ABSTRACT
We report on the first deep, direct search for a magnetic field via the circular polarization of Zeeman splitting in a Wolf-Rayet (W-R) star. Using the highly efficient ESPaDOnS spectropolarimeter at the Canada–France–Hawaii Telescope, we observed at three different epochs one of the best W-R candidates in the sky expected to harbor a magnetic field, the bright, highly variable WN4 star EZ CMa = WR6 = HD 50896. We looked for the characteristic circular polarization (Stokes V) pattern in strong emission lines that would arise as a consequence of a global, rotating magnetic field with a split monopole configuration. We also obtained nearly simultaneous linear polarization spectra (Stokes Q and U), which are dominated by electron scattering, most likely from a flattened wind with large-scale corotating structures. As the star rotates with a period of 3.766 days, our view of the wind changes, which in turn affects the value of the linear polarization in lines versus continuum at the ∼0.2% level. Depending on the epoch of observation, our Stokes V data were affected by significant crosstalk from Stokes Q and U to V. We removed this spurious signal from the circular polarization data and experimented with various levels of spectral binning to increase the signal-to-noise ratio of our data. In the end, no magnetic field is unambiguously detected in EZ CMa. Assuming that the star is intrinsically magnetic and harbors a split monopole configuration, we find an upper limit of B ∼ 100 G for the intensity of its field in the line-forming regions of the stellar wind.
Export citation and abstract BibTeX RIS
1. INTRODUCTION
In the universe, magnetic fields can be found in a variety of celestial objects. Stars are no exception. In fact, stellar magnetism is ubiquitous in most parts of the H-R diagram. However, its characteristics such as the field strength, topology, and the percentage of magnetic stars at a given spectral type are known to a lesser extent and need to be further investigated. Nonetheless, some stars are now definitely considered as magnetic, even strongly so, while others present a much less obvious portrait of their magnetism. Massive stars (Minit > 8 M☉) fall in the latter category. Despite their relatively small numbers, they dominate the ecology of the universe via their extreme output in photons and particles. This occurs not only during the final supernova explosion but, especially for the more luminous hot stars, via their fast dense winds during their entire lifetimes. Whereas the presence of a magnetic field seems to be a common feature among low-mass stars (Minit < 1.5 M☉), only a minority of high-mass stars show magnetic properties.
Nonetheless, there are very good reasons to expect some massive stars to harbor magnetic fields. As the interstellar medium itself possesses organized, large-scale magnetic fields (Vallée 2004), it is unavoidable, assuming magnetic flux conservation (Heiles & Crutcher 2005) in plasmas, that all types of stars, because they result from cloud collapse, will have a fossil magnetic field, possibly a very strong one, at least when they enter the zero-age main sequence. Moreover, Cantiello et al. (2009) studied the iron convection zone of hot massive stars and suggested that magnetic fields produced in this sub-photospheric convective layer could rise to the surface of OB stars. These models certainly represent a step forward, but refinements are still needed. In particular, magnetohydrodynamic (MHD) models have not yet confirmed the efficiency of the Tayler–Spruit instability in stellar interiors (Mathis & Zahn 2005). It is clear that models with magnetic fields need to be urgently constrained observationally. Magnetic fields could also be the cause of abundance anomalies (Henrichs 2001; Henrichs et al. 2003), be a key factor in the generation of non-thermal and/or X-ray emission (Chlebowski 1989; Drake 1990), and possibly be at the origin of large-scale wind asymmetries that lead to rotationally modulated, periodic spectral and photometric variability (St-Louis et al. 1995). Indeed, the morphology of a rotating stellar wind can be influenced if the mass-loss rate per unit area of the stellar surface is non-uniform, perhaps due to a corotating magnetic field (Harries 2000). Corotating interaction regions (CIRs) can then be formed by radial streams of higher and/or lower density material twisted by differential wind rotation into spiral structures (Cranmer & Owocki 1996). Therefore, spectropolarimetry of population I Wolf-Rayet (W-R) stars at a high level of sensitivity will most certainly provide constraints for future modeling and thus may have major implications on our understanding of their evolution.
Furthermore, neutron stars (NSs), the descendants of many massive stars with initial masses in the range of 8 M☉ ≲ Minit ≲ 20 M☉ (Hansen et al. 2004), display large magnetic fields, typically with B ∼ 1012–13 G, which translates, via magnetic flux conservation, to B ∼ 2–20 G at the surfaces of early OB-type stars. Extrapolating to more massive stars, these relatively low field values possibly explain the fact that at present, detectable magnetic fields seem to be the exception for O stars rather than the rule (Wade et al. 2012). The few O stars with strong magnetic field detections may be related to magnetars with B ∼ 1015 G (Thompson et al. 2004), which are likely the remnants of massive stars above 30–40 M☉ (Gaensler et al. 2005). Magnetars are NSs, which implies that at least some stars above 20 M☉ can become NSs after all, and not necessarily black holes (Heger et al. 2003; Fryer & Kalogera 2001).
Currently, less than 20 OB-type stars have definite detections of surface magnetic fields (Walder et al. 2011), and no direct detection has been made, up to now, for their evolutionary successors, W-R stars; the real challenge in the latter case is, in fact, to unambiguously detect the field. The direct detection of a magnetic field in W-R stars requires instruments that were not available until the arrival of ESPaDOnS (Echelle SpectroPolarimetric Device for the Observations of Stars) at the Canada–France–Hawaii Telescope (CFHT) and FORS1 (FOcal Reducer and low dispersion Spectrograph) at the Very Large Telescope. These instruments are an order of magnitude more sensitive than previous ones (Donati 2003). Since the former offers a higher spectral resolution than the latter (∼50 times better), it allows observers to detect more subtle variations throughout the line profiles while being also able to reach higher signal-to-noise ratio (S/N) by binning data (reaching similar resolution as unbinned FORS data). Now, at last, we have a unique opportunity to make significant progress in this unexplored domain.
In the case of core He-burning W-R stars, the hydrostatic core radii (Rcore) are typically an order of magnitude smaller than those of their O progenitors, so the expected surface magnetic fields in W-R stars could be ∼100 times higher than in O stars, i.e., B ∼ 200–2000 G, assuming magnetic flux conservation. However, because of W-R stars' opaque stellar winds, we can generally not observe down to their hydrostatic surfaces. According to Schulte-Ladbeck et al. (1995) and using Rcore ∼ 2.65 R☉ (Hamann et al. 2006) and β = 3 in the velocity law—as for EZ CMa—one can calculate that the strong W-R winds only allow us to see emission lines down to ∼4 Rcore. If one adopts the likely configuration of a split monopole to describe the field (ud-Doula & Owocki 2002; Gayley & Ignace 2010)—essentially a bipolar field pulled radially outward by the strong outflow—then the magnetic field would vary as R−2 and would be ∼42 smaller at 4 Rcore than at the hydrostatic core. This implies fields of ∼10–100 G expected in the inner observable parts of W-R winds, with much larger values for pre-magnetars. In a sense, it is even more urgent to detect magnetic fields in W-R stars, which are evolutionarily closer to compact remnants than are O stars.
1.1. EZ CMa = WR6 = HD 50896
The W-R star EZ CMa (WR6 = HD 50896) is one of the brightest and best studied stars of its kind. It has now been observed at almost all wavelengths available with ground-based and space observatories in an attempt to understand its puzzling nature and ultimately the extreme stellar wind properties of W-R stars and the consequences on their circumstellar environment. EZ CMa is a WN4 star of magnitude mV = 6.94 (van der Hucht 2001), the sixth brightest W-R star in the Galaxy and in the night sky. EZ CMa exhibits unusual properties that still need to be explained and confirmed observationally. It shows periodic optical and UV line and continuum variability with a period of P = 3.766 days (Lamontagne et al. 1986). The multiple signs of variability also point toward long-term dynamic changes in the large electron-density distribution and geometry. These wind variations are seen in photometric, polarimetric, and spectroscopic observations over the same timescale, suggesting that a common mechanism is responsible for the slow and continuous evolution of the observed changes (Robert et al. 1992). Matthews et al. (1992) and Duijsens et al. (1996) also reported flare-type variability in EZ CMa. The former authors suggested that the intriguing brightness increase is consistent with a field strength of Bcore ∼ 103 G, if due to the reconnection of magnetic field lines at the stellar surface. Robert et al. (1992) argued that the lack of long-term coherency in the shape of the phase variations could be accounted for by a slow random growth and fading of disturbances or spots in the visible parts of the rotating star. Similar modulations are observed in T Tauri and RS CVn stars. Constrained but variable magnetic loops are implied, and a field strength of Bwind > 90 G in the accelerating part of the wind is required. Furthermore, Duijsens et al. (1996) proposed a 400 day timescale for the maximum light amplitude variations that would be caused by a potential magnetic cycle. To explain the variable properties of EZ CMa, a likely scenario is the modulation of a rotating single star with CIRs, as seen in the Sun (Mullan 1986). Additionally, a corotating inhomogeneous density distribution in the stellar wind causing variable transparency could create a variable mass loss. CIRs could emanate from hot, magnetically active regions near the surface (due to a global magnetic field) and would fade away when the star is in the low regime of its magnetic cycle (St-Louis et al. 1995; Duijsens et al. 1996).
In spite of the multiple studies conducted on EZ CMa, a number of questions remain unresolved. Is the variability related to a dynamic, structured, and rotating envelope? If so, what is its shape? Can magnetic fields account for some of the observed characteristics? If so, does EZ CMa harbor a global, large-scale field or does it only have small-scale localized magnetic loops at its surface? Can modern spectropolarimetry be exploited to directly detect the magnetic activity of the star? In an attempt to address these questions, we have collected circular and linear spectropolarimetry of EZ CMa throughout three epochs of observations.
2. OBSERVATIONS AND DATA PROCESSING
We observed EZ CMa at the CFHT using ESPaDOnS.1 This instrument is a bench-mounted high-resolution échelle spectrograph and spectropolarimeter designed to obtain a complete optical spectrum (from 3700 Å to 10500 Å) in a single exposure with a resolving power of about 68,000 in the spectropolarimetric mode. Essentially, light passes through a polarization analyzer and two beams corresponding to orthogonal states of polarization are generated. Then, they are fiber-fed to a stationary and temperature-controlled cross-dispersed spectrograph, and two interleaved spectra are recorded on a CCD. The full spectrum spans 40 orders. To create the Stokes parameter I, the two spectra are added together while the polarization component of the Stokes vector, either V, Q, or U, is formed by basically subtracting the two spectra. Furthermore, one observation of the star (dedicated to circular or linear polarization, Q or U) consists of four successive exposures where, for the second and third, the optics settings are changed so as to exchange the positions of the two spectra on the CCD. This enables the minimization of systematic errors due to misalignments, transmission, seeing effects, etc. (cf. Donati et al. 1997).
Our observations were carried out in 2005 and, through the MiMeS CFHT Large Program,2 in 2009 and 2010. Table 1 summarizes the observing runs. We devoted most of our observing time of WR6 to circular polarization measurements (Vλ) since they are expected to yield a larger Zeeman signature in emission lines than linear polarization in WR6, which in any case for this type of star is strongly dominated by electron-scattering polarization in a globally asymmetric, rotating wind (Schulte-Ladbeck et al. 1991, 1992). Of course, in the wind, the expected field strength is reduced compared to the surface value depending on the radial distance of the spectral line formation zones. For those lines whose peaks can reach many times the level of the continuum, the attainable S/N in Stokes V will be much higher, thus compensating to a large extent for the wind dilution of the line polarized flux by the (circularly) unpolarized continuum flux. Linear polarization exposures (Q and U) were also collected to empirically model the crosstalk between Stokes parameters and compensate for its effect as linear polarization (usually depolarization) across spectral lines could come from scattering off free electrons in a rotation-flattened wind (Schulte-Ladbeck et al. 1992). This is especially important in WR6, where linear continuum (and some line) polarization is known to be significant and variable (Robert et al. 1992). This will be discussed in more detail in Section 4.
Table 1. Journal of EZ CMa Observations
Date | HJD | Phase | texp | S/N pixel−1 |
---|---|---|---|---|
(UT) | (2,450,000+) | (s) | ||
2005 Dec 19 | 3723.853–3724.098 | 0.155–0.220 | 12000 | 1062 |
2005 Dec 20 | 3724.935–3725.123 | 0.442–0.492 | 12600 | 1247 |
2005 Dec 21 | 3725.839–3726.123 | 0.682–0.758 | 14400 | 1824 |
2009 Jan 7 | 4838.919–4839.033 | 0.242–0.273 | 6480 | 1177 |
2009 Jan 8 | 4839.810–4839.923 | 0.479–0.509 | 6480 | 1192 |
2009 Jan 9 | 4840.827–4840.939 | 0.749–0.779 | 6480 | 1336 |
2009 Jan 10 | 4841.818–4841.930 | 0.012–0.042 | 6480 | 1349 |
2010 Feb 25 | 5252.851–5252.935 | 0.155–0.178 | 4968 | 1124 |
2010 Feb 26 | 5253.840–5253.925 | 0.418–0.440 | 4968 | 1132 |
2010 Feb 27 | 5254.804–5254.888 | 0.674–0.696 | 4968 | 1086 |
2010 Feb 28 | 5255.858–5255.944 | 0.954–0.977 | 4968 | 1120 |
Notes. Dates (UT), range of heliocentric Julian Dates, associated range of phases, exposure time (texp), and corresponding signal-to-noise ratio per pixel−1 attained in the continuum for all observations. Expressed in seconds, texp is the total amount of time spent recording Stokes V observations each night, i.e., the total of all sub-exposures recorded in a given night. The phases are computed according to the ephemeris of Lamontagne et al. (1986), using P = 3.766 days, E0 = HJD 2, 446, 153.61. The S/N pixel−1 represents the average of signal-to-noise ratio values attained in each 1.8 km s−1 spectral bin within three continuum regions: 496–510, 507–521, and 556–574 nm.
