Abstract
In this work we investigate interfacial effects in bilayer systems integrated by La2/3Sr1/3MnO3 (LSMO) thin films and different capping layers by means of surface-sensitive synchrotron radiation techniques and transport measurements. Our data reveal a complex scenario with a capping-dependent variation of the Mn oxidation state by the interface. However, irrespective of the capping material, an antiferromagnetic/insulating phase is also detected at the interface, which is likely to originate from a preferential occupancy of Mn 3d 3z2−r2 eg orbitals. This phase, which extends approximately to two unit cells, is also observed in uncapped LSMO reference samples, thus pointing to an intrinsic interfacial phase separation phenomenon, probably promoted by the structural disruption and inversion symmetry breaking at the LSMO free surface/interface. These experimental observations strongly suggest that the structural disruption, with its intrinsic inversion symmetry breaking at the LSMO interfaces, plays a major role in the observed depressed magnetotransport properties in manganite-based magnetic tunneling junctions and explains the origin of the so-called dead layer.
Export citation and abstract BibTeX RIS
For more information on this article, see LabTalk.
1. Introduction
Complex oxides have emerged as one of the most interesting classes of materials due to their remarkable variety of properties which are of strong theoretical and technological interest, including superconductivity, ferromagnetism, ferroelectricity, etc. Transition metal (TM) oxides are especially relevant since they present large electronic correlations leading to strong competition between various degrees of freedom [1]. The physical properties of TM oxides are determined by d electrons and the way they are distributed between the five-fold degenerate 3d orbitals. Hence, control of 3d-orbital occupancy is expected to allow the engineering of new functionalities [2–4]. The strong electronic correlations in these materials induce a local entanglement of the charge, spin and orbital degrees of freedom, which enables different options, such as structural distortions and crystal chemistry, to control 3d-orbital occupancy. These features offer the possibility of an active tuning of physical (electronic and magnetic) and chemical (catalytic reactivity, wettability, etc) properties. From this perspective TM oxide heterostructures offer a unique arena for engineering new functionalities since charge, spin and orbital degrees of freedom meet at interfaces and are strongly affected by electronic processes such as charge transfer, hybridization and exchange interactions. In recent years, there has been intense activity in this field and several new phenomena and novel states of matter have been reported [2, 5–7].
Among complex oxides, manganites, with their very robust perovskite structure, offer a plethora of possibilities, from both a fundamental and an applied physics point of view. Manganites are complex systems exhibiting a broad range of physical phenomena, including large spin polarization and colossal magnetoresistance [8]. These properties make them very appealing for the development of novel concepts for the implementation of oxide-based spintronic devices. Especially relevant is the case of La0.67Sr0.33MnO3 (LSMO) which exhibits the highest magnetic transition temperature (TC ~ 370 K) of this family of compounds [9], and therefore could be implemented in magnetic tunneling devices that work at room temperature. However, state-of-the-art LSMO-based junctions show vanishingly small tunneling magnetoresistance (TMR) values above 280 K [10]. This is usually attributed to the existence of a so-called dead layer, a few unit cells wide, at the interface between the manganite and the tunnel barrier, whose origin is not yet well understood. TMR is a spin-dependent process and, as such, depends critically on the conducting and magnetic properties of the few atomic layers next to the insulating barrier. When two dissimilar oxides are placed together, such as the case of manganite/tunnel barriers in all oxide magnetic tunneling junctions (MTJs), electronic and structural reconstruction at the interface, controlled primarily by elastic strain and electrostatic boundary conditions, may substantially modify 3d-orbital filling, breaking the eg-orbital degeneracy, thus drastically modifying the magnetic and transport properties.
