However, the geometric anisotropy of the hydrogen molecule makes the rescattering dynamics complex [34–48] and still needs investigation.
In this paper, we focus on the ionic excitation dynamics affected by the internuclear distance of the hydrogen molecule along the molecular axis. Experimentally, the diatomic molecules can be aligned to the laser polarization direction by a weak infrared pulse [49]. Because of the one-dimensional model used in our calculations, the molecular axis has to been restricted to the laser polarization axis. The one-dimensional (1D) time-dependent Schrödinger equation (TDSE) is applied to simulate the two-electron dynamics by the exact solution (2e-TDSE), which can give a qualitative description of the excitation dynamics of the [50]. Based on the rescattering picture in the tunnelling regime, our calculation results show that, besides the recollision process, there is also an internuclear collision process in the laser-induced rescattering. Both impact processes will lead to the electron-correlated excitation of the
ions. It also indicates that the rescattering processes vary distinctly with various internuclear distances. The excitation processes of
are found to depend on the interplay of the rescattering dynamics and the charge-resonant states of the ions and the molecules. Only one single recollision event has been realized with the pulse duration decreasing into the near-single cycle [51–55]. Therefore, for a clear insight into the underlying process of the rescattering, we focus on the two-electron dynamics of
subjected to single-cycle laser pulses.
This paper is organized as follows: the methods of the 2e-TDSE and the details for calculating the population of the excited ions are briefly described in section 2. The results are discussed in section 3. Finally, a conclusion is given in section 4.
2. Methods
A 1D hydrogen model molecule is considered within the dipole approximation [56], where the Coulomb potential of two nuclei are fixed on the x-axis [57, 58], the Hamiltonian can be written as (atomic units are used unless otherwise stated):

with
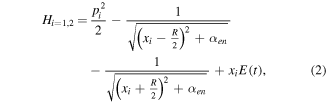
and

where Hi and Hee denote the single-particle and double-particle interaction operators, and R is the distance between the two nuclei. When one electron is ionized, the Hamiltonian of the ion can be given by H1. In this work, the time-dependent two-electron wavefunction
is restricted on the singlet states, which satisfies the symmetry property
. The softening parameters
and
are chosen to reproduce the molecular potential of the hydrogen [50]. With the softening parameters, the energy level of the hydrogen molecule system can be obtained by the diagonalization of the Hamitonians of the
and
.
The potential curves of the ground state and the lowest two states of
are presented in figure 1, which correspond to the
of
and the
and
of
, respectively. The equilibrium distances of the ground states of
and
are 1.6 a.u. and 2.2 a.u., being close to those of the real hydrogen molecule, 1.4 a.u. and 2.0 a.u.. At the equilibrium distance, the 1D model shows the energy difference between the first three energy levels of the
approach to the 3D model [59]. For details of the comparisons of the 1D model with the 3D model, one can refer to [50, 60]. For
at the equilibrium distance, the energy difference between the first two states
and
is 11.18 eV, which is close to the 7.79 eV for the 3D
model [61]. However, the ionization potential of
at the equilibrium distance is 37.14 eV higher than 29.9 eV for the real system, such that the ionization of the second electron needs larger energy. However, the 1D model is adequate for qualitatively studying the rescattering dynamics of the diatomic system.
Figure 1. Molecular potentials for the ground state () of
and the first two states (
,
) of
.
Download figure:
Standard image High-resolution imageFor a clear insight into the underlying process of the rescattering, a single-cycle laser pulse of 400 nm wavelength is used with the electric field:

where τ equals an optical cycle, and when
.
The population probabilities of the ionic states are calculated with the help of absorption boundary, which has the form of:

where in the simulation and the xmax is half of the simulation box size. For each time evolution step, the single-ionization part of the 2D space can be considered as the region [62]:
, or
. In this single-ionization part, we neglect the interaction of the ionized electron with the remaining electron which is bound near the nuclei. Then the populations of the ionic states can be obtained by projecting the single-ionization part on the ionic states:

where factor 2 is given by the indistinguishablity of electrons x1 and x2, and is the ionic state obtained from the diagonalization of the field-free Hamiltonian of the
ion and q labels the quantum number. In the simulation, we assume that the second electron remains in the bound states of the double-well potential for the eigen states with
, while those states with
are treated as double ionization.