Download table as: ASCIITypeset image
For the 2005 observations, data reduction was carried out using Libre-ESpRIT, a self-contained data reduction package developed specifically for reducing échelle spectropolarimetric data. This package was first developed by Donati et al. (1997) and later upgraded in order to be used specifically with ESPaDOnS. In 2009 and 2010, data reduction was performed by CFHT's reduction pipeline Upena, which is based on Libre-ESpRIT.
The reduction of ESPaDOnS data proceeds in two general steps.3 The first consists of a geometrical analysis of each order from a sequence of calibration exposures. Their position and shape are derived from a mean flat-field image. The wavelength-to-pixel relationship along and across each spectral order is obtained from a comparison frame (thorium). In the second step, the geometrical information previously found is used to perform an optimal extraction of each object spectrum. Libre-ESpRIT processed spectra include not only the flux and polarization information, but also two check spectra (N1 and N2) and error bars at each wavelength point in the spectrum. The check spectra are null polarization profiles and should be used to help identify spurious polarization signatures. In fact, they are particularly important to check that any potential signatures in polarization profiles (either Stokes V, Q, or U) are indeed real and not the result of instrumentation and/or reduction-related problems (Donati et al. 1997). If such issues are non-existent, N1 and N2 should reflect the noise level present in the observations. More details concerning the extraction technique implemented in Libre-ESpRIT can be found in Horne (1986) and Marsh (1989). In the end, observations obtained with ESPaDOnS and reduced with Libre-ESpRIT (Upena) deliver continuum-subtracted linear or circular polarization spectra consisting of about 214,000 data points, each point corresponding to a velocity bin of 1.8 km s−1.
In all, 70 complete Stokes V spectra of EZ CMa were obtained. During the three observing runs, data were acquired over consecutive nights to cover the 3.766 day cycle. Each of these 70 spectra required series of four independent positions of the wave plate. Each night, we also obtained similar series in each of Stokes Q and U. Table 1 presents the total exposure time spent on nightly Stokes V measurements and the corresponding S/N attained in the continuum of EZ CMa's spectrum.
2.1. Getting the Most Out of Processed Data
In this section, we present the different methods used to increase the detectability of a potential magnetic field. A typical observing run consisted of the following sequence of Stokes parameter exposures: Q, U, V1,..., Vn, U, Q, where n corresponds to the total number of Stokes V exposures recorded during the night. After passing through the reduction pipeline, five quantities are computed at each wavelength; e.g., for a Stokes V exposure, we get IV, V, N1, V, N2, V, and σV. The latter represents the error on all quantities since these are generated using similar manipulations of light beams (Donati et al. 1997).
Stokes Q and U spectra were taken at the beginning and at the end of a Stokes V observing sequence to check if significant variations had occurred in linear polarization while the V exposures were taken. In the event that linear line polarization has changed significantly, Q and U spectra could be, for example, linearly interpolated to correct the individual Stokes V exposures. In actual fact, no significant variations were observed in linear polarization during any sequence, although Q and U spectra do vary over longer timescales.
To reach a sufficiently high S/N, we needed about two hours of exposure time (estimated by the ESPaDOnS exposure-time calculator) on EZ CMa each night. However, we had to split that time into several shorter observations, as the detector would otherwise saturate. Therefore, multi-exposures of circular polarization were taken during each observing sequence. Furthermore, strong emission lines forced us to split the exposures even more than if based on the continuum intensity, depending on the peak line intensity. Once processed by the Upena pipeline, the spectra were then combined to generate one high-S/N Stokes V profile per night. The same reduction was applied to the Stokes Q and U and check spectra (N1 and N2). Processing one order at a time, a weighted mean was calculated at each wavelength with the rms errors added accordingly (see Bevington & Robinson 2003). In the end, three polarization profiles were generated each night. Stokes Q and U measurements were later used to correct Stokes V observations for crosstalk effects (see below).
Figure 1 shows the four Stokes parameter spectra collected during the first night of observation of the 2010 run. The top panel presents the relative intensity spectrum in arbitrary units. When being processed by Upena, the actual number of counts recorded at each wavelength is lost during the optimal extraction of data.4 The bottom three panels show the combined (flux) Stokes parameters V, Q, and U. Another feature of ESPaDOnS échelle data is the order-to-order overlaps. In Figure 1, these typically correspond to regions where the noise level in Stokes V, Q, and U significantly increases. Figure 2 offers a better view of this characteristic property of échelle spectra as it shows the same spectrum of EZ CMa but now focuses only on three orders. The dashed vertical lines indicate where the orders overlap and especially at the extremities, where the noise level is higher. This is a property of spectrographs using échelle gratings in which a spectrum will be underexposed at the ends of the orders since the échelle efficiency varies across each order (Schroeder 1970). To reduce the impact of noisy order extremities, we decided (following the work of Semel & Li 1996) to eliminate the overlap region of each order while at the same time joining together the 40 orders into one continuous spectrum. For each Stokes parameter, the junctions between orders were determined based on their uncertainty profiles. Figure 2 shows an example of this operation done on Stokes V. The top and bottom panels show the Stokes IV profile and its associated error σV as a function of wavelength, with order overlaps, clearly visible (bottom profiles in black and blue). This shows that the error (noise) increases at the beginning and end of each order. Consequently, the order junctions were set at wavelengths where the uncertainty profiles from two successive orders cross each other. The result is a continuous spectrum with no overlaps. The dashed vertical lines in the figure illustrate where the order junctions were cut and joined together. The top curves in these panels show in red the final spectrum and associated error once the orders were trimmed and joined together. The middle panels of Figure 2 show the three Stokes parameters V, Q, and U.
Figure 1. Complete set of Stokes parameters recorded on 2010 February 25. Each parameter is generated by combining multiple exposures taken during the observing sequence. From top to bottom: observed flux in Stokes IV, V (circular polarization), Q, and U (linear polarization) in arbitrary units. The variation in polarized flux seen in Stokes V, Q, and U reflects (1) the varying noise level in the spectral orders and (2) the variation of noise level with flux within spectral lines. In the top panel, the strongest emission lines are identified.
Download figure:
Standard image High-resolution imageFigure 2. Order-to-order overlap of ESPaDOnS échelle data. From top to bottom: observed flux in Stokes IV, V, Q, U (in arbitrary units) and the uncertainty profile (σV) showing five orders and their overlaps (data taken on 2010 February 25). Emission lines of the Pickering series, He ii λ4200, and He ii λ4339 are visible in Stokes IV. The top and bottom panels show (in red) continuous Stokes IV and σV profiles obtained after eliminating the inter-order overlaps. While vertical dotted lines indicate the regions where orders overlap, dashed vertical lines indicate where, based on the σV profile crossings, orders are trimmed and joined together (see the text). Continuous Stokes IV and σV spectra appearing in red (top profiles in the top and bottom panels) were both vertically shifted for clarity.
Download figure:
Standard image High-resolution image3. VARIABLE BINNING
The noise level present in the raw data makes it impossible to distinguish a signal visually. Ignace & Gayley (2003) estimated that for a 3σ detection of a 100 G field, an S/N ∼ 104 was required while the S/N typically reaches S/N ∼ 3000 at the peak of the He ii λ4686 emission line when all observations taken in a single night are combined into a single nightly spectrum, as described above. Since EZ CMa shows several emission lines of various intensities in its spectrum, the S/N per pixel is not constant. In order to obtain the same S/N at every pixel of our Stokes V spectra (i.e., similar error bars per pixel), we then applied a variable size binning. An identical binning procedure was carried out for Q, U spectra, but with slightly lower final S/N in each bin due to the shorter time spent observing them and to the large signal we detect. The method employed to carry out the variable binning is based on Bevington & Robinson (2003). Assume a quantity Xλ with its related uncertainty σλ. Then the weighted mean is defined by

and the error on the weighted mean is

Here the quantity X can represent any Stokes parameters and their respective check spectra. The spectral range over which the summations in Equations (1) and (2) are calculated, i.e., the spectral bin width, is determined according to an S/N threshold. For each element summed in the calculation of and
, the corresponding S/N inside that bin is calculated (see Equation (3)). When a certain threshold is reached, the bin is closed (for all Stokes parameters) and the next bin begins. This binning method generates bins of variable widths but with similar S/N:

where and
are calculated according to Equations (1) and (2), respectively. The aforementioned threshold depends on the spectral region examined. Since emission-line intensities in the spectrum of EZ CMa can vary greatly from one line to another, the threshold chosen to examine a line reaching, e.g., twice the continuum is inadequate to examine a line reaching 10 times the continuum, and vice versa. In fact, choosing the appropriate level of binning is crucial. Bins that are too small do not help to identify any expected signature as the final S/N might be too low while bins that are too wide will enhance cancellation where expected polarization reversals are observed, thus essentially smoothing out the otherwise present signal. Note that it is not necessary to retain the highest spectral resolution of ESPaDOnS, given that W-R winds are turbulent with typical speeds of vturbulence ∼ 100 km s−1 (Lépine & Moffat 1999), equivalent to 1.5 Å at λ = 4500 Å. In the end, the high spectral resolution of ESPaDOnS was not wasted since the high dispersion allowed us to reach without saturation a very high S/N per bin. Variable data-binning yielded good results, as patterns and features then became distinguishable, particularly in the two linear polarization spectra, where such effects are known to occur in EZ CMa (see Figure 3). We will discuss later what level of binning was applied to the data, but, in principle, it should have no impact on integrated quantities (e.g., detection probability and longitudinal magnetic field intensity) computed from the binned data. In the end, the variable binning process produces a certain pattern of pixels of different sizes. Arguably, the resulting pattern depends on the starting and ending wavelengths of the binning procedure. However, we have checked that this dependence does not influence our results. In fact, at the binning levels used in the analysis, the pixel pattern within an emission line is quasi-independent of the spectral range over which variable binning is applied.
Figure 3. Normalized Stokes parameters recorded on 2010 February 25. Since more time was spent recording circular polarization (Stokes V) than linear polarization (Stokes Q and U), these are presented in their relative form (i.e., normalized by their respective intensity flux) so both types of polarization can be compared effectively. From top down: raw Stokes I/Ic, V/I, Q/I, and U/I profiles (left) and their binned equivalent (right) obtained after the variable binning process (S/Nthreshold = 22500). This results in bins of about 2 Å at the line peak of He ii λ4686, which is the most intense emission line of EZ CMa's spectrum. Note that the Stokes V/I binned profile is amplified 10 times for clarity.
Download figure:
Standard image High-resolution image4. CROSSTALK ANALYSIS
It was observed that the ESPaDOnS instrument, while behaving as expected, induced crosstalk between Zeeman parameters. In our case, circular polarization measurements clearly appear to contain a fraction of linear polarization. Since the shape in V profiles was similar to a linear combination of Q and U, we deduced that we have significant crosstalk from Q, U to V and not vice versa. Through the years and through modifications to the instrument, the origin of this crosstalk was successfully identified by the ESPaDOnS support team, and it was considerably reduced but not completely suppressed. EZ CMa shows clear linear line polarization signatures of typically ∼0.2% at line peak, in both Stokes Q and Stokes U. Since Stokes V signatures at the 0.01%–0.1% level are expected (Gayley & Ignace 2010), we could not neglect the existence of crosstalk from linear to circular polarization, during our observing runs, given the strength of linear polarization, which is an order of magnitude stronger than the expected circular polarization. This is clearly illustrated by Figure 4, in which the normalized Stokes parameters V, Q, and U are shown for the three different nights of the 2005 observing run. At this epoch, the reported crosstalk factor was approximately 7%, and it is obvious, particularly for the first two nights of observations, that the observed V/I profiles resemble a linear combination of the observed profiles seen in Q/I and U/I. To make up for a possible contamination of Stokes V measurements, we adopted the pessimistic but prudent conservative view that the entire V signal consisted of crosstalk from Q and U and then proceeded as follows to remove it. First, each Stokes parameter is normalized by the corresponding unpolarized flux recorded during the exposure sequence (cf. Section 2.1). Working with normalized Stokes parameters ensures that each spectral pixel reflects the fraction of recorded light that is polarized (circularly or linearly) relative to the unpolarized Stokes I parameter. Then, we assumed a linear and wavelength-dependent relation for the crosstalk contamination as follows:

The five parameters aQ, bQ, aU, bU, and δ in Equation (4) were assumed to be constant from night to night at any given epoch. Our results for the three observing runs are presented in Table 2. For a given run, the relative V profile of the strongest emission lines, He ii λ4686, He ii λ5411, He ii λ6560, and N iv λ7111, were simultaneously fitted to Equation (4) for each of the nightly spectra, taking the model value (V/I)' to be the closest possible to the observed V/I parameter. We then defined αλ = aQλ + bQ and βλ = aUλ + bU to be Fλsin 2Ωλ and Fλcos 2Ωλ, respectively. Consequently, we refer to as the crosstalk factor at one specific wavelength and
as the rotation angle of the Q–U plane relative to some arbitrary but fixed internal axis in the V system (see Table 3). In Equation (4), the coefficient δ represents a constant offset in circular polarization that we assume to be independent of wavelength. By default, the reduction pipeline subtracts the continuum polarization from the polarization spectrum, as well as from the null spectra. Typical emission lines found mostly in young stars are excluded when determining the continuum regions, but if a lot of other emission lines are present, as is the case for EZ CMa, the continuum normalization will not exclude those lines, yielding inaccurate results.5 When examining reduced data of EZ CMa that were automatically normalized by the continuum intensity, it is obvious that the automatic removal process fails to correctly do so. We thus suspect that the automatic removal of continuum polarization might also be erroneous. Hence, the δ coefficient in Equation (4) compensates for the fact that the reduction pipeline fails to adequately remove the continuum polarization.