The study of interfacial effects is difficult because in LSMO/tunnel barrier structures interfaces are buried several nm below the top electrode. Consequently, most approaches have consisted of investigating the film/substrate bottom interface in ultra-thin films. In these cases it is expected that the structural strain imposed by the substrate will play a dominant role. The substrate constrains the in-plane film cell parameters and imposes its in-plane symmetry operations on the film. The latter, being much weaker than the former, will affect the first few cells close to the film/substrate interface and relax [11,12]. In fact, it has been shown both theoretically [13] and experimentally [14–16] that tensile stress favors the x2−y2 orbital occupancy and thus CE-type antiferromagnetic (AF) ordering while compressive strain favors 3z2−r2 occupancy leading to a C-type AF ordering. However, x-ray linear dichroism (XLD) investigations in manganite ultra-thin films show that, irrespective of the biaxial strain conditions, the interfacial Mn atoms show a preferential occupancy of the Mn 3d 3z2−r2 eg orbitals [15–17]. The reason for this strain-independent selective orbital occupancy is not yet clearly established.
In this work we address the origin of the so-called dead layer appearing by the interface in the manganite/tunnel barrier MTJ. We characterize the magnetic and transport properties at the interface between LSMO and different capping layers (CLs) of interest for spintronic applications (SrTiO3, LaAlO3 (LAO), NdGaO3 (NGO), MgO and Au), representative of the manganite/tunnel barrier interface in MTJ. In order to isolate the electronic structure at the interface, we performed a systematic series of experiments on heterostructures with different CLs, taking advantage of the element specificity and shallow probing depth of x-ray absorption spectroscopy (XAS), x-ray magnetic circular dichroism (XMCD) and XLD in the total electron yield (TEY) mode.
Our data reveals the presence of about 1 nm thick AF/insulating layer at the LSMO/CL interface, which is independent of the capping material and very similar to that found at the free surface of the LSMO thin films. Our results indicate that the structural disruption, with its intrinsic inversion symmetry breaking at the interfaces, lay at the origin of the so-called dead layer.
2. Results and discussion
LSMO samples used in this work were prepared by radio frequency (RF) magnetron sputtering on top of the (0 0 1)-oriented SrTiO3 (STO) substrates. Prior to deposition STO substrates were cleaned in an ultrasonic bath with Milli-Q water and annealed at 1000 °C in air for 2 h to obtain a typical morphology of terraces and step with unit cell height (≈ 0.4 nm), thus selecting a mostly unique atomic termination, likely to be TiO2. The thickness of the samples (~40 nm) was determined by using grazing incident x-ray reflectometry. The thickness of the CLs (tc~1.6 ± 0.2 nm) was determined by controlling the evaporation time after a careful calibration of the growth rate for each of the different materials used. It was also checked 'a posteriori' by using high-resolution transmission electron microscopy (HRTEM), obtaining good agreement with the nominal values (see figure 1). Further details regarding sample preparation can be found in [18]. Reciprocal space mapping was performed using a Bruker D8 GADDS system equipped with a 2D Hi-Star x-ray detector to determine the degree of strain on the films. LSMO films are in-plane fully strained [19], which according to previous results will favor the preferential occupancy of x2−y2 orbitals [13–16]. Samples exhibit ferromagnetic (FM) transition temperatures slightly below that of the bulk material with values of the saturation magnetization close to that of the bulk. A complete magnetic characterization of the samples can be found in [19].
Figure 1. HRTEM picture of the LSMO/LAO interface
Download figure:
Standard image High-resolution imageThe synchrotron radiation experiments were performed at the electron storage ring of the Helmholtz-Zentrum Berlin (BESSY) by using the 70 kOe high-field end station located at the UE46-PGM1 beamline. The experiments were done at low temperature (T=10 K), thus well within the FM phase of the LSMO thin films (Tc≈350 K). XAS and XMCD spectra at the Mn L3,2-edge were obtained at various fields ranging from 0 to 6 T applied perpendicular to the sample surface with the incoming circularly polarized radiation impinging the sample at normal incidence with respect to its surface plane. XMCD spectra were obtained by reversing the polarization of the incoming circularly polarized light. The XLD experiments were done using incoming horizontal linearly polarized radiation at the Mn L3,2-edges and measuring spectra at two different angles of incidence, i.e. 90° (normal incidence) and 30° in order to gain sensitivity to in-plane x2−y2 and out-of-plane 3z2−r2 oriented eg Mn 3d orbitals, respectively. TEY detection mode was used in all cases. The escaping depth of the secondary photoelectrons (2–5 nm) guarantees that the measured spectra are mainly determined by the Mn atoms close to the interfacial region.