To count accurately the populations of the ionic states, a box large enough for propagation of the wavepacket is needed to ensure that the ionized electron is absorbed after the interaction of the laser field. The total single-ionization probability is given by

After the laser field is ended, the final probabilities of the single ionization and the populations of the ionic states
are obtained by summing over each evolution step.
The TDSE is solved with the split-operator method with time-step length [63]. Considering the computational cost, we simulate the problem with
, and the number of spatial grids is 4096.
3. Results and discussion
As shown in figure 1, the first two states of tend to be degeneracy with the increasing distance R [50, 64, 65]. The two states
and
of
can be strongly coupled to the laser field, composing a pair of charge-resonant (CR) states [66]. The CR states play a important role in the ionization of
, resulting in CR-enhanced ionization (CREI) at critical large internuclear distances R [5, 67]. Furthermore, due to the coupling of the CR states at large R, the remaining electron will be localized at either core when single ionization occurs [30]. If the internuclear distance R further increases, the single ionization of
will occur like the emission of an electron from two separate hydrogen atoms. In figure 2, we examine the single-ionization probabilities as a function of the laser field intensity at different internuclear separations. For comparison, we present the single-ionization yields of the model helium atom with the parameters
and
.
Figure 2. Probabilities of the single ionization as a function of the laser field intensity, where the results labelled with H are estimated by uncorrelated electro emission from two independent hydrogen atoms.
Download figure:
Standard image High-resolution imageIt is seen in figure 2 that the probabilities of the single ionization with larger internuclear distances saturate (reach maximum yields) at lower intensities. The phenomenon illustrates that the single ionization is mainly determined by the ionization potential (Ip) of the first ionized electron. The double-well potential with a larger R will generate a lower Ip, which makes ionization of the molecule easier. According to the molecular potentials shown in figure 1, the Ip of
with
a.u. is almost changeless. Therefore the single-ionization curves of
with R = 6.4 a.u. and R = 10 a.u. exhibit similar variations with respect to the laser field intensity. The maximum probabilities of the single ionization
for R = 6.4 a.u. and R = 10 a.u. approach 0.5, which is consistent with the approximation by summing the electron yields from two independent hydrogen atoms. The single-ionization probabilities approximated by the uncorrelated hydrogen atoms provides the limit of molecular single ionization at large R for various laser intensities.
In order to identify the feature of localization of the remaining electron, the populations of the first two states and
of
are presented in figure 3. For the helium atom and
with R = 2 a.u., the probabilities of finding the remaining electron in the excited state is very small. But at large internuclear distance R, like the cases with R = 6.4 a.u. and R = 10 a.u., the two states
and
are nearly equally populated at various field intensities. The equal populations of the two states indicate that the remaining electrons are dynamically localized in either core [30]. Because the electrons localized in the potential well facing the returning electrons will cause stronger scattering events, we examine the details of the recollision dynamics by investigating the yields of ionic excitation. The yields of the ionic excitations are presented as ratios in figure 4, which are divided by single-ionization probabilities
.
Figure 3. Populations of the first two states (blue circles) and
(green squares) as a function of the laser field intensity.
Download figure:
Standard image High-resolution imageFigure 4. Ratios of the respective yields of excitation to the single-ionization probability as a function of the laser intensity, for (a) helium and
at the internuclear distances R = 2 a.u.; (b)
at R = 6.4 a.u. and R = 10 a.u. (a) and (b) also show the excitation ratio from
, labelled by 1e, directly interacting with the laser fields. The knee structures are indicated by arrows, where blue arrows indicate the knee structure for the contribution from internuclear collision and green arrows indicate the recollision.