Figure 4. Spectra in all four normalized Stokes parameters for the three different nights of the 2005 observing run. The top panel shows the nightly mean profile of the He ii λ4686 emission line. The next three panels show in black the normalized and binned Stokes V (vertically shifted for clarity and zoomed in y-scale), Q, and U parameters for that profile. The polarization profiles are binned to a constant S/N (∼22, 500) per bin, and the resulting mean error bar appears at the top-right corner of each frame. Note that while Stokes I is normalized by the continuum level (Ic), the Q, U, and V spectra are normalized to the total wavelength-dependent intensity obtained with the same exposures used to obtain a given Stokes parameter (IV, IQ, IU). In the second panel from top, the crosstalk model (F = 6.98% ± 0.26% and Ω = −22° ± 1° at line center) based on Q/I and U/I in the bottom two rows is shown in red (middle profile), while the residual spectrum obtained by subtraction of the modeled from the observed signal (also vertically shifted) is shown in blue (bottom profile). Dashed lines represent the zero-polarization level.
Download figure:
Standard image High-resolution imageTable 2. Crosstalk Parameters Computed from Stokes V Profiles
Observing Run | Crosstalk Parameters | ||||
---|---|---|---|---|---|
(year) | aQ | bQ | aU | bU | δ |
(× 10−5 nm−1) | (× 10−2) | (× 10−5 nm−1) | (× 10−2) | (× 10−5) | |
2005 | −0.39 ± 0.32 | −4.75 ± 0.17 | −3.53 ± 0.39 | 6.59 ± 0.22 | −0.94 ± 0.06 |
2009 | 6.05 ± 0.42 | −5.96 ± 0.23 | −5.11 ± 0.21 | 1.26 ± 0.12 | −1.26 ± 0.12 |
2010 | −2.11 ± 0.29 | 1.22 ± 0.16 | 2.35 ± 0.20 | −1.44 ± 0.11 | −2.18 ± 0.16 |
Notes. Four emission lines (He ii λ4686, He ii λ5411, He ii λ6560, and N iv λ7111) were used to model the crosstalk signature according to Equation (4). Results for all epochs of observations are presented.
Download table as: ASCIITypeset image
Table 3. Crosstalk Factors Computed for the Four Strongest Emission Lines
Observing Run | Crosstalk Factor Fλ(%) | ||||
---|---|---|---|---|---|
(year) | He ii λ4686 | He ii λ5411 | He ii λ6560 | N iv λ7111 | ESPaDOnS |
λ0 ≃ 4688 Å | λ0 ≃ 5415 Å | λ0 ≃ 6563 Å | λ0 ≃ 7116Å | (Reported) | |
2005 | 6.98 ± 0.26 | 6.82 ± 0.27 | 6.59 ± 0.30 | 6.48 ± 0.31 | ∼7 |
2009 | 3.34 ± 0.29 | 3.11 ± 0.29 | 2.93 ± 0.28 | 2.94 ± 0.26 | ∼2–4 |
2010 | 0.41 ± 0.17 | 0.18 ± 0.17 | 0.19 ± 0.23 | 0.36 ± 0.23 | ≲ 1 |
Notes. The crosstalk factors are computed from observations obtained at three different epochs and are compared with their respective reported values.
Download table as: ASCIITypeset image
Figure 4 shows the rectified profile for the strongest line in our spectrum, i.e., He ii λ4686, as well as the three other normalized Stokes parameters (in black) for the four nights of the 2005 CFHT run. It shows, in red, the above-mentioned model spectrum for the crosstalk in V/I and, in blue, the residual V/I spectrum after the removal of the model crosstalk. The observed V/I spectra (in black) and their residuals were vertically shifted for clarity. Note that the above method assumes that the crosstalk has not varied over the time interval of an observing run, that is at most, over four consecutive nights of observations. This assumption is arguably incorrect since in 2009 May the crosstalk was observed to be quite variable, sometimes showing large variations within a single night (Barrick et al. 2010). After the changes made to the spectropolarimeter in the summer and fall of 2009, the crosstalk level was reduced to below 1% but still shows a small level of variability due to the variable observing conditions (see the latest news about ESPaDOnS' crosstalk6 and Barrick et al. 2010). Our constant crosstalk assumption seems thus reasonable for data recorded in 2010 but less so for the two other sets of observations obtained in 2005 and 2009. On the one hand, the nightly crosstalk level is unpredictable and our knowledge of the various sources responsible for its variability does not allow us to model its exact behavior yet. On the other hand, the level of linear polarization within line profiles is high, and given the existence of crosstalk, its impact on recorded Stokes V profiles cannot be neglected (see Figure 4). Modeling the crosstalk using all consecutive nights of an observing run might not determine its exact nightly value, but we believe that our methodology provides a good estimate of the mean crosstalk level present at that time. This is supported by the good agreement between the crosstalk factors we obtained and the ones estimated by the ESPaDOnS team at the time the observations were taken (see Table 3). Furthermore, we checked that the crosstalk removal process did not introduce variations similar to the expected Stokes V signal (see Figure 3 of Gayley & Ignace (2010) for the expected Stokes V profile within emission lines formed in a hot-star wind threaded with a weak radial magnetic field). To do so, we examined the fitted crosstalk model prior to subtracting it from the observed Stokes V profiles. In the context of this study, the crosstalk level was highest during the 2005 run. Even at this level, Figure 4 shows that the crosstalk model takes the form of a smooth, continuous function within the emission-line profile. Conversely, when the crosstalk level was at its lowest (in 2010), the model is almost constant throughout the line (see the second row of Figure 5). In any case, we are confident that the crosstalk removal process did not introduce any artificial variations that could be mistaken for a real signal by the subsequent analysis.
Figure 5. Normalized Stokes V, NV, 1, and NV, 2 parameters for the four different nights of the 2010 observing run. In the bottom three rows, crosstalk models (middle profiles) computed from Equation (4) and using parameters presented in Tables 3 and 4 are shown in red, while the residuals (bottom profiles) are shown in blue. The observed data (top profiles, in black), along with the residuals, are vertically shifted for clarity.
Download figure:
Standard image High-resolution image4.1. Null Profiles Affected by Crosstalk?
While it is known and reported that crosstalk affects measurements made with ESPaDOnS, the two null profiles are not expected to be influenced by such an effect. We discuss here a curious characteristic observed in NV, 1 and NV, 2. As mentioned previously, two null spectra for each Stokes parameter are generated during an observing sequence. Theoretically, these should show a flat profile centered at the zero polarization level. However, as shown in Figure 5, NV, 1 and NV, 2 profiles exhibit structure inside the spectral line. Moreover, those patterns are sometimes of amplitude comparable to or larger than the Stokes V signatures. Furthermore and perhaps more obvious is the fact that NV, 1 and NV, 2 do not seem to be centered at zero as they are supposed to be but appear shifted in polarization level. This suggests that, at the very least, a constant amount of spurious polarization is added to the null spectra when these are generated. Since signatures in NV, 1 and NV, 2 also seem to follow the shape of the Stokes Q and U profiles (much like what was observed in Stokes V before the crosstalk removal; see Figure 4), perhaps crosstalk effects are also present in the null spectra. Mueller matrix calculations allow us to verify that there is a potential for crosstalk effects to appear in the null spectra (e.g., if the Fresnel rhombs and their respective positions are not without imperfections; see the Appendix). Since the four sub-exposures needed to compute Stokes V are independent, i.e., different combinations of their azimuthal axis are used, an error on any of the prism positions can yield the presence of Q and/or U in V, NV, 1, and NV, 2. Moreover, rotational variability and stress birefringence from optical elements located before the polarization module could also be an additional source of crosstalk, even though these elements have been studied and improved throughout the years. According to the ESPaDOnS support team, some of the crosstalk left, along with its variation, is due to the atmospheric dispersion corrector, which probably still exhibits stress birefringence, and since its configuration changes as a function of time (as a function of the telescope's position), this small stress birefringence introduces a small level of variable crosstalk. With that in mind, we have decided to be cautious and eliminate crosstalk from NV, 1 and NV, 2 using the same method described in Section 4. The crosstalk parameters are shown in Table 4. When parameters aQ, bQ, aU, and bU are considered, there is no systematic trend present in the results obtained from Stokes V that appear in NV, 1 or in NV, 2. They are, however, of similar magnitude. Since Stokes V is fundamentally different in nature (what it represents and how it is generated) than the null spectra—it potentially contains a polarization signal intrinsic to the star—this is expected as the crosstalk removal method is aimed at eliminating all signal present in a given parameter. On the other hand, the δ parameter value obtained from Stokes V, NV, 1, and NV, 2 should be consistent because it is independent of Q and U and constant within the line profile. Comparing δ values presented in Tables 2 and 4 confirms this.
Table 4. Crosstalk Model Parameters Computed from Stokes null Profiles
Observing Run | Crosstalk Parameters | ||||
---|---|---|---|---|---|
(year) | aQ | bQ | aU | bU | δ |
(× 10−5 nm−1) | (× 10−2) | (× 10−5 nm−1) | (× 10−2) | (× 10−5) | |
−N1 − | |||||
2005 | 0.51 ± 0.32 | −1.08 ± 0.17 | −3.27 ± 0.39 | 0.53 ± 0.22 | −0.86 ± 0.04 |
2009 | 11.34 ± 0.42 | −7.86 ± 0.23 | 2.74 ± 0.21 | −1.91 ± 0.12 | −1.56 ± 0.14 |
2010 | −1.90 ± 0.29 | −0.44 ± 0.16 | −1.83 ± 0.20 | −0.32 ± 0.11 | −1.77 ± 0.09 |
−N2 − | |||||
2005 | 3.09 ± 0.32 | 2.19 ± 0.17 | −17.41 ± 0.39 | 11.31 ± 0.22 | −0.47 ± 0.08 |
2009 | 9.13 ± 0.42 | −4.43 ± 0.23 | −4.29 ± 0.21 | 4.27 ± 0.12 | −1.47 ± 0.09 |
2010 | −14.94 ± 0.29 | 9.27 ± 0.16 | −4.07 ± 0.20 | 3.57 ± 0.11 | −0.69 ± 0.18 |
Notes. The same modeling technique applied to Stokes V observations is used on both NV, 1 and NV, 2 spectra. Results for all epochs of observations are presented.
Download table as: ASCIITypeset image
Within most of the emission line, the crosstalk model behaves like a polarization constant that centers the null profiles at the zero polarization level once it is subtracted from the observed data. Equations (A24)–(A26) along with Equations (A27)–(A34) (see the Appendix) indicate that the net crosstalk effect on NV, 1 and NV, 2 due to positional errors of the Fresnel rhombs is likely more complex than a simple linear combination of Q and U. Thus, the model used to remove the crosstalk in the two check spectra is likely oversimplified. Nonetheless, since terms in Q and U represent the most important contributions to crosstalk, the model still is a good first-order approximation.
5. MAGNETIC ANALYSIS
5.1. Zeeman-splitting Polarization
As mentioned in Section 3, we have used a binning procedure aimed at reducing noise and thus favoring the detection of any potential signal due to the presence of a magnetic field. The crosstalk removal could, at worst, eliminate a fraction of the Stokes V profile intrinsic to the star. However, using the same crosstalk parameters for all the nights in one run helps to minimize this because there is no reason to believe that any intrinsic, variable polarization would exhibit the same pattern as the crosstalk, which depends entirely on the behavior of Q and U. Nonetheless, we checked (by visual inspection) that the crosstalk model was a smooth, continuous function within the line profile and that it did not resemble, in shape and amplitude, the Stokes V signature predicted by Gayley & Ignace (2010). Moreover, except for the 2005 data (see Figure 4), the crosstalk model is approximatively constant throughout the line profiles. The presence of a magnetic field in the wind of EZ CMa observed via Zeeman splitting was looked for in two ways: (1) calculation of the reduced-χ2 within the Stokes V line profile and (2) measurement of the longitudinal field Bl directly from the first-order moment of the Stokes V profile. In the first method, the reduced-χ2 within the Stokes V line profile is computed to verify if the signal diverges significantly from a flat, null profile characteristic of a non-magnetic star. To diagnose the presence of a magnetic field in EZ CMa, we followed the detection diagnostic of Donati et al. (1992) and Donati et al. (1997). A circularly polarized signature is definitely detected if the associated detection probability is larger than 99.999%. A detection probability between 99.9% and 99.999% corresponds to a marginal detection, and below 99.9%, no formal detection is reported. Moreover, any positive detection across the spectral line is considered real if the detection probabilities calculated in both null profiles and in the continuum are below 99.9%.
For reasons discussed in Section 3, the level of binning that is applied to the data is important as it could influence the detectability of any potential Stokes V signature present inside the line profile. Because the expected signatures are extremely small in amplitude (Donati et al. 1997), especially for spectral lines formed in a stellar wind (Gayley & Ignace 2010), they need to be measured with high accuracy, i.e., with relative noise levels lower than 10−4. Since choosing a different S/Nthreshold for each spectral line is somewhat arbitrary, variable binning was thus conducted on the data so that individual error bars reflected a 0.01% uncertainty. With such a relative noise level, a strong magnetic field (∼102–3 G) present in the wind of EZ CMa would yield Stokes V signatures detectable at the 3σ level. On the other hand, the presence of a weaker field at ≲ 100 G level requires an even higher S/N level to be clearly detected. Ignace & Gayley (2003) and Gayley & Ignace (2010) predict that such a magnetic field intensity, in a split monopole configuration, would generate Stokes V line profiles reaching ∼0.01%–0.04% in amplitude for emission lines formed in the wind and viewed under ideal conditions. Based on these considerations, we computed the detection probability within the Stokes V line profiles after binning the data so that the relative error bars reflected σV ≃ 10−4. Table 5 reports those detection probabilities calculated for the Stokes V profiles of He ii λ4686, as well as for the two null spectra, NV, 1 and NV, 2. We present only the results obtained from the He ii λ4686 emission line because it is the strongest emission line in the spectrum with the smallest error bars. Although this might be compensated for by a lower signal, since it is formed farther from the star, no other lines nor a combination thereof show any better signal.