XAS results, obtained by averaging spectra measured with left and right incoming circularly polarized light are shown in figure 2(a), together with the reference spectrum corresponding to an uncapped LSMO film. Due to their similarities, the latter will be taken as representative of bulk LSMO [20]. It is evident from the figure that NGO and MgO capping promotes the appearance of increased intensity at the high-energy side of the L3 peak (643–645 eV) with respect to the reference spectrum. Spectral shifts towards higher energy values are also observed, thus indicating an increase of the oxidation state of the Mn ions, i.e. an increase in the Mn4+ content at the interface. A comparison with the reference sample allows us to estimate that the increase in the Mn4+ content at the interface amounts to ~15–18% in both cases. In contrast, STO and Au capping promotes an increase of the L3 peak intensity at the low-energy side (641.2 eV), together with a spectral shift (~0.1 eV) towards lower energy values. The spectral energy shift and the size of the spectral feature at 641.2 eV observed for the case of LSMO films allows for the exclusion of Mn4+ and Mn3+ contributions [21] and points to the presence of Mn2+ by the interface [20]. According to the procedures described in [20] the amount of Mn2+ is estimated to be ~6% for STO capping and ~14% for Au capping. Of particular interest is the LAO capping which exhibits almost bulk-like spectrum with only minute differences at the low-energy side of the L3 peak, indicating a tiny increase of Mn3+/reduction of Mn4+ (~1–2% [21]).
Figure 2. (a) Mn L3-edge absorption spectra for LSMO/CL bilayers obtained at H = 0 T and T = 10 K. Spectra are vertically shifted in order to allow better comparison. The reference spectrum corresponding to an LSMO uncapped film is shown in gray. (b) XMCD spectra corresponding to LSMO films with different CLs. Spectra are vertically shifted in order to allow better comparison.
Download figure:
Standard image High-resolution imageThe magnetic properties of the interface have been studied by using XMCD and XLD. XMCD is experimentally obtained as the difference between two absorption spectra measured with opposite helicities (μ+ and μ−) at normal incidence and with the films magnetically saturated out-of-plane by using a magnetic field of 3 T. A non-zero difference is the signature of FM phases. The results for different interfaces are compiled in figure 2(b). An exact determination of the interface Mn magnetization by using the so-called sum rules [22, 23] is not possible due to the large error associated with the determination of the spin contribution to the magnetic moment of Mn [24]. However, a relative comparison between the magnetic moment (mspin + morb) per Mn atom for the different interfaces can be performed by normalizing their values to that obtained for the reference uncapped sample. It is found that, compared to the uncapped film, all CLs, except LAO, promote depressed magnetic moments at the LSMO/CL interface, in agreement with the departure from the Mn3+/Mn4+ valence balance corresponding to the 2/3–1/3 nominal composition previously shown. The behavior observed in the case of LAO capping has been explained in terms of the different effect of electron–hole doped schemes at the LSMO interfacial layers [6, 19]. As shown in [6], hole doping by the interface, as in the case of the LSMO/LAO interface, seems to be less detrimental for the FM ordering at the uppermost LSMO layers than electron doping. The close similarity of all the XMCD spectra with that of the uncapped LSMO allows for the exclusion of any other FM phase, a part from the one expected to correspond to the 2/3–1/3 nominal composition. It is therefore clear that the reduction of magnetic properties at the LSMO/CL interface must arise from the local presence of non-magnetic (NM) and/or AF phases. To explore this possibility we employed XLD.