Download figure:
Standard image High-resolution imageIn figure 4(a), the results for the helium and at R = 2 a.u. are compared. For the helium, a distinct 'knee' structure is shown in the EX curve around
. The knee structure indicates the existence of the two-electron-correlated excitation. According to the rescattering picture, the remaining electron of the ions can be excited by the recollision depending on the impact energy of the returning electron. This impact energy can be estimated by the maximum return energy
[68, 69], where
is the ponderomotive energy and
is the peak strength of the laser field. The factor α characterizes the shape of the laser field envelope and is given 1.6 by the present single-cycle field. In figure 4(a), it is seen that the excitations of the helium ions are almost zero at
, since the return energy is not high enough to excite the remaining electron. If the maximum return energy approaches to the first excitation energy gap
, the remaining electron of the ion can obtain enough energy from the returning electron by the correlated interaction to conquer the energy gap
. The corresponding critical laser intensity is defined as I1 which is listed in table 1. The recollisional excitations begin to significantly participate in the rescattering processes at the critical laser intensity. For the helium, the excitations of the helium ions begin to increase with the rising laser intensity around the critical intensity
, where the knee structure is exhibited. It verifies the dominance of the recollisional excitation in the ionic excitation dynamics at this low laser intensity.
Table 1.
Critical laser field intensities for the excitations of the ions, where the laser intensities I1, I2 and I3 are in units of .
![]() |
Ia2 | Ib1 |
![]() |
Ic3 | |
---|---|---|---|---|---|
![]() |
29.07 | 1.22 | 54.43 | 2.28 | |
![]() ![]() |
12.53 | 1.85 | 0.52 | 38.37 | 1.61 |
![]() ![]() |
11.18 | 0.14 | 0.46 | 25.69 | 1.08 |
![]() ![]() |
12.23 | 0.07 | 0.51 | 23.88 | 1.00 |
For the helium atom, the cross section of the field-free impact excitation will increase with the impact energy and reach the maximum when the impact energy rises to the ionization potential of the singly charged ion [70, 71]. The impact electrons are from the returning processes induced by the laser field. Because of the return energy increasing with the laser intensity, the slope of the ratio for the impact excitation with respect to the laser intensity begins to rise when the laser intensity exceeds the critical laser intensity I1. The slope will increase until the maximum with the increasing of the laser field, and then decrease for the return energy exceeding the ionization potential
. The effect of the impact excitation causes the variation of the slope of the excitation ratio, such that the ratio curve is observed as a knee structure, as shown in figure 4. For the helium, the critical laser intensity
corresponds to the distinct knee structure around
. But for the hydrogen model with R = 2 a.u., the curve shows two knee structures, which are indicated by arrows.
For molecules, because there may be two kinds of impact processes in the rescattering induced by the laser field, we attribute the two knee structures to the two kinds of the impact processes. One impact process is the recollision. The effects of the recollision contribute to the first knee structure indicated by the green arrow, which is dependent on the return energy. For the case with R = 2 a.u., the (given in table 1) corresponds to the first knee structure in the low laser-intensity region, being similar to the recollisional excitation of the helium atoms. The other impact process is the internuclear collision process. When the released first electron is driven in the laser field, it can gain additional impact energy while crossing the inner potential barrier to the other atomic core. The first ionized electron may impact the second electron at the neighbouring core, and the maximum impact energy induced by the laser field can be roughly as
. When the laser intensity is high enough, the impact energy of the internuclear collision will excite the ions. For the small distance R = 2 a.u., the laser intensity needs larger than
(given in table 1), which induces the impact energy of the internuclear collision higher than the first excitation energy gap
of
. Thus the internuclear collisional excitation will contribute to the excitation of the
ion with
. This high intensity corresponds to the second knee structure in the high intensity region. But, in the high intensity region, the laser-induced direct excitation of
can also be significant. In order to examine the mechanism of the laser-induced direct excitation of the ions, we calculate the direct excitation yields of the
ion by solving the 1e-TDSE. For comparison with the results of the hydrogen molecule model, the soft-core parameter value used for the
ion is the same as the hydrogen molecule. The calculation results are indicated by the dashed lines in figure 4. It is seen that the excitation of
induced by the laser field grows linearly with increasing intensity when the laser intensity is higher than
. The contribution of the laser-induced direct excitation makes the second knee structure elevated in the high intensity region.