Table 5. Detection Probabilities Computed for the He ii λ4686 Emission Line
Year | Phase | PV | ![]() |
![]() |
---|---|---|---|---|
(ϕ) | (%) | (%) | (%) | |
2005 | 0.155 | 65.4 | 83.4 | 98.8 |
0.442 | 59.1 | 78.4 | 99.1 | |
0.682 | 62.3 | 37.7 | 38.7 | |
2009 | 0.242 | 72.7 | 99.779 | 78.2 |
0.479 | 94.4 | 99.843 | 77.5 | |
0.749 | 33.9 | 61.5 | 3.0 | |
0.012 | 87.5 | 90.3 | 59.3 | |
2010 | 0.155 | 83.7 | 85.8 | 68.1 |
0.418 | 28.7 | 81.1 | 67.8 | |
0.674 | 10.8 | 95.2 | 64.4 | |
0.954 | 90.0 | 35.8 | 50.0 |
Notes. Values for PV, , and
represent the detection probabilities derived from within the Stokes V, N1, and N2 line profile, respectively, and binned in phase according to the ephemeris of Lamontagne et al. (1986). The results are presented at the three-decimal precision level when the probability reaches 98% so the criteria of Donati et al. (1997) can be adequately evaluated. The phase ϕ corresponds to the first observation taken each night, and detection probabilities are obtained from profiles binned to a precision of σV ≃ 10−4.
Download table as: ASCIITypeset image
In a few instances, the criteria are met to report, at best, a marginal detection in Stokes V (99.915% for He ii λ5411 on the third night of the 2005 observing run; 99.904% and 99.964% for N iv λ7111 on the third and fourth nights of the 2009 observing run, respectively). However, no systematic detections can be reported for all spectral lines or for a given emission line at all phases. Therefore, this test does not allow us to infer the presence of a magnetic field, as most of the Stokes V profiles are not significantly different (according to the χ2 statistics) from a null, non-magnetic signature. Strangely, marginal detections are also achieved on a few occasions within check profiles and continuum regions. This would suggest that excess noise in the data can sometimes randomly affect the shape of the signal, leading to an erroneous marginal detection on the basis of a χ2 test when compared to a Stokes V = 0 profile. As a whole, these results probably indicate that if there is a large-scale magnetic field in the wind of EZ CMa, it is not strong enough to consistently generate detectable signatures. By binning the data further to increase the S/N, any potential signature could consequently be smoothed out, thus lowering the upper detection limit. Also, the approach taken to eliminate crosstalk effects might remove a fraction of a real magnetic signature and hence decrease its detectability. In the end, the fact that no Zeeman-splitting signature is, in general, unambiguously detected points toward the following conclusion: any magnetic field present in the wind of EZ CMa (in the line formation zones) is too weak for the detection capabilities of both the instrument and the methods used in this study.
Of course, at this stage no information about the strength and structure of a potential magnetic field is obtained. Ionization stratification in the wind of EZ CMa (Hillier 1987; Schulte-Ladbeck et al. 1995; Herald et al. 2000) implies that emission lines present in the spectrum are formed in different conditions (e.g., different distances from the stellar surface, different velocities), and hence line-emitting regions would be threaded by different field intensities, no matter what large-scale magnetic configuration could be present. Moreover, emission lines often suffer blends with other emitting ions, which can dilute the circular polarization component of the dominating emitting ion. For these reasons, averaging the circularly polarized profiles from different individual emission lines (as is done for stars showing a large number of photospheric absorption lines) is inadequate. Thus, all results presented in this paper must come from the analysis of single emission lines. Consequently, this could explain why no significant detections are made according to the strict criteria mentioned above, which are adapted for profiles analyzed by the least-squares deconvolution method. In the context of hot stars, this technique combines several (∼101–2) photospheric absorption lines in order to increase the detectability of a potential Stokes V signal (Donati et al. 1997) and has proven its efficiency of exposing magnetic signatures that are hidden by noise within single line profiles. Donati et al. (1992) used different criteria when analyzing individual lines, and so perhaps the analysis of W-R emission lines should be based on better adapted criteria. Nonetheless, we feel that analyzing single emission lines of EZ CMa with the highest standards represents the safest, most conservative way of detecting a magnetic field. Moreover, eye inspection of Stokes V data led us to conclude that profiles resembling an organized, s-wave type, Zeeman signature do not necessarily result in a marginal (or even significant) detection, whereas unorganized, relatively flat profiles with a few divergent data points sometimes do. We feel that the search for a Zeeman-splitting signature by computing the χ2 statistics within individual emission lines would yield a positive detection only if a strong, organized magnetic signature is present in the data.
The other technique used to evaluate the presence of a magnetic field (and its amplitude) in the wind of EZ CMa is the determination of its mean longitudinal intensity by calculating the first-order moment of the Stokes V profile within the line according to

(Rees & Semel 1979; Mathys 1989; Wade et al. 2000), where the wavelength λ of the center of gravity of the line is in nm, geff is the effective Landé factor, and Bl is expressed in gauss. The uncertainties associated with Bl were computed by propagating the errors of each spectral bin through Equation (5). The center of gravity (from which the velocity was determined) was calculated according to the following equation:

where Wλ is the equivalent width of the line and I(λ) is the unpolarized line profile. The effective Landé factors were computed through the formula

where g1, g2 and J1, J2 are, respectively, the Landé factors and total angular momentum quantum numbers of the involved levels of the line transition. For the emission lines considered in this work, the Landé factor of a certain level was computed by the LS-coupling approximate formula:

where L and S are the orbital angular momentum and spin quantum numbers of the considered level, respectively, and subscript i refers to the transition level of interest. Using Equations (7) and (8), the effective Landé factors for He ii λλ4686, 5411, 6560, and N iv λ7111 emission lines are geff = 1.07, 1.06, 1.06, and 1.17, respectively. The integration ranges for both λ and Bl were chosen to be symmetric about the center of gravity of the line going from −2000 to 2000 km s−1. These limits of integration were chosen to encompass all information about the magnetic field in the Stokes V profile while avoiding the inclusion of excess continuum and line blends outside of the profile. In the end, the longitudinal field measured from Stokes V is detected significantly (at the 3σ level and over) if |Bl|/σ ⩾ 3. For the sake of conciseness, only the results for the He ii λ4686 emission line are presented in Table 6 since a longitudinal magnetic field is significantly detected at the 3σ level (and higher) within the observed Stokes V profiles on only a few occasions (one such instance can be found in Table 6). The only other significant detection occurs for the He ii λ5411 line, on the third night of the 2009 observing run (Bl = −128 ± 35 G ⇒ z = 3.7). Also, the first moment technique, when applied to both N1 and N2, never results in a detection, as expected.
Table 6. Longitudinal Field Values Calculated for the He ii λ4686 Emission Line
Year | Phase | Stokes V | Null N1 | Null N2 | |||
---|---|---|---|---|---|---|---|
ϕ | Bl ± σB | zV | Bl ± σB | ![]() |
Bl ± σB | ![]() |
|
2005 | 0.155 | −20 ± 17 | 1.2 | −22 ± 17 | 1.2 | 21 ± 17 | 1.2 |
0.442 | −13 ± 15 | 0.8 | 2 ± 15 | 0.1 | 37 ± 15 | 2.5 | |
0.682 | 29 ± 11 | 2.7 | −17 ± 11 | 1.6 | −19 ± 11 | 1.7 | |
2009 | 0.242 | −8 ± 16 | 0.5 | 5 ± 16 | 0.3 | −12 ± 16 | 0.8 |
0.479 | 52 ± 16 | 3.2 | 41 ± 16 | 2.5 | −2 ± 16 | 0.1 | |
0.749 | 6 ± 14 | 0.4 | 38 ± 14 | 2.7 | −6 ± 14 | 0.4 | |
0.012 | −18 ± 15 | 1.3 | 1 ± 15 | 0.1 | 8 ± 15 | 0.6 | |
2010 | 0.155 | −24 ± 17 | 1.4 | −7 ± 17 | 0.4 | −8 ± 17 | 0.5 |
0.418 | −11 ± 17 | 0.7 | 16 ± 17 | 1.0 | −9 ± 17 | 0.5 | |
0.674 | −27 ± 18 | 1.6 | 14 ± 17 | 0.8 | −1 ± 17 | 0.1 | |
0.954 | 8 ± 16 | 0.5 | 16 ± 16 | 1.0 | 25 ± 16 | 1.6 |
Notes. The detection significance of the longitudinal magnetic field is expressed by z = |Bl/σB|. The longitudinal magnetic field is given in gauss, and ϕ corresponds to the phase of the first observation taken each night according to the ephemeris of Lamontagne et al. (1986). The significant detection (z > 3σ) appears in bold font.
Download table as: ASCIITypeset image
Figure 6 illustrates the phase variation of the longitudinal field for all three epochs of observations and for all four emission lines analyzed. A least-squares fit of a cosine curve to the data was performed using the form Bl(ϕ) = B0 + B1cos (2π(ϕ − ϕ0)). The resulting parameters along with the reduced χ2 value are presented in Table 7. The least-squares fit was applied assuming that the variations are continuous and consistent from one epoch of observations to another. This continuity has not been confirmed, but it still represents a reasonable hypothesis. According to the least-squares statistic, the longitudinal field is not significantly different from zero and the variation of the field is detected at only a 2σ level. Based on this test, there is not enough evidence to infer the presence of a magnetic field in the wind of EZ CMa. Moreover, the reduced χ2 statistic obtained through the curve fit is essentially the same as that computed for the magnetic null hypothesis, i.e., B = 0. These calculations were also applied to the N profiles, and the results suggest that they are also compatible with a null profile, as expected. For completeness, we employed a Kolmogorov–Smirnov test to investigate if the distribution of the detection significance from Stokes V (zV) is significantly different from that derived from the null profiles, N1 and N2. For the cumulative distributions of zV and , we obtain D = 0.1363, and for zV and
, D = 0.2045, indicating that the null hypothesis (that the distributions are the same) can be rejected at confidence levels of about 36% and 79%. In other words, the two-sided Kolmogorov–Smirnov test indicates that the distribution of zV does not appear to be significantly different from the
and
distributions. This supports our claim that the longitudinal magnetic field test does not provide enough evidence to infer the presence of a detectable magnetic field.
Figure 6. Phase variation of the longitudinal field. For all three epochs of observations and all emission lines considered in this study, the top panel presents the longitudinal field values measured within Stokes V profiles, whereas the bottom two panels show values obtained from within N1 and N2 profiles, respectively. The symbols represent the different emission lines, and the epochs of observations are color coded: 2005 data are in green, 2009 in red, and 2010 in blue. At the bottom of each panel, the resulting parameters of the cosine curve, Bl(ϕ) = B0 + B1cos (2π(ϕ − ϕ0)), least-squares fit are presented.
Download figure:
Standard image High-resolution imageTable 7. Results of the Longitudinal Field Variation Cosine Fit
Parameters | Stokes V | Null N1 | Null N2 |
---|---|---|---|
B0(G) | −3 ± 3 | 5 ± 3 | −3 ± 3 |
B1(G) | 8 ± 4 | 3 ± 4 | 5 ± 4 |
ϕ0 | 0.1 ± 0.1 | −0.1 ± 0.2 | −0.2 ± 0.1 |
χ2ν (fit) | 1.82 | 1.11 | 1.24 |
χ2ν (B = 0) | 1.81 | 1.11 | 1.21 |
Notes. The best parameters of the cosine curve are computed through a χ2 goodness-of-fit test for the Stokes V, N1, and N2 observations. The last two rows present the resulting χ2ν applied to the cosine curve and to a null B = 0 line, respectively.
Download table as: ASCIITypeset image
The longitudinal magnetic field considered here is only used as a statistical indicator of the field strength. It is important to note that the longitudinal magnetic field is not used as the primary diagnostic of the presence of a magnetic field. This is because of a number of considerations. First, a variety of magnetic configurations can produce a null mean longitudinal field component, one for which the first-order moment of the Stokes V profile is zero (i.e., a profile roughly symmetric about the center of gravity of the emission-line profile). Nonetheless, almost all of these configurations generating a null mean longitudinal field component will produce a detectable Stokes V signature in the line profile, given that the magnetic field is strong enough. In the next section, we will discuss how this might actually be the type of profile expected for the magnetized stellar wind of W-R stars. Second, Equation (5) was derived for magnetic dipoles in rotating stellar photospheres under some simplifying assumptions. It is thus important to verify if it can be applied to W-R star winds in which the field topology is not dipolar and where the dominant spectral line broadener is not in rotation. The derivation of Equation (5) assumes that the emergent Stokes V line profiles are antisymmetric about their center. As shown by Gayley & Ignace (2010), this is expected in the context of the present study even if the magnetic field topology in W-R stellar winds resembles a split monopole rather than a dipole. The derivation of Equation (5) shows that it is independent of the projected rotational velocity of the star under very general conditions, i.e., the effect of the rotation on the line profiles has been fully accounted for and the first-order moment technique takes advantage of the rotational Doppler effect to get an enhanced diagnosis (Mathys 1988, 1989). However, the Zeeman broadening produced by a line observed in the visible band and a modest magnetic field is much smaller than the velocity broadening in the winds of hot stars. This leads to severe polarimetric cancellation of the σ components of the standard Zeeman triplet, and hence the ability to detect the longitudinal magnetic field through the Zeeman effect depends generally on the amount of Zeeman shift relative to the relevant broadening processes (Ignace & Gayley 2003). As described by these authors, this cancellation is, however, mitigated by velocity gradients in the wind, which allows for some Zeeman signals to persist in the line profile and produce an antisymmetric Stokes V profile about its center. What the exact physical meaning of Equation (5) is, in the context of W-R stars, is not clearly established, and thus it cannot a priori be taken for granted that it is the average over the emitting volume of the line-of-sight component of the magnetic field. Furthermore, Equation (5) should be used for the analysis of single spectral lines that are effectively unblended (Mathys 1989). In the case of W-R stars, their strong stellar winds generate very broad lines that often consist of a blend of different ion species. Even if one ion usually dominates (in intensity) at the wavelength of interest, contributions from other species can affect the results of the first-order moment technique. To minimize the contributions due to other spectral lines on the edges of the line profile, the limits of integration were chosen to only include the information of the Stokes V profile within the emission line. Moreover, Mathys (1989) states that the mean longitudinal field obtained from the observation of different spectral lines is not a priori identical, especially for an element inhomogeneously distributed over the star. This is also relevant in the case of EZ CMa, and possibly for W-R stars in general, where ionization stratification occurring in its stellar wind (Hillier 1987; Schulte-Ladbeck et al. 1995; Herald et al. 2000) implies that different spectral lines are formed in regions where the local field strength varies due to the radial dependence of the magnetic field intensity. While the spectral lines considered in this work are, in first approximation, homogeneously distributed over the stellar surface (the radial extent of individual formation zones is larger than the stellar core radius), their measurements may still yield discrepant field values. If different radial distributions are found for the various ions considered, as appears to be the case for EZ CMa, then Equation (5) will depend in a different manner on the spectral line, leading to a discordant value of the longitudinal magnetic field. As mentioned in Section 1.1, the variations observed in EZ CMa are epoch dependent. If the magnetic field is also subject to such a dependency, the fit of a cosine curve through the phased-bin variations of its longitudinal value (recorded over a five-year span) should be interpreted with extra care, even more so when a straight line (B(ϕ) = 0) yields a similar fit according to the reduced-χ2. Finally, another shortcoming of this technique may come from the process of line formation in the winds of W-R stars. The first-order moment method is based on several hypotheses (e.g., Milne–Eddington approximation, weak-line approximation, and LTE) that are not relevant for radiation-driven winds from hot stars. In all, the determination of the longitudinal magnetic field using Equation (5) appears to be an approximation of its true value. Certainly, a better understanding of spectral line formation in the atmospheres of W-R stars and how the moment technique should be modified for different ions formed in a hot star wind would help understand the exact physical meaning of Equation (5). Still, Figure 6 illustrates that when the longitudinal field values derived from all four emission lines (for all three epochs of observations) are combined together, the cosine fit finds a field value at a ∼2σ significance level in Stokes V, while in the null N1 and N2, it is significant at ∼1σ. Even if 2σ is not statistically significant enough to report a detection, it is worth nothing that, when all the above considerations are taken into account, the cosine-fitted longitudinal field reaches such a significance level while, for N1 and N2, it is around unity, as expected. In summary, the longitudinal magnetic field variation does not provide enough evidence to infer the presence of a magnetic field, but it should not be on its own interpreted as the absence of magnetism in EZ CMa. We look closer into this below.