A magnetic field of 20 kOe was applied along the beam propagation direction, saturating the sample magnetization in order to eliminate the FM contribution to the XLD spectra, thus leaving only AF and anisotropic orbital occupancy contributions. Two spectra were obtained at θ=90° (β90) and at θ=30° (β30) in order to gain sensitivity to in-plane x2−y2 and out-of-plane 3z2−r2 oriented eg Mn 3d orbitals, respectively. The XLD spectrum, (defined as XLD = β90–β0, being β0= 4/3(β30–1/4β90[25])) corresponding to the uncapped LSMO sample is depicted in figure 3(a). Comparison with previously reported temperature-dependent XLD spectra [17] allows us to identify the presence of an AF phase whose magnetic axis is aligned perpendicularly to the sample´s plane. Note that the experimental conditions guarantee that the XLD signal arises only from AF and anisotropic orbital occupancy contributions. The XLD curve for the LSMO surface is identical to that reported by Aruta et al [17] (see figure 4(b) in [17]) as indicative of a C-type AF phase which originates from a preferential Mn 3d 3z2−r2 eg orbital occupancy, indicating that at the topmost LSMO layers close to the free surface, the eg orbital degeneracy is broken. Similarly, recent results reported in [16] indicate that irrespective of the strained state of the samples, a preferential 3d 3z2−r2 eg orbital occupancy occurs at free surfaces. Our data go a step further and reveal that, irrespective of the CL material, a breaking of the eg orbital degeneracy takes place at the LSMO/CL interface. The XLD spectra for the different CLs are shown in figure 4. The closeness in their spectral shape indicates that the AF phase observed in the uncapped film is also present at the interface of LSMO with different CLs, irrespective of the capping material. Moreover, a quantitative analysis of the XLD spectra demonstrates that this similarity extends not only to the spectral shape, but also to its amplitude. The amplitude, defined as 100I|XLD|/IXAS, where I|XLD| stands for the integrated intensity of the |XLD| spectrum and IXAS corresponds to that of the absorption spectrum β, is similar for all samples. Comparison with the results obtained by XAS and XMCD show that the XLD amplitude is neither correlated to the NM phase content (table 1) nor to the interface magnetization reduction deduced from XMCD data. However, the XLD amplitude does exhibit a clear correlation with the DE-FM phase with the nominal Mn3+/Mn4+ valence balance (figure 5), thus indicating the intrinsic character of this interfacial layer.
Table 1. Values of the barrier thickness, t, and the barrier height, ϕ0, for the different CLs obtained from equation (1) in the low bias voltage regime. Δt corresponds to the difference between t and the thickness of the CL (~1.6 nm). NM corresponds to the fraction of phase with Mn oxidation state differing from the nominal Mn3+/4+ mixed valence state. The relatively low value of Δt in the case of MgO deserves further investigation and might be related to the active role of the MgO barrier and its spin filtering effect. It is also worth mentioning here that for MgO capping J(V) curves are slightly asymmetric and the fittings from equation (1) are worse than in the other cases. This might also affect the evaluation of Δt for MgO capping.
Capping layer | ϕ0 (eV) | t (nm) | Δt ~ t−tc (nm) | NM (%) |
---|---|---|---|---|
LAO | 0.45 | 2.6 | 1 | ~0% |
STO | 0.32 | 3 | 1.4 | 6% |
NGO | 0.41 | 3.6 | 2 | 15–18% |
MgO | 0.38 | 2.5 | 1 | 15–18% |
Figure 3. (a), (b) Mn L3,2-edge XAS spectra corresponding to the uncapped and NGO-capped LSMO samples, respectively. The electric field vector of the incoming linearly polarized radiation was set parallel (β90) and almost perpendicular (β30) to the surface of the sample. Both sets of data allowed calculation of the β0 spectrum (see text). A magnetic field of 20 kOe was applied parallel to the beam propagation direction saturating the sample magnetization (inset 1(a)) to remove the FM contribution to the XLD (β90−β0) signal (blue line). Inset 1(b): the βNGO−βuncapped difference agrees with the expected shape for Mn4+ after proper scaling (open dots) (see [21]). βuncapped is characterized by a bulk-like mixed valence Mn3+/Mn4+ spectral shape.