According to the dependence of the internuclear impact energy Ed on the laser intensity and the internuclear distance R, the internuclear collision will dominate in the lower laser-intensity region when R is large. For the sake of examining the effect of the internuclear collisional excitation, we present the EX ratios for R = 6.4 a.u. and R = 10 a.u. in figure 4(b), where the yields of the excited ions are counted by the excited populations of
with
to exclude the strong coupled
. In figure 4(b), however, there are some abnormal phenomena that the EX ratios with R = 10 a.u. are higher than R = 6.4 a.u. at low intensity, while the results are reversed at the high intensity around
.
In the high intensity region, both the laser-induced direct excitation channel and the recollisional excitation channel can contribute to the ionic excitation. For high intensities around , it is seen as a declining slope in the EX ratio curve with R = 6.4 a.u., which shows a distinct knee structure indicated by the green arrow in figure 4(b). This is because the maximum recollision energy approaches
at the intensity
, which is given as I3 in table 1. With the laser intensity further increasing, the contribution to the ionic excitation from the recollisional process begins to decrease, resulting in that the slope of the ratio curve declines.
The contributions from the different channels can change with the internuclear distance. For the curve with R = 10 a.u., the ionic excitation is lower than the case with R = 6.4 a.u. in the intensity around . The larger excitation of
at R = 6.4 a.u. can be attributed to the direct excitation through a CR-enhanced mechanism similar to CREI [67], as suggested previously [29]. The CREI usually occurs at internuclear distances of
. In order to examine the mechanism of the enhanced excitation, we calculate the direct excitation yields of
at R = 6.4 a.u. and R = 10 a.u. respectively by solving the 1e-TDSE. The results indicate that the direct excitation yields with R = 6.4 a.u. are generally higher than R = 10 a.u. at various laser intensities, as shown in figure 4(b), which is consistent with the CR-enhanced mechanism.
On the other hand, at the lower laser intensities, the excitation ratios at R = 10 a.u. are higher than that of molecules at R = 6.4 a.u. in contrast to the single-electron approximation based on the CR-enhanced mechanism. The internuclear collision mechanism is proposed to account for this abnormal excitation. The impact energy can be much larger than the recollision energy at large R. This can be estimated from the previously defined critical intensity I2 which is
, for R = 10 a.u. while
for R = 6.4 a.u. Therefore it is more efficient to excite molecules at larger nuclear distance (R = 10 a.u.) at the low laser intensity through internuclear collision. Again, as the laser intensity increases when this impact energy exceeds the second ionization potential
, the impact excitation yields saturate as seen in the knee structure around the intensity
for R = 10 a.u., as indicated by the blue arrow in figure 4(b). Note that for the contribution from the internuclear collision channel to the ionic excitation, the knee structure can appear at much lower laser intensities with a larger internuclear distance.
4. Conclusion
In conclusion, we have investigated the interplay of the rescattering and the charge-resonant states dynamics for a 1D hydrogen molecule model. The results suggest that the core excitation and the ionization of the hydrogen molecule strongly depend on the internuclear distance, and hence depend on the electron localization and the two-electron correlation. The 1D model gives a qualitatively study of the laser-intensity dependence of the ionic excitation.
For the diatomic molecule, it suggests that there is a contribution from the internuclear collision channel to the ionic excitation. This contribution may rise significantly with the internuclear distance and the laser intensity increasing. For the hydrogen molecules initialized at the ground vibrational state, experimental observation may be difficult, since the laser intensity needed is too high. If the hydrogen molecules are produced in the highly excited vibrational state, the effect of internuclear collision in the rescattering dynamics cannot be neglected. However, one may search for another molecular system, such as the rare-gas dimers [72–75], which have large equilibrium internuclear separations at the ground vibrational state. The argon dimer, with the equilibrium internuclear distance 7.13 a.u., has been used to study the CR-enhanced ionization [74, 75] of the diatomic molecules, and provides a chance to investigate the internuclear collision effect.
Acknowledgments
This work is supported by the National Basic Research Program of China (973 Program) under grant no 2013CB922203, the NSF of China (Grants No. 11374366, 11404401 and 11474359).