5.2. Potential Magnetic Field Geometry
Despite the negative results on the presence of a detectable global magnetic field in EZ CMa using techniques relying on the amplitude of the Stokes V signature and its (non)symmetric nature, it is useful to take yet another approach: fitting a simple model to the data. To this end, we assume that the magnetic field of EZ CMa can be described by the split monopole model of Gayley & Ignace (2010), allowing us to place an upper limit on its intensity and to investigate its potential geometry. The first step taken by these authors to establish a model describing the effect of a magnetic field on the stellar wind of hot stars came earlier when they incorporated the weak-field longitudinal Zeeman effect in the Sobolev approximation (Ignace & Gayley 2003). They derived expressions for the circularly polarized emission from a magnetized wind. Particularly interesting in the case of W-R stars, they investigated the split monopole configuration to schematically illustrate the expected polarized profile. They found that the signature resembles an s-wave pattern and that for a surface field of about 100 G (seen pole-on), the largest polarizations peaking at approximately 0.05% in Stokes V result for optically thin but strong recombination emission lines. More recently, Gayley & Ignace (2010) published the results of an improved model of Stokes V(λ) profiles for emission lines formed in hot-star winds threaded with a weak magnetic field. For the most favorable orientation (i.e., pole-on), they found that the circularly polarized profile has a characteristic heartbeat shape exhibiting multiple sign inversions across the spectral line (see Gayley & Ignace 2010, Figure 3). For a wind magnetic field intensity of B ≃ 100 G and at depths where the wind speed is v ≃ 100 km s−1, the overall scale of V/I is of the order of 0.1%.
A split monopole is considered under the assumptions that the magnetic field has been radially combed by the wind and rotation does not play a central role. It is important to note that the overall competition between the wind's mechanical energy density and the magnetic energy density can be described by the magnetic wind confinement parameter , where B⋆ and R⋆ are, respectively, the equatorial magnetic field and the radius at the star's surface. Based on the work of Hamann et al. (2006), R⋆ corresponds to a radial optical depth of τRoss = 20. In regions far from the stellar surface, ud-Doula & Owocki (2002) demonstrated that the magnetic field lines are stretched out to follow the approximately radial wind outflow since the wind dominates the interaction. However, closer to the surface where the wind velocity is smaller, a sufficiently strong magnetic field can channel the wind plasma along closed field lines and hence significantly influence the wind flow. Quantitatively, for η⋆ < 1, the field is fully opened by the wind outflow. For stronger confinements, η⋆ > 1, the magnetic field remains closed over a limited range of latitudes and heights above the equatorial surface but eventually is opened into a nearly radial configuration at large radii. ud-Doula & Owocki (2002) and Owocki & ud-Doula (2004) also demonstrated that, for confinements as small as η⋆ = 0.1, the magnetic field can have a significant influence in enhancing the density and reducing the wind speed near the magnetic equator. In the case of EZ CMa, η⋆ < 1 requires a magnetic field intensity of B⋆ < 4 kG and η⋆ = 0.1 would imply a field strength of B⋆ ∼ 1.3 kG, for the following wind and stellar parameters:
, v∞ = 1700 km s−1, and R⋆ = 2.65 R☉ (Hamann et al. 2006). Because of the enormous mass-loss rates of W-R stars, even relatively small values of η⋆ require large field strengths. Hence, in the weak-field limit, a split monopole configuration, resulting from the interaction of a dipolar field with a strong stellar wind, is a relatively good representation of the magnetic field present in the wind of W-R stars.
When modulated with phase, the model of Gayley & Ignace (2010) can be described by four parameters linked to the magnetic field geometry: (1) the viewing inclination angle of the stellar rotation axis, i0; (2) the obliquity angle β between the field axis and the rotation axis; (3) the phase of closest approach of the magnetic pole to the line of sight, ϕ0; and (4) the magnetic field intensity at the line formation region Bline, contained in the quantity b1 in their parameterization (see Gayley & Ignace 2010, Equations (30) and (32)). Additionally, in order to account for lines of different widths, one more parameter is used to determine the crossover wavelengths (|x| = 1 in their parameterization) on each wing of the line profile. Along with the reversal existing at line center, this is where the polarization reversals occur in the Stokes V model. It is typically the wavelength that resonates at the line formation region, which appears around the half-width at half-maximum for rounded profiles, expressed as λx = 1 in what follows.
Our approach was to simultaneously fit all Stokes V profiles recorded during one epoch of observation (within a chosen spectral line). Thus, for the 2005 data, three nights were simultaneously modeled while four nights were used for both fitting processes of the 2009 and 2010 data. At first, a χ2 minimization was used to compare the observed Stokes V signatures to a grid of computed, theoretical profiles so the best set of parameters can be determined. The minimization was done in two successive steps: find the value of λx = 1 first, and then determine the magnetic geometry [Bline, i0, β, ϕ0]. We proceeded this way since the shape of Stokes V model profiles is not a variable; only its amplitude with time is. In this framework, [Bline, i0, β, ϕ0] are free parameters, but constants for a given model. Because the geometry of the magnetic field does not influence where |x| = 1 occurs on the emission-line profile, we chose to dissociate both while performing the χ2 minimization. This means that a first grid (five parameters) is generated to determine λx = 1 and a second grid (four parameters) to find the best set of [B, i0, β, ϕ0] with the previously found value of λx = 1 fixed.
To begin with, wavelengths λ were transformed into scaled wavelengths x (as used by Gayley & Ignace 2010) according to Equation (9):

where λo is the central wavelength of the line, ΔλHWHM = λHWHM − λo is the half-width at half-maximum of the line profile, and κ is a constant scale factor. When κ = 1, this definition of x assumes that λx = 1 = λHWHM. To find the correct position of |x| = 1, we allow κ to vary by no more than ±20%, i.e., the scale factor was sampled from 0.8 to 1.2 at every 0.02. Ideally, eye inspection of the observed profiles would clearly indicate where |x| = 1, and finding its position on the line profile would be straightforward. Since the recorded data do not yield such a clear visual picture, and because the binning process smooths out the polarization reversal regions, the κ parameter sampling is sufficient to obtain reasonable precision. Furthermore, Gayley & Ignace (2010) mention that the sharp wing spike occurring at |x| = 1 is an artifact of their treatment, and a smoother transition (which would involve some cancellation near the discontinuity) is to be expected for emission lines with rounded top. The following specifies how the first grid samples the other parameters: 0 ⩽ Bline ⩽ 3000 G at every 20 G; 0° ⩽ i0 ⩽ 180° at each 10° (idem for β); and 0 ⩽ ϕ0 ⩽ 1 at each 0.1 phase. Once the best κ value is found (according to χ2 statistics), the second grid of computed profiles is generated sampling each of the remaining four parameters within the same range of values but using a smaller step size: ΔBline = 10 G, Δi0 = Δβ = 10°, and Δϕ0 = 0.025. In the end, the parameters that provided the lowest reduced-χ2 were chosen to represent the best-fitting model. This χ2 minimization procedure was applied to the four strongest emission lines present in the spectrum of EZ CMa: He ii λλ4686, 5411, 6560, and N iv λ7111.
Figure 7 shows an example (for the 2010 data set) of the χ2 landscapes computed from the observed Stokes V profiles of the He ii λ4686 emission line. Each landscape is a two-dimensional slice of the grid (with the other three parameters being fixed at their best-fit values), showing the reduced-χ2 values with the minimum indicated by a white star. Additionally, the 1σ, 2σ, and 3σ uncertainty contours are superimposed on the landscapes, and the former allows us to estimate the uncertainty of the best-fitting model parameters. These are summarized in Table 8.
Figure 7. Parameter estimation of the split monopole model from a χ2 goodness-of-fit test. All panels show two-dimensional χ2 landscapes of the model grid with the white star indicating the position of the minimum reduced-χ2, representing the best parameter estimates. Also, the 1σ, 2σ, and 3σ uncertainty contours are superimposed. Here the χ2 test is applied to the Stokes V profile within the He ii λ4686 emission line (2010 data set).
Download figure:
Standard image High-resolution imageTable 8. Best Parameter Estimates of the Split Monopole Model
Epoch | Line | Bline | i0 | β | ϕ0 | κ | χ2ν (Fit/Null) |
---|---|---|---|---|---|---|---|
(1) | (2) | (3) | (4) | (5) | (6) | (7) | (8) |
2005 | He ii λ4686 | 100−92+224 | 55−55+40 | 115−31+65 | 0.800−0.270+0.200 | 0.96−0.16+0.24 | 1.02/1.03 |
125−40+55 | 65−65+31 | ||||||
He ii λ5411 | 170−91+1399 | 45−42+62 | 80−77+27 | 0.300−0.255+0.180 | 1.14−0.07+0.06 | 1.47/1.46 | |
135−62+42 | 100−27+77 | ||||||
He ii λ6560 | 240−228+375 | 55−47+55 | 70−62+40 | 0.925−0.065+0.075 | 0.90−0.06+0.12 | 1.07/1.09 | |
125−55+47 | 110−40+62 | ||||||
N iv λ7111 | 140−120+620 | 60−41+41 | 105−26+55 | 0.575−0.075+0.215 | 1.20−0.04+0.00 | 1.01/1.02 | |
120−41+41 | 75−55+26 | ||||||
2009 | He ii λ4686 | 80−71+234 | 50−50+58 | 65−65+43 | 0.275−0.140+0.203 | 1.14−0.09+0.06 | 1.06/1.12 |
130−58+50 | 115−43+65 | ||||||
He ii λ5411 | 120−82+262 | 70−56+37 | 70−56+37 | 0.500−0.050+0.210 | 0.80−0.00+0.11 | 1.09/1.09 | |
110−37+56 | 110−37+56 | ||||||
He ii λ6560 | 70−38+123 | 60−37+27 | 60−37+27 | 0.125−0.101+0.097 | 1.08−0.08+0.04 | 1.11/1.11 | |
120−27+37 | 120−27+37 | ||||||
N iv λ7111 | 70−63+315 | 65−64+50 | 70−69+45 | 0.975−0.215+0.025 | 0.94−0.09+0.26 | 1.32/1.32 | |
115−50+64 | 110−45+69 | ||||||
2010 | He ii λ4686 | 70−44+289 | 145−49+35 | 35−35+49 | 0.400−0.200+0.150 | 0.94−0.11+0.05 | 1.00/1.02 |
He ii λ5411 | 80−55+283 | 65−41+40 | 70−46+35 | 0.275−0.105+0.120 | 1.10−0.04+0.08 | 1.04/1.04 | |
110−35+46 | 115−40+35 | ||||||
He ii λ6560 | 40−40+82 | 45−45+71 | 50−50+66 | 0.300−0.300+0.700 | 1.06−0.26+0.14 | 1.07/1.04 | |
135−71+45 | 130−66+50 | ||||||
N iv λ7111 | 80−47+377 | 25−25+34 | 25−25+34 | 0.775−0.185+0.225 | 1.16−0.02+0.04 | 1.22/1.24 | |
155−34+25 | 155−34+25 |
Notes. Columns 3–7 list each parameter value with its 1σ uncertainty corresponding to the extent of the contour level. Bline is expressed in gauss, while i0 and β are in degrees. The reduced-χ2 value of the best-fitting model and null profile are presented in column 8.