Download figure:
Standard image High-resolution imageFigure 4. XLD spectra obtained at Mn L3,2-edge for the various LSMO/CL bilayers included in this study. The XLD has been normalized to its maximum value and an offset has been artificially applied for better comparison.
Download figure:
Standard image High-resolution imageFigure 5. Comparison between the integrated intensity of |XLD|, i.e. I|XLD| versus the integrated intensity of the raw XAS (no normalization) originating from a nominal 2/3–1/3 mixed valence Mn3+/4+ state, i.e. IMn3+/Mn4+ (a) and from the Mn2+ and Mn4+ impurities (b). The intensity corresponding to the NM has been calculated by using the NM (%) values of table 1. A clear linear correlation is observed only between I|XLD| and IMn3+/Mn4+.
Download figure:
Standard image High-resolution imageThese results therefore reveal a complex scenario at the manganite's surface and interfaces in which FM, NM and AF phases coexist. Both NM and AF phases depress interfacial magnetotransport properties. The NM phase is characterized by the existence of Mn2+ or by an excess of Mn4+ atoms and is sensitive to the absence/presence and nature of the CL. On the other hand, the AF phase seems to be bound to the amount of the 2/3−1/3 DE-FM LSMO phase at the surface/interface. This fact, in addition to the surface sensitivity of the TEY detection technique, suggests that the AF phase extends homogeneously at the interface, i.e. it is indeed an interfacial layer.
To further characterize this AF phase we have investigated the transport properties across the interface for the samples capped with LAO, STO, NGO and MgO by means of an atomic force microscope (AFM) system, working in the current sensing (CS) mode (see [26] for details). As expected, I(V) characteristic curves across the LSMO/CL interfaces exhibit the typical features of a tunneling conduction process (see figure 6). The effective thickness of the insulating barrier layer has been estimated from a quantitative analysis of the I–V characteristic curves by using the Simmons model in the intermediate voltage range given by the equation [27]
Figure 6. Dependence of the current density as a function of the voltage bias, J(V), across the LSMO/CL interface for different CLs. The continuous line corresponds to the fitting from equation (1) in the text. Insets: details of the J(V) curve for the LSMO/MgO (upper) and LSMO/NGO (lower) systems.
Download figure:
Standard image High-resolution image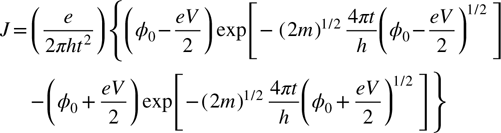
ϕ0 being the barrier height and t the effective insulating barrier thickness. Some examples of these fits are shown in figure 6. The obtained values for ϕ0 and t are summarized in table 1. The values obtained for ϕ0 are in good agreement with those previously reported [27–30]. Our analysis yields values for the effective insulating barrier thickness, t, larger by Δt ~ 1–2 nm than those corresponding to the nominal thickness of the CL (see figure 1). This indicates that the effective insulating barrier thickness has been increased by interfacial effects in the LSMO topmost layers. In principle, there are two possible sources for explaining this extra insulating layer at the interface, i.e. the NM and the AF phases. Comparison of the values obtained for Δt with the amount of secondary NM phases detected with XAS (see table 1) for the different interfaces yields an almost perfect linear correlation (r2=0.99) between Δt and the amount of NM phase. However the intercept for NM= 0% turns out to be non-zero (~1 nm). This can be understood by assuming that in all cases there is an extra ~1 nm thick interfacial insulating layer, i.e. independent of the amount of NM phase. We argue that this ~1 nm insulating layer is related to the AF layer, whose existence at the LSMO surface/interfaces has been highlighted by XLD measurements and whose presence turned out to be independent of the capping material. Indeed this idea is corroborated by the results obtained for the LSMO/LAO interface in which no NM phase has been detected, but a Δt =1 nm is still observed. These results therefore allow us to assign an effective thickness of appromixately 2 ML (~1 nm) for the C-type AF-insulating phase present at the LSMO surface and LSMO/CL interfaces.
The origin of this C-AF insulating phase is controversial. The main forces at work by the interface can be grouped in (i) structural strain [31], (ii) electronic reconstruction [32], and (iii) structural disruption with inversion symmetry breaking at the interface [1, 11, 33]. In what follows, we will analyze each of these contributions in order to clarify their relevance in determining the eg orbitals occupancy at the interfacial region, and therefore their magnetic and electronic properties.
- (i) Structural strain is very important since the substrate constrains the films in-plane cell parameters. Indeed our samples are in-plane fully strained (aFilm = aSubst ~0.3905 nm) with a slightly reduced out-of-plane cell parameter c (~0.3870(3) nm). This elongation of the in-plane cell parameters would favor the preferential occupancy of the x2−y2 orbitals due to the combined effect of the anisotropic change of the cell parameters, and therefore of the hopping amplitudes leading to a CE-type AF phase [11, 13, 14]. However our XLD data, corresponding mainly to the LSMO/CL interfacial region, exhibit clear features indicative of a 3z2−r2 preferential orbital occupancy promoting a C-type AF ordering irrespective of the CL material and the structural strain. In fact, recent results reported in [16] clearly demonstrate that, irrespective of the strained state of LSMO thin films, a preferential occupancy of the Mn 3d 3z2−r2 eg orbitals takes place at LSMO free surfaces. Consequently it is clear that strain alone cannot explain the observed preferential occupancy of the 3z2−r2 orbitals neither at substrate/LSMO nor at LSMO/surface of LSMO/CL interfaces.
- (ii) The electronic structure of the LSMO film and the CLs interact at the interface and possible delocalization effects should be taken into consideration. For instance, in the case of LSMO/STO interfaces it is expected that the eg manganese orbitals delocalize to some extent into the Ti 3d empty states. This scenario is particularly favored in the case of the 3d 3z2−r2 orbitals of the Mn and Ti atoms. This delocalization energy tends to favor a Jahn–Teller distortion increasing the occupancy of the 3z2−r2 orbitals of the Mn and therefore the C-AF phase [11]. Although the expected preferential occupancy in that case agrees with our experimental finding, interfacial electronic effects can also be ruled out based on our experimental results. First, the amplitude of the XLD signal is similar in both capped and uncapped LSMO films pointing to a minor (if any) effect of the capping on the appearance of the C-AF phase. Second, the effect is observed for different CL materials such as, STO; with neutral SrO or TiO2 layers, NGO; with charge unbalanced NdO or GaO2 layers or LAO; where the above mentioned hole-doping mechanism does not apply [34]. In addition it is also observed in the case of CL materials with no perovskite structure, such as MgO, or even a metallic capping as for Au.
- (iii) Finally, it has to be considered that the interface introduces a structural disruption that implies inversion symmetry breaking, which may strongly modify the electronic properties of the LSMO interfacial layers. In this sense, the evident effect of the interface is distorting the cubic symmetry around the Mn ions. This distortion is accommodated by a combination of uniform deformations and staggered rotations of the metal-oxide octahedra, which influence the orbital occupancy [6]. The distortion of the cubic symmetry around the Mn ions by the interface implies changes of the local crystal field and the oxygen mediated covalent bonding between metal ions. These changes may trigger specific spin–orbital reconstructions and selective orbital occupancy with the concomitant change of the magnetic and electronic properties. In fact, in the extreme case of manganite free surfaces it has been theoretically predicted that the charge state of the Mn ions is strongly modified leading to charge localization and the preferential occupancy of the 3z2−r2 orbital within the 2 ML closest to the surface [35], i.e. the formation of a thin C-type AF phase at the surface. Our experimental results obtained in the uncapped LSMO film clearly confirm these theoretical predictions. We observe the existence of an AF phase at the LSMO surface by XLD and also in the LSMO/CL interfaces. In addition, transport measurements in the bilayers allow it to demonstrate its insulating character and estimate its thickness (~1 nm), which is also in excellent agreement with the theoretical predictions [36].