Download table as: ASCIITypeset image
The i0–β landscape shows the presence of two minimum values of χ2ν. This is a direct consequence of modulating the heartbeat Stokes V profile according to the phase of observation. To achieve this, Equations (30) and (32) of Gayley & Ignace (2010) need to be corrected by a phase-dependent scale factor, F(ϕ) = 1 − sin (iB), where iB represents the effective viewing inclination of the field axis at any moment. It is defined as cos (iB) = cos (i0)cos (β) + sin (i0)sin (β)cos (ϕ) (Ignace et al. 2011). According to this definition and because i0 and β are allowed to vary from 0° to 180°, there exist two pairs of i0 − β values that generate the same modulating factor F(ϕ), and this degeneracy is reflected by the presence of two minima in Figure 7 (i0–β landscape). Furthermore, due to the trigonometric properties of the sine and cosine functions, four pairs of i0 − β values can also yield the same minimum in a χ2ν landscape. When reading Table 8, it is important to remember that values of i0 and β can be swapped; the order in which they are presented does not reflect any preferences. This method of determining the best estimates for the split monopole model parameters gives poor constraints on the angles i0 and β. It would be arbitrary to invoke any trends or preferred values for both parameters given the degeneracy of the phase-dependent modulation applied to the model. The magnetic field intensity at the line formation region appears to decrease from the first epoch of observation to the last. Assuming that the emission lines considered here are formed roughly at the same distance from the star and under the same conditions, the average of Bline is ∼163 G in 2005, ∼85 G in 2009, and ∼68 G in 2010. The same decreasing trend is noticeable, in general, when each spectral line is considered individually. However, Bline estimates suffer from large uncertainties, and this epoch-dependent variation cannot be confirmed. It is important to note that the quality of ESPaDOnS observations has increased over time, which is reflected by the generally decreasing error bars on Bline at each epoch of observation. This could also explain the observed decreasing trend as better data were recorded in 2010 than in 2005. At best, this analysis allows us to propose an upper-limit value for the magnetic field strength in the wind of EZ CMa of Bline ∼ 100. Conversely, the reduced-χ2 values are generally close to unity while the field strength approaches zero, within its lower uncertainty limit. This could also mean that the non-magnetic hypothesis offers a reasonable fit to the data.
Still within the framework of the split monopole model, we performed a Bayesian analysis of the spectropolarimetric observations following the work of Petit & Wade (2012). This analysis describes the relative likelihood of the split monopole model by generating a multidimensional posterior probability density that covers the parameter space of the model. Since only ϕ is allowed to change between different observations, rotation-independent magnetic configurations [Bline, i0, β] can be computed in order to determine which configuration yields good posterior probabilities for all observed Stokes V profiles. The set of parameters that provides the maximum likelihood model is then considered the most probable magnetic configuration fitting the data. In this Bayesian statistics approach, we do not constrain the phases (ϕ being treated as a nuisance parameter). The inclination angle or the obliquity angle can vary from 0° to 180°, while the magnetic intensity has an upper bound at 3 kG. The rotational phase and obliquity both have simple flat priors, and the inclination angle prior is described by a random orientation probability distribution of the form p(i0) = 0.5sin (i0). Since the magnetic field strength is a parameter that can vary over several decades, we need to set an equal probability per decade while avoiding the singularity at B = 0. Therefore, we used a modified Jeffreys prior (Gregory 2005; Petit & Wade 2012) with a cut at B = 20 G, which corresponds to twice the grid step. The computed probability distribution functions (PDFs) marginalized for i0, β, and Bline are presented, from top to bottom, in Figure 8 for our 2010 observations. The PDFs have been normalized by their maximum values to facilitate the display. Also, credible regions corresponding to 68.3%, 95.4%, and 99.7% of the total posterior probability distribution (analogous to the 1σ, 2σ, and 3σ contours of a Gaussian-like distribution) appear (superposed) in shades of green, from dark to pale colors, respectively. To express the best estimates of model parameters, we present the maximum of the joint posterior probability density (MAP) and the modes of the marginalized PDF for each parameter. These are computed for the four strongest lines, for all epochs of observations, and are summarized in Table 9. According to Petit & Wade (2012), MAP and mode values are not always equal, especially if the probability distribution is complex. The MAP values generally produce the best fit to the data but do not necessarily reflect the bulk of each PDF. On the other hand, the mode of each parameter represents adequately the bulk of the probability but does not necessarily give a good fit to the observations. The Bayesian analysis was performed assuming κ values found previously when applying the χ2 goodness-of-fit test. We nonetheless verified that the same κ values were obtained through the Bayesian framework by making no assumptions on the scaling parameter and running the analysis. Since this verification yielded the same results, we then focused on the parameters describing the large-scale magnetic split monopole.
Figure 8. Parameter estimation of the split monopole model from a Bayesian analysis. Posterior PDFs marginalized for the rotational axis inclination, magnetic obliquity angle, and the magnetic field strength at the line formation zone are presented from top to bottom, respectively. The 68.3%, 95.4%, and 99.7% credible regions appear (superposed) in shades of green, from dark to pale colors, respectively. Here the Bayesian analysis is applied to the He ii λ4686 emission line Stokes V profiles recorded in 2010.
Download figure:
Standard image High-resolution imageTable 9. Results of the Bayesian Analysis Applied to Stokes V Observations
Epoch | Line | MAP Estimates | Mode Estimates | χ2ν | ||||
---|---|---|---|---|---|---|---|---|
Bline | i0 | β | Bline | i0 | β | MAP/Mode/Null | ||
(1) | (2) | (3) | (4) | (5) | (6) | (7) | (8) | (9) |
2005 | He ii λ4686 | 0 | 90 | 0 | 0+80 | 85−35+45 | 10−10+49 | 1.04/1.04/1.03 |
95−45+35 | 170−49+10 | |||||||
He ii λ5411 | 0 | 90 | 90 | 0+80 | 90−37+37 | 90−45+45 | 1.49/1.49/1.46 | |
He ii λ6560 | 40 | 110 | 70 | 0+115 | 85−31+41 | 85−51+61 | 1.07/1.11/1.09 | |
95−41+31 | 95−61+51 | |||||||
N iv λ7111 | 0 | 90 | 90 | 0+125 | 90−35+35 | 90−55+55 | 1.04/1.04/1.02 | |
Combined H ii lines | 0 | 90 | 0 | 0+40 | 90−39+39 | 0+42 | 1.15/1.15/1.15 | |
180 | 180−42 | |||||||
2009 | He ii λ4686 | 0 | 90 | 90 | 0+65 | 90−38+38 | 90−26+26 | 1.11/1.14/1.12 |
He ii λ5411 | 50 | 75 | 70 | 0+120 | 90−35+35 | 90−53+53 | 1.10/1.10/1.09 | |
He ii λ6560 | 70 | 65 | 60 | 80−80+135 | 75−22+52 | 65−31+18 | 1.06/1.07/1.11 | |
105−52+22 | 115−18+31 | |||||||
N iv λ7111 | 0 | 90 | 90 | 0+75 | 90−38+38 | 90−28+28 | 1.34/1.34/1.32 | |
Combined H ii lines | 30 | 125 | 145 | 20−20+45 | 85−35+45 | 10−10+49 | 1.10/1.11/1.10 | |
95−45+35 | 170−49+10 | |||||||
2010 | He ii λ4686 | 60 | 145 | 20 | 60−60+110 | 70−32+14 | 10−10+43 | 1.00/1.03/1.02 |
110−14+32 | 170−43+10 | |||||||
He ii λ5411 | 80 | 110 | 115 | 80−80+125 | 85−29+39 | 80−38+58 | 0.99/1.02/1.04 | |
95−39+29 | 100−58+38 | |||||||
He ii λ6560 | 0 | 90 | 0 | 0+60 | 90−40+40 | 0−0+45 | 1.07/1.07/1.04 | |
180−45+0 | ||||||||
N iv λ7111 | 90 | 145 | 175 | 90−50+165 | 55−27+21 | 5−5+35 | 1.24/1.40/1.30 | |
125−21+27 | 175−35+5 | |||||||
Combined H ii lines | 30 | 90 | 75 | 30−30+55 | 90−33+33 | 90−51+51 | 1.02/1.02/1.03 |
Notes. Columns 3–5 present the best estimates for the rotation-independent parameters based on MAP. The mode values along with their respective uncertainties (based on the 68.3% credible region) appear in columns 6, 7, and 8. Bline is expressed in G, and angles i0 and β in degrees. The last column gives three values of the reduced-χ2 computed for the split monopole model using MAP estimates, same model using mode estimates, and null (non-magnetic) profile, respectively. For all epochs of observations, the Bayesian analysis is applied to the four strongest emission-line profiles (individually), as well as to all He ii lines of that subset (simultaneously).
Download table as: ASCIITypeset image
Another useful application of Bayesian statistics resides in testing the plausibility of one model versus another by computing the so-called odds ratio, . It provides a more sensitive diagnostic of the presence of a weak magnetic signature embedded in noise than the detection probability technique or the longitudinal field method (Petit & Wade 2012). In this case, we can quantitatively evaluate the plausibility of the magnetic split monopole hypothesis, M1, by calculating its odds ratio with the null model, M0, representing the non-magnetic hypothesis (Stokes V = 0). The evidence supporting model M1 is considered very strong when
is >102 (100:1), strong when >101.5 (30:1), moderate when >101 (10:1), and weak when >100.5 (3:1) (Jeffreys 1998). The odds ratios computed from Stokes V profiles are also summarized in Table 10. As a sanity check of these values, the same analysis was applied to the check spectra N1 and N2.
Table 10. Odds Ratio Computed for Stokes V, N1, and N2 Observations
Epoch | Line | Log(![]() |
||
---|---|---|---|---|
Stokes V | Null N1 | Null N2 | ||
(1) | (2) | (3) | (4) | (5) |
2005 | He ii λ4686 | −0.30 | −0.33 | −0.46 |
He ii λ5411 | −0.31 | −0.34 | −0.51 | |
He ii λ6560 | −0.16 | −0.47 | −0.49 | |
N iv λ7111 | −0.18 | −0.46 | −0.48 | |
Combined H ii lines | −0.50 | −0.47 | −0.64 | |
2009 | He ii λ4686 | −0.35 | −0.50 | −0.17 |
He ii λ5411 | −0.45 | −0.40 | −0.49 | |
He ii λ6560 | 0.62 | −0.54 | −0.43 | |
N iv λ7111 | −0.26 | −0.52 | −0.52 | |
Combined H ii lines | −0.14 | −0.57 | −0.49 | |
2010 | He ii λ4686 | 0.66 | −0.53 | −0.50 |
He ii λ5411 | 0.23 | −0.38 | −0.14 | |
He ii λ6560 | −0.35 | −0.50 | −0.35 | |
N iv λ7111 | 1.95 | −0.32 | −0.13 | |
Combined H ii lines | 0.00 | −0.68 | −0.22 |
Notes. For all epochs of observations, the odds ratios are computed for the four strongest emission lines (individually) and for all He ii lines of that subset (simultaneously). The values are expressed in a base-10 logarithm form, i.e., log().
Download table as: ASCIITypeset image
The Bayesian analysis of individual emission lines in the spectrum of EZ CMa results, in most applications, in a non-detection. In fact, Tables 9 and 10 illustrate that field intensities of Bline = 0 are usually preferred to describe the observations, which is reflected by the associated odds ratio favoring the non-magnetic hypothesis. The magnetic split monopole hypothesis is preferred on four occasions, three of them being part of the 2010 observing run. This could be explained by the upgrades done on ESPaDOnS through the years, increasing the spectropolarimeter efficiency and precision. On the other hand, it could also reflect an intrinsic change in EZ CMa's magnetic properties. Although less likely, this hypothesis is still a possibility given the variable nature of the W-R star with epoch (Robert et al. 1992). Taking into account all nights simultaneously, the odds ratio for the He ii λ6560 emission line Stokes V profiles observed in 2009 is ∼4: 1 in favor of the magnetic hypothesis, while the N1 and N2 profiles are both ∼3: 1 in favor of the null, non-magnetic hypothesis, as expected. In 2010, the odds ratio is ∼5: 1, ∼2: 1, and ∼89: 1 in favor of the magnetic split monopole for Stokes V profiles for the He ii λ4686, He ii λ5411, and N iv λ7111 emission lines, respectively. Meanwhile, all odds ratios computed from their respective N1 and N2 profiles favor the non-magnetic scenario, as expected. It is interesting to note that Bayesian model comparison, through the calculation of the odds ratio, automatically disadvantages more complicated models. It has a built-in Occam's razor implying that simpler explanations are to be preferred, unless there is sufficient evidence in favor of more complicated explanations (Gregory 2005). The theorem of Bayes both quantifies such evidence and determines how much additional evidence is sufficient by assigning a large probability to a more complicated model only if the complexity of the data justifies the additional complication of the model. Since the expected Stokes V amplitudes expected for a magnetized stellar wind are at the noise level of our ESPaDOnS observations, it is not surprising to obtain an odds ratio in favor of a non-magnetic scenario. Only observations showing larger (in amplitude) and more clearly organized Stokes V signatures are assessed additional evidence via the calculation of the split monopole model global likelihood. It is thus interesting to note the existence (in a few cases) of the odds ratio favoring the magnetic hypothesis even if the evidence is, in general, weak.
With this method, when a magnetic field is detected, only limited information can be obtained for angles i0 and β. The magnetic geometry is recovered by the mode values of the marginalized PDFs (most probable inclination and obliquity), but the credible regions are too extended to put strong constraints on these parameters. On average, the field strength (when detected) is 〈Bline〉 = 78−31+88, which is significant at approximately 2.5σ. The latter assumes that the emission lines considered are formed roughly under the same conditions, in the wind of EZ CMa. Since it was reported (Hillier 1987; Schulte-Ladbeck et al. 1995; Herald et al. 2000) that EZ CMa exhibits ionization stratification in its stellar wind, averaging the field strength from different emission lines is a simplistic way of quantifying the magnetic intensity. Nonetheless, even if different spectral lines form at different distances for the star's surface, their formation zones are quite extended and often overlapping. Consequently, 〈Bline〉 should be considered as an order-of-magnitude indication of the field strength. Hoping that the combination of more observations (from the same epoch) would yield better constraints on the split monopole model parameters, we combined data from the He ii λ4686, He ii λ5411, and He ii λ6560 emission lines and performed the Bayesian analysis. These He ii spectral lines (same ionic species) are roughly formed at the same distance from the stellar core, 8–10 Rcore according to Hillier (1987), and should therefore be generated in a similar magnetized plasma. In 2009 and 2010, the most probable values for Bline are 20−20+45 and 30−30+55, respectively, while for the 2005 observing run, Bline = 0+40 is the most probable estimate of the field strength (see Table 9). The corresponding odds ratios (see Table 10) are all in favor of the non-magnetic hypothesis, which is not surprising given the weak estimates obtained for the field strength.
6. DISCUSSION AND CONCLUSIONS
In this study, we report new magnetic measurements of the W-R star EZ CMa based on spectropolarimetric observations obtained with ESPaDOnS at CFHT. Most of the observations and their analysis were undertaken within the context of the Magnetism in Massive Stars (MiMeS) Project. High-resolution circular polarization measurements allowed us to investigate individual emission lines for Zeeman-splitting patterns in their Stokes V profiles. Assuming the split monopole model of Gayley & Ignace (2010), we propose an upper limit for the magnetic field strength in the wind of EZ CMa of Bwind ∼ 100 G. It has been previously demonstrated that EZ CMa, like probably all W-R stars, shows the presence of ionization stratification in its stellar wind (Hillier 1987; Schulte-Ladbeck et al. 1995; Herald et al. 2000). By fitting a Gaussian profile to the emission lines considered in our analysis, we obtain, on average, half-width at half-maximum velocities of 1125, 956, 1056, and 1192 km s−1 for He ii λ4686, He ii λ5411, He ii λ6560, and N iv λ7111, respectively. Assuming v∞ = 1700 km s−1 (Hamann et al. 2006) and a β-velocity law, it is then possible to transform these velocities into radial distances from the stellar core.
Typically, the velocity-law index β is set to unity in models simulating a W-R stellar wind (Sander et al. 2012; Hamann et al. 2006). However, while β = 1 adequately describes the strong acceleration in the inner part of the wind, the outer part tends toward higher β values (Gräfener & Hamann 2005; Lépine & Moffat 1999; Schmutz 1997). Adopting a single value of β would thus approximate the entire wind structure, whereas the double β-velocity law proposed by Hillier & Miller (1999) could offer a better match to the overall structure. While this remains to be clarified in the case of EZ CMa, Hillier (1987) and Schulte-Ladbeck et al. (1995) have demonstrated that the emission lines considered in this study form farther out in the wind rather than close to the star's surface. Furthermore, Schmutz (1997) showed that the outer velocity structure of EZ CMa can be approximated using β ⩾ 3. In any case, we present in Table 11 the different line formation zone radii obtained by adopting both β = 1 and β = 3. In their model of a magnetized stellar wind, Gayley & Ignace (2010) parameterized the wind velocity structure as v = r, which is better represented by a β-velocity law using a high power index. This parameterization, combined with the work of Gräfener & Hamann (2005), Lépine & Moffat (1999), and Schmutz (1997), tends to suggest that a high value of the index β should be preferred. Consequently, the discussion below assumes a value of β = 3.
Table 11. Emission-line Formation Zone Properties of EZ CMa
Emission Line | ![]() |
Rline (R⋆) | |
---|---|---|---|
(km s−1) | β = 1 | β = 3 | |
He ii λ4686 | 1125 ± 31 | 3.0 ± 0.2 | 7.7 ± 0.5 |
He ii λ5411 | 956 ± 23 | 2.3 ± 0.1 | 5.7 ± 0.2 |
He ii λ6560 | 1056 ± 21 | 2.6 ± 0.1 | 6.8 ± 0.3 |
N iv λ7111 | 1192 ± 28 | 3.3 ± 0.2 | 8.9 ± 0.6 |
Notes. The radial distances (Rline) are computed (according to the β-velocity law) for values of β = 1 and β = 3. We adopt v∞ = 1700 km s−1 (Hamann et al. 2006) and assume that a given line formation zone is characterized by a wind velocity approximately equal to the averaged half-width at half-maximum velocity () of the corresponding emission line. R⋆ represents the stellar core radius.
Download table as: ASCIITypeset image
Since the intensity of a split monopole magnetic field decreases as 1/r2, we propose a conservative upper limit for the surface magnetic field strength of B⋆ ∼ 5.4 kG. For completeness, we also derived B⋆ (as well as other parameters introduced below) using β = 1, which appears in Table 12. Using this upper limit for the surface magnetic field strength and the stellar parameters taken from Hamann et al. (2006), we find that the wind confinement parameter (ud-Doula & Owocki 2002) is η⋆ ∼ 1.8. The radial variation of η(r) is modeled in terms of a magnetic power-law index q (=3 for a dipole7) and the velocity-law index β. This allows us to calculate the Alfvén radius at which the magnetic and wind energy densities are equal, η(RA) ≡ 1 (see ud-Doula et al. 2006, Equation (1)). According to ud-Doula & Owocki (2002), this value provides a reasonable estimate for the maximum radius at which magnetic loops remain closed in a wind outflow, before being eventually opened into a nearly radial configuration at larger radii. Based on our upper limit for the surface magnetic field of EZ CMa, the Alfvén radius extends to RA ∼ 2.0 R⋆ if we adopt β = 3. Within this distance, the flow is channeled toward the magnetic equator causing shock collisions. MHD simulations by ud-Doula & Owocki (2002) show that, for a moderate magnetic confinement parameter η⋆ ∼ 1, the deflection toward the magnetic equator becomes more pronounced than at low values of η⋆, with a correspondingly stronger equatorial change in density and radial flow speed. Even for the weak magnetic case (η⋆ < 1), there is still a noticeable deflection of the outflow leading to an increased density and a decreased radial flow speed in the equatorial region. These authors also stipulate that the shock collisions occurring within closed loops are potentially strong enough to produce hard X-rays while the stagnated material on top of closed field loops is then pulled by gravity inward, back onto the star.
Table 12. Magnetic and Line-emission Zone Parameters of EZ CMa
Parameters | β-velocity Law Structure | |
---|---|---|
β = 1 | β = 3 | |
![]() |
∼2.8 | ∼7.3 |
B⋆ (kG) | ∼0.8 | ∼5.4 |
η⋆ | ∼0.04 | ∼1.8 |
RA(R⋆) | ∼1.0 | ∼2.0 |
Notes. Parameters , B⋆, η⋆, and RA are computed for the velocity-law indices β = 1 and β = 3. The radii
and RA correspond to the average of the line-formation zone distances from the stellar surface (typically where Bwind ∼ 100 G) and to the Alfvén radius, respectively. B⋆ is the magnetic field strength at the stellar core, and η⋆ is the wind confinement parameter as described by ud-Doula & Owocki (2002).
Download table as: ASCIITypeset image
Using the stellar parameters of EZ CMa modeled by Hamann et al. (2006) and an equatorial velocity (veq ∼ 40 km s−1) computed by St-Louis et al. (2009) assuming that the 3.766 day period is the rotation period, we can calculate the rotation parameter W = veq/vcrit ≃ 0.03 and the Kepler (corotation) radius RKep = W−2/3R⋆ ≃ 9.5R⋆. For any material trapped on magnetic loops inside the Kepler radius, the outward centrifugal support is less than the inward pull of gravity (ud-Doula et al. 2008). In other words, when R⋆ < RA < RKep, much of the material (compressed into clumps) is too dense to be line driven and falls back toward the star. Consequently, the transient suspension of trapped material in closed loops establishes a dynamical magnetosphere (DM) as opposed to when RA > RKep > R⋆, in which case the rotationally supported, magnetically confined material accumulates to form a centrifugal magnetosphere (CM; Petit et al. 2013). EZ CMa falls in the former domain, and if there is indeed a weak, split monopolar magnetic field, the effects of rotation are most likely limited to some modest, transient enhancement in equatorial density and overall mass-loss rate, relative to non-rotating cases. Beyond the Alfvén radius and into the more open field flow, the channeling could also be sufficient to deflect the flow toward the magnetic equator and possibly produce oblique shocks. If strong enough, these shocks could create moderate density enhancements and potentially generate X-rays. These MHD simulations were not specifically intended to obtain a portrait of the magnetized stellar wind of a W-R star, but combined with the upper limits found for EZ CMa, they provide interesting groundwork for interpreting the intrinsic variability of the star, which could be the result of surface magnetic fields perturbing its inner stellar winds.
Due to the intrinsic instability of the line-driving mechanism for radiative stellar winds (Feldmeier et al. 1997; Runacres & Owocki 2002; Dessart & Owocki 2003), massive stars are generally luminous in X-rays, with a canonical value for early OB stars of LX > 10−7.2L⋆ (Berghoefer et al. 1997; Nazé et al. 2011; Gagné et al. 2011). Cassinelli & Swank (1983) proposed two sources of X-ray emission: X-rays that arise from fragmented shocks in the wind and X-rays from very hot, probably magnetically confined loops, near the base of the wind. The latter scenario was studied by Babel & Montmerle (1997). Based on a magnetically confined wind shock (MCWS) model, they predicted that a collision between the wind components from the two hemispheres in the closed magnetosphere leads to strong shocks and characteristic X-ray emission. More precisely, several predictions can be directly compared with observations: (1) the hottest plasma should be located at a few stellar radii from the stellar surface where the wind streams collide; (2) the X-ray emission lines should be rather narrow, because the hot plasma is nearly stationary; (3) magnetic stars should be more X-ray luminous than their non-magnetic counterparts of similar spectral type; (4) the X-ray spectrum of magnetic stars should be harder than that of non-magnetic stars, with the bulk of the hot plasma at the hottest temperature; and (5) the X-ray emission should be modulated periodically as a consequence of the occultation of the hot plasma by a cool torus of matter, or by the opaque stellar core. When magnetic O stars are considered, it appears that only one star, θ1 Orionis C (Donati et al. 2006), displays properties that are fully compatible with the predictions of the MCWS model. The X-ray observations of other magnetic O stars led to perplexing results: while some comply to the predictions of the magnetically confined wind model, others do not. Overall, the X-ray properties of O-type stars are diverse. Strong, hard, and variable X-ray emission may be a sufficient attribute of magnetic massive stars, but it is not a necessary one (Oskinova et al. 2011a, 2011b). Consequently, it seems at first glance that luminous, hard, and variable X-ray emission could be a proxy for magnetism in massive stars even if these properties are not always present in those stars. Petit et al. (2013) compiled a comprehensive list of O stars for which magnetic fields have been convincingly detected via the Zeeman effect and classified their magnetospheres. As all these stars show some level of overluminosity (log(LX/L⋆) > −6.7), they proposed that a potential explanation for the X-ray emission enhancement in CMs is the centrifugal acceleration for fast rotators, which contributes by propelling the confined material up the magnetic loop, leading to stronger shocks than what could be achieved by radiative acceleration alone like in a DM. Studying the X-ray emission could thus provide a different perspective on the structure and dynamics of magnetospheres and the shock physics occurring in both DMs and CMs. While our understanding of the connection between X-rays and magnetism in OB stars is progressing, the X-ray emission from W-R stars, the evolved descendants of O-type stars, remains enigmatic. Because their dense winds are much more opaque to X-rays than those of O stars, one may thus naively expect W-R stars to be less X-ray bright than their progenitors. Also, because of their large mass-loss rates, even small values of η* require generally large magnetic field strengths. Yet some W-R stars are X-ray sources (Ignace et al. 2003; Skinner et al. 2010), while others remain undetected despite low upper limits on LX (Gosset et al. 2005).
Even if the global magnetic field of EZ CMa is likely too weak to generate a CM that would take the form of a thin, dense, slowly outflowing disk at the magnetic equator, it could be the seed of CIRs thought to be present in the wind of this star (e.g., St-Louis et al. 1995). Deflection of the wind outflow toward the magnetic equator past the Alfvén radius and the resulting shock collisions could also offer an explanation for the occasional flare-type variability (Matthews et al. 1992; Duijsens et al. 1996) and X-ray emission (Willis et al. 1994; Georgiev et al. 1999; Skinner et al. 2002). Furthermore, based on the work of ud-Doula & Owocki (2002), a B⋆ ∼ 5 kG could perturb the inner wind of EZ CMa and thus explain the dynamic changes in the large electron-density distribution and geometry proposed by Robert et al. (1992).
Additionally, if we assume that the magnetic field is effectively channeling the rotating wind, the above-mentioned results are consistent with the work of Harries et al. (1998, 1999). These authors argue that the observed continuum (linear) polarization behavior could result from a combination of wind flattening and corotating regions that would generate variable, large-scale structures in the stellar wind. They also state that the observed continuum polarizations of EZ CMa can be matched by models with equator:pole density ratios of 2–3, typical of W-R stars showing a reduction in linear polarization at emission-line wavelength. Furthermore, they propose that the most obvious mechanism for producing these large-scale structures is a global, corotating magnetic field. As we are only able to place an upper limit to the magnetic field rather far out in the wind, our result is compatible with such a flattening in the continuum-forming region of the wind, closer to the stellar core. As discussed above, if a DM exists within the Alfvén radius, it could produce a non-spherically symmetric electron density distribution that polarizes the continuum radiation but that is not detected in the line-forming region farther out in the wind. More observational data are necessarily needed to confirm whether or not EZ CMa harbors a magnetic field that could generate such an increase in density toward the magnetic equator (cf. Harries et al. 1998).
The magnetic field upper limit set in this paper needs to be considered with care. We do not infer the presence of a magnetic field in EZ CMa since the evidence supporting that statement is not strong enough. If a Zeeman signature is present in our recorded Stokes V observations, its detection is at the limit of ESPaDOnS' capabilities and is mostly embedded in noise. Indeed, the majority of our nightly Stokes V profiles (from different emission lines) seem to be compatible with a null, non-magnetic profile. Eye inspection of those profiles, at different levels of binning, does not show the typical s-wave pattern expected for a magnetized wind, and statistical tests applied to these signatures confirm a preference for the non-magnetic hypothesis. On the other hand, a few observed Stokes V profiles do show organized patterns that fit well the split monopole predictions. Although we cannot completely neglect the possibility of noise randomly taking the form of a magnetic signature, this scenario is unlikely since we put the raw data through a binning algorithm (to increase the S/N), which would average out random noise centered at zero. Numerical tests done on synthesized, null profiles afflicted by random noise similar to that present in the observations confirm this. If what appears to be an organized magnetic signature is not intrinsic to EZ CMa, it is probably due to spurious polarization coming from the polarimetric module of ESPaDOnS. However, the observations offering the strongest evidence for a magnetic detection were mostly recorded in 2010, i.e., after the spectropolarimeter had been successfully upgraded with better optical components and when the crosstalk level was below 1%.