We would like to emphasize the fact that the strength of the detected C-AF phase is independent of the CL material and similar in all the cases to that of the LSMO uncapped surface. From these results we conclude that a robust C-AF phase arises at the surface of LSMO films immediately after the growth due to the structural disruption and breaking of the inversion symmetry, and once formed this AF phase is strong enough to persist after capping.
The AF/insulating layer detected here offers a clear explanation of the microscopic origin of the so-called dead layer that has often been studied in the case of manganite/substrate interfaces. The structural strain is very relevant, but scarcely investigated at the interface, which determines the magnetotransport properties in oxide-based MTJ, i.e. the interface with the insulating barrier. Moreover, it might also explain why the improvement of the microstructural quality with atomic sharp interfaces in manganite MTJ, although leading to an increase of the temperature range where TMR can be observed, does not lead to the observation of a sizable room temperature magnetoresistance.
As we have shown, the capping of the LSMO samples leads in most cases to the appearance of NM phases. In addition, due to the structural disruption, we also detect the existence of an AF/insulating phase which should be considered as concomitant to the interface because of inversion symmetry breaking. Advances in thin film deposition techniques might have yielded almost perfect atomic sharp interfaces from a chemical and crystallographic point of view, thus reducing the presence of the NM phase. The structural disruption, however, with its intrinsic inversion symmetry breaking and the appearance of the concomitant robust C-type AF phase, is unavoidable.
These results allow us to envisage the correct strategy for the construction of manganite-based tunneling devices. Our results suggest that in order to avoid the degradation of the performances of manganite layers at the interfaces, the insulating barrier has to be chosen to avoid the structural disruption, i.e. taking advantage of the very rich phase diagram of these materials and modifying the doping rate to obtain an AF-insulating phase for the barrier. This could explain the good performance of La0.67Ca0.33MnO3/La0.3Sr0.7MnO3/La0.67Ca0.33MnO3 tunneling junctions presenting TMR values vanishing only 10 K below the TC of one of the electrodes [36].
In conclusion, the combination of synchrotron radiation spectroscopic techniques with transport measurements at room temperature, both sensitive to the LSMO interface, highlights a complex scenario at the manganite thin film surfaces and interfaces in which FM, NM and AF phases coexist. Transport measurements show that the disruption of the DE-FM phase occurs only at the interface where a thin insulating layer of about 2–4 ML is present. This insulating layer is linked to the NM and AF phases. However, in addition to this, we have detected the existence of a residual 2 ML thick AF-insulating layer whose existence is concomitant to the nominal 2/3–1/3 DE-FM phase. Its origin lies on the preferential occupancy of the 3d z2−r2 eg orbitals at the interface, due to the structural disruption and inversion symmetry breaking of the crystal structure, leading to a C-AF ordering. This AF phase is robust enough to survive after capping and therefore is likely to be present in MTJ interfaces. The presence of this phase modifies the features of the tunneling barrier, severely affecting the tunneling conduction process.
Acknowledgments
We acknowledge financial support from the Spanish MINECO (MAT2012-33207), CONSOLIDER (CSD2007-00041), and FEDER program. The research leading to these results has received funding from the European Community's Seventh Framework Program (FP7/2007–2013) under Grant agreement no 226716. ZK thanks the Spanish MEC for the financial support through the RyC program.