Another, perhaps more natural, explanation for the lack of systematic significant detections could be directly related to EZ CMa's magnetic properties. If, during its 3.766 day rotation cycle, a favorable orientation (with respect to the observer) of the magnetic pole happens rarely, then the field component responsible for the longitudinal Zeeman effect would be too weak for any circular polarization to be detected. Assuming a weak magnetic field, the majority of the Stokes V profiles would not have large enough amplitudes to be distinguished from noise. On occasions though, the geometry of the field could allow the star to be observed at a more favorable orientation, therefore producing a detectable Stokes V signature. In this scenario, the simultaneous analysis of several consecutive nights of observations would lower the odds of a positive detection since most of the observed circular polarization profiles would favor the non-magnetic hypothesis. In addition, if EZ CMa was at a phase (when observations were made) of low magnetic activity, as proposed by Duijsens et al. (1996), then the odds of making positive detection are consequently lowered. The fact that we obtain some evidence, although weak, favoring the split monopole model over the non-magnetic hypothesis, when simultaneously analyzing Stokes V profiles recorded on consecutive nights, should be noted and should encourage the acquisition of new observations, preferably of even higher quality. At this point, a fundamental question arises. Could the detected circular polarization be due to some other process rather than being magnetic in origin? One conceivable possibility is multiple scattering even if the simple scattering process yields linear polarization. Circular polarization can actually be the result of multiple scattering of polarized radiation by non-spherical dust grains (Wolf et al. 2002). However, in the case of electron scattering, which completely dominates in hot-star winds, multiple scattering will always lead to linear polarization (see van de Hulst 1981), and in any case, there is no evidence for dust being generated in the wind of this W-R star.
The split monopolar configuration considered in this paper was chosen because of its generic character in a dense, radial stellar wind (Gayley & Ignace 2010). It represents a field with a high degree of symmetry, which is a natural assumption when rotation is not playing a central role. However, the wind of EZ CMa shows evidence of a departure from spherical symmetry (Schulte-Ladbeck 1994; St-Louis et al. 1995; Morel et al. 1998) in which rotation appears to be dynamically important. Taking this into account, Gayley & Ignace (2010) propose a possibility for magnetic detections in hot-star winds to result from aspherical pockets of intense emission from structures in the wind, rather than from smooth radial fields. Their analysis does not consider stellar occultation or emission from the stellar photosphere, but this is likely negligible since the emission lines studied in this paper are formed far out in the wind. Also, the split monopole model does not account for clumping, but the authors argue that it would likely not have a significant impact on the degree of polarization. It might even generate a local increase in B, depending on the dynamics of the clumping process, by increasing the field detectability in pockets where emission is especially strong. The absence of significant detections at all phases for a given emission line or for all spectral lines on a given night could perhaps be explained by the presence of clumps or dense pockets of material flowing into the emission-line formation zones and generating a local increase of the magnetic field. It would explain why magnetic signatures (on a given night) seem to appear within certain Stokes V line profiles while, most of the time, evidence supports the non-magnetic scenario. The chaotic and turbulent nature of W-R winds would make this process random, and thus a better understanding of the dynamics of line emission in such strong stellar winds would help predict the presence of circular polarization in observed data.
In summary, if EZ CMa has a large-scale magnetic field resembling a split monopole configuration far out in the wind (at v ∼ 1000 km s−1), we propose an upper limit of Bwind ∼ 100 G, which translates into B⋆ ∼ 5 kG at the stellar surface for velocity-law index β ∼ 3. New, better quality observations would certainly help to understand the true magnetic nature of EZ CMa and the mechanisms responsible for the circular polarization signatures observed occasionally in this study, if the latter are indeed intrinsic to the star. With higher S/N observations, the analysis of weak emission lines (e.g., higher members of the Pickering series such as He ii λ4100, He ii λ4200, and He ii λ4339) could help detect the presence of a magnetic field because these lines are formed closer to the stellar surface, where, if present, the field is much stronger. In the present study, the observed Stokes V profiles of weak lines, especially of higher ionization level, are unfortunately dominated by noise and do not show any magnetic signature. We believe that attaining a higher S/N in those emission lines would thus help set more stringent limits to the magnetic field of EZ CMa as we would be sampling its inner wind.
We thank Gregg Wade and Veronique Petit for very helpful conversations about state-of-the-art techniques to analyze polarization measurements. We acknowledge the crucial inputs of Ken Gayley and Rico Ignace regarding the understanding of their split monopole model and its phase-dependent modulation. We also thank Nadine Manset for her ongoing support in the reduction of ESPaDOnS data. Anthony F. J. Moffat and Nicole St-Louis are grateful for financial assistance from NSERC (Canada).
Facilities: CFHT (ESPaDOnS) - Canada-France-Hawaii Telescope
APPENDIX: MUELLER MATRIX CALCULUS FOR THE ESPaDOnS POLARIMETER
The purpose of this section is to predict, via Mueller matrix calculus, the consequence of angular errors on the positions of the Fresnel rhombs in the ESPaDOnS polarimeter. Deviations from the theoretically prescribed orientations can potentially produce crosstalk between Stokes parameters Q and U and Stokes V null profiles Stokes NV, 1 and NV,2.
After passing through the aperture hole (pierced mirror) at the f8 Cassegrain focus of CFHT, a beam of light goes through a triplet lens (to make it parallel) and the polarization optics (three Fresnel rhombs and one Wollaston prism). Specifically, the beam goes through the following successive optical elements: a λ/2-rhomb (rotatable), a λ/4-rhomb (fixed), a λ/2-rhomb (rotatable), and a Wollaston prism.
A.1. Mueller Matrices of Polarizing Elements
To calculate the outcome of an optical path, Mueller calculus requires the application of several matrices that must be organized in the appropriate order (Kliger et al. 1990; Clarke 2010). Below are the matrices representing the optical elements of the ESPaDOnS polarimeter: λ/2-Fresnel rhomb,
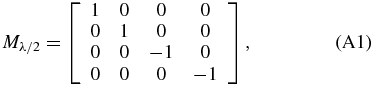
and λ/4-Fresnel rhomb,
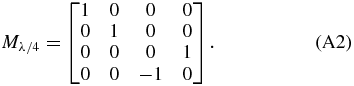
For a general linear polarizer, such as the Wollaston prism, with its optical axes rotated by an angle ω from the x-axis of a right-handed Cartesian frame, the Mueller matrix is given by
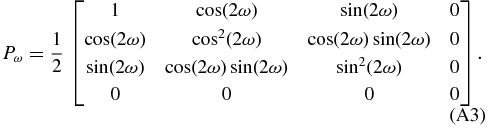
In the reference frame, the light beam travels along the z-axis and the x–y plane is perpendicular to direction of propagation. Angle ω is positive and is measured anti-clockwise, as seen looking against the direction of propagation.
ESPaDOnS is designed to record two orthogonal spectra, side by side, on its CCD detector. The first one corresponds to the ∥ beam of light (ω = 0°), and the second one to the ⊥ beam of light (ω = 90°). From Equation (A3), two Mueller matrices can be calculated for the two components emerging from a Wollaston prism: Wollaston ordinary beam (∥),
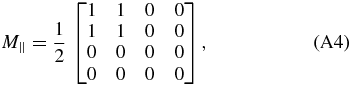
and Wollaston extraordinary beam (⊥),
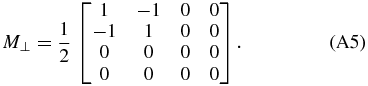
Equations (A4) and (A5) assume that the Wollaston prism exhibits perfect transmission. If transmission happens to be different from the ideal case, the matrices should be multiplied by coefficients (<1) to allow for this.
The individual matrices above represent the different elements of the polarization analyzing device of ESPaDOnS, but they do not account for their orientation, when applicable. For instance, a λ/2-Fresnel rhomb with a horizontal transmission axis has a different Mueller matrix from the same rhomb that is turned by some degrees. The Stokes vector representing the beam of light is expressed in a particular reference frame and, to determine the effect of any polarizing element, it is first necessary to set the description of the polarization to the frame corresponding to the principal axes of the device (Clarke 2010). Thus, the operation of a rotatable λ/2-Fresnel rhomb, say, may be represented by
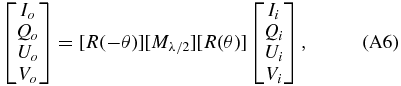
where the indices "o" and "i" stand for output and input, respectively. In the above example, the third 4 × 4 matrix rotates the output Stokes vector back to the original reference frame. Rotation of the direction of vibration of the linear polarization or of the major axis of the polarization ellipse can be expressed as
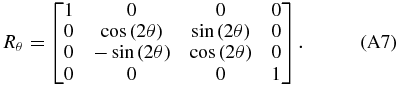
Positive θ is measured anti-clockwise from the x-axis toward the y-axis as seen looking against the z-direction (Clarke 2010).
In calculating the outcome of ESPaDOnS polarimeter optics, Mueller calculus requires the application of several matrices in combination that must be organized in the correct order. Following matrix algebra, we can calculate the four Stokes parameters outputted by the polarimeter with the equation below:
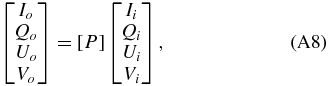
where

Here θ and ϕ are, respectively, the angle of the first and second λ/2-rhomb, and the [Wollaston] matrix stands for both the parallel and perpendicular components. So if one wants to calculate the ordinary component, [Wollaston] = [M∥], and for the extraordinary component of the output beam, [Wollaston] = [M⊥]. Equations (A10) and (A11) present the polarization matrix of ESPaDOnS for both components:
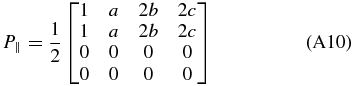
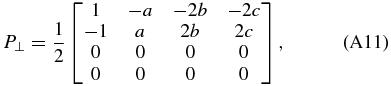
where



Following the double-ratio method described by Donati et al. (1997), four exposures using each a different combination of θ and ϕ are used to calculate Stokes parameters Q, U, and V. The general expressions for the parallel and perpendicular components of the intensity beam are described in Equations (A15) and (A16):
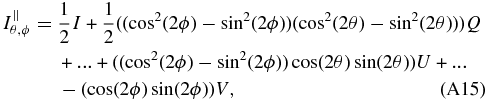
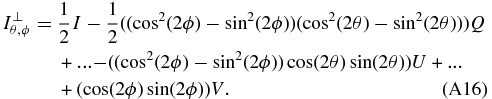
To measure Stokes V, the four different combinations8 of θ and ϕ are
- 1.θ = 0°, ϕ = 67
5,
- 2.θ = 90°, ϕ = 22
5,
- 3.θ = 45°, ϕ = 22
5,
- 4.θ = 135°, ϕ = 67
5.
In the end, two spectra(I∥θ, ϕ and I⊥θ, ϕ) are recorded side by side on the detector and are described as follows, depending on θ and ϕ, by the equations below:




With these equations, we can calculate the normalized Stokes V parameter along with its two null spectra with



where RV, , and
are defined by
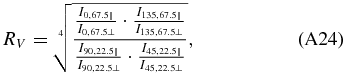
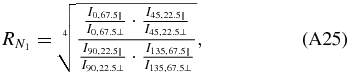
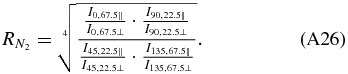
One can then explore how an angular error on θ and ϕ can affect the values of I∥θ, ϕ and I⊥θ, ϕ. Applying a third-order multivariate Taylor expansion on, say, I∥90, 22.5, we get
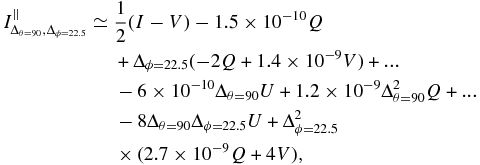
where Δτ = t = τ' − t are the angular errors on the Fresnel rhombs orientations, expressed in radians(τ' and t being the instrumental and theoretical orientations, respectively). For example, Δϕ = 67.5 = ϕ' − 675, expressed in radians. Neglecting terms with coefficients of order 10−9 or less, the full set of spectra recorded can be expressed as








From these, we can see that the calculation of RV, , and
does not yield the same results as in Equations (A24)–(A26). In particular,
and
do not equal one as they are design to be. Hence, the presence of errors on the orientations of the Fresnel rhombs could potentially yield crosstalk effects NV, 1 and NV, 2 from Stokes Q and U. For example, an error of ±1° on each position of the two Fresnel rhombs, in the first sub-exposure only, yields ±0.035Q and ±0.002U to enter the calculation of
and
. Even though the crosstalk model presented in Section 4 is oversimplified to adequately characterize the effects of crosstalk on observed NV, 1 and NV, 2 profiles, it still offers a good approximation. For example, using the values presented in Table 4, parameters αλ and βλ(on top of the He ii λ4686 emission line, λ ∼ 468.8 nm) are, for the 2010 data set, α = −0.013 and β = −0.012 within the NV, 1 line profile and α = −0.023 and β = −0.005 within the NV, 2 line profile. Similar values(order of magnitude) are obtained for the 2005 and 2009 observations.
Footnotes
- *
Based on observations obtained at the Canada–France–Hawaii Telescope (CFHT), which is operated by the National Research Council of Canada, the Institut National des Sciences de l'Univers of the Centre National de la Recherche Scientifique of France, and the University of Hawaii. This research used the facilities of the Canadian Astronomy Data Centre operated by the National Research Council of Canada with the support of the Canadian Space Agency.
- 1
- 2
- 3
- 4
- 5
- 6
- 7
The split monopole configuration assumed to be present in the line formation zones is the result of a dipolar magnetic field stretched out by the stellar wind.
- 8
See the PhD thesis of Veronique Petit(Petit 2011).