ABSTRACT
We investigate the propagation of 0.54–3.5 MeV ions accelerated at the termination shock of the solar wind. Data are from Voyager 2 and refer to a time interval about one year long, just before the Voyager 2 termination shock crossing at the end of 2007 August, at roughly 83.7 AU. A recently developed technique, which allows to unravel the transport properties from an analysis of the energetic particle time profiles, is used. The ion time profiles exhibit a power-law decay from a few days to 200 days before the shock front, so that transport is found to be superdiffusive, with a mean square deviation growing like 〈Δx2〉 ∝ tα, with α ∼ 1.3. This means that ion propagation in the heliosphere can be intermediate between normal diffusion and ballistic motion. The implication of ion superdiffusion on particle acceleration mechanisms at the termination shock is discussed, as well as some observational evidence coming from both Voyager 1 and Voyager 2, which questions diffusive shock acceleration.
Export citation and abstract BibTeX RIS
1. INTRODUCTION
After a travel more than 30 years long, and almost 100 AU away, the interplanetary probes Voyager 1 (V1) and Voyager 2 (V2) have reached the first boundary of the heliosphere, that is the solar wind termination shock (TS). These spacecraft are the first to explore the regions where the solar wind interacts with the interstellar medium, and the direct investigation has revealed a number of unexpected features, such as the discoveries that the thermal plasma flow is still supersonic after the shock transition (Richardson et al. 2008), that nonthermal ions have a major role in mediating the shock structure (Burlaga et al. 2008; Decker et al. 2008), and that the source of anomalous cosmic rays (ACRs) is not at the termination shock (Stone et al. 2005, 2008).
The transport of energetic particles and cosmic rays in turbulent environments, such as the solar wind, is usually described in terms of diffusive motion (e.g., Jokipii 1966; Bieber et al. 1994; Matthaeus et al. 2003). This diffusive motion is due to the pitch-angle scattering of particles interacting continuously with electric and magnetic turbulence present in the interplanetary medium. From a dynamical point of view, particles perform a random walk made of uncorrelated jumps (Markovian, one-step memory process). After a large number of jumps, the probability P(x, t) of finding a particle at position x at time t is a Gaussian. Indeed, assuming a finite second-order moment, the Central Limit Theorem predicts a Gaussian distribution and a mean square displacement growing linearly in time, i.e., 〈Δx2〉 ∝ t, in the limit of a large number of independent jumps (Boffetta et al. 2003). On the other hand, transport regimes different from normal, Gaussian diffusion have been observed in several physical systems (Metzler & Klafter 2000, 2004), and they are characterized by a mean square displacement growing like 〈Δx2〉 ∝ tα with α ≠ 1, encompassing both subdiffusion (α < 1) and superdiffusion (α>1), up to ballistic propagation (α = 2). These transport regimes, called anomalous, imply the breaking of the Central Limit Theorem; indeed, they are characterized by a non-Gaussian distribution P(x,t) and by the presence of long-range correlations (non-Markovian, multistep memory processes) (Klafter et al. 1987; Zaslavsky et al. 1989). The diffusion coefficient D is related to the particle velocity correlations by (Matthaeus et al. 2003)

If the velocity correlation decays exponentially, after a characteristic time the integral converges, D is finite, and a normal diffusion results. Conversely, if the velocity correlation has, for example, power-law tails, such that the integral diverges, a long-range memory process occurs, and the resulting transport is superdiffusive. Such anomalous transport regimes are associated with power-law tailed distributions of free path lengths, corresponding to a Lévy random walk rather than a Brownian motion (Klafter et al. 1987, 1996; Zaslavsky et al. 1989).
In astrophysical plasmas, a long-range correlated velocity parallel to the background magnetic field means that wave–particle interactions and pitch-angle diffusion are rather weak, to the point that the parallel mean free path can diverge. This possibility has been already discussed by Bieber et al. (1994).
While solar energetic protons often exhibit diffusive transport (Droge 2003; Ruffolo 2006), nearly ballistic, i.e., scatter-free, transport is routinely observed for solar energetic electrons (Lin 2005). Recent numerical simulations have shown that perpendicular subdiffusion and parallel superdiffusion are possible for energetic particle propagation in the presence of magnetic turbulence (Qin et al. 2002; Zimbardo 2005; Zimbardo et al. 2006; Shalchi & Kourakis 2007; Pommois et al. 2007). Understanding energetic particle propagation in space is important both for assessing the efficiency of cosmic-ray acceleration mechanisms (Bell 1978; Reames 1999; Lee 2005) and for the forecasting of solar energetic particle events which may be harmful for spacecraft orbiting Earth. Recently, Perri & Zimbardo (2007, 2008a) have shown, by analyzing the energetic particle profiles associated with corotating interaction regions (CIRs), and measured by the Ulysses spacecraft, that electron transport in the 40 keV to 300 keV energy channels is superdiffusive, 〈Δx2〉 ∝ tα with α ≃ 1.07–1.70. This indicates that propagation regimes intermediate between Gaussian diffusion and ballistic transport have to be taken into account for energetic particles in the solar wind. Here, we apply the technique developed by Perri & Zimbardo (2007, 2008a) to the energetic particles detected by the Low-Energy Charged Particle (LECP) instrument on board V2 upstream of the termination shock. We find experimental evidence that the transport of ions in the 540–3500 keV energy range is characterized by superdiffusion with α ≃ 1.29–1.32. The implications of ion superdiffusion on particle acceleration at the termination shock are also discussed.
2. DATA ANALYSIS
The shock transitions are characterized by an abrupt decrease in the solar wind velocity, an increase in the density and temperature, and by enhanced levels of turbulence and energetic particles. The method of analysis described in Perri & Zimbardo (2007, 2008a) aims to deduce the transport properties from an analysis of the energetic particle profiles measured by spacecraft, and is based on the computation of these profiles at the observer position by means of the propagator formalism (see also Webb et al. 2006). The propagator P(x − x', t − t') gives the probability of observing a particle at (x, t) if it was injected at (x', t'), and it has a Gaussian form for normal diffusion while it has power-law tails in the case of superdiffusion (Zumofen & Klafter 1993):

where b is a dimensional constant and 2 < μ < 3 for superdiffusion. By assuming a statistically homogeneous medium at some distance from the shock front, in order to have a level of magnetic turbulence approximately constant (Lee 2005), and a planar, one-dimensional shock moving at constant velocity with respect to the observer, so that the source of energetic particles can be described by a distribution function fsh(x', E, t') = λf0(E)δ(x' − Vsht'), where λ is a characteristic length and f0(E) represents the distribution function of particles of energy E emitted at the shock front, the particle time profile can be obtained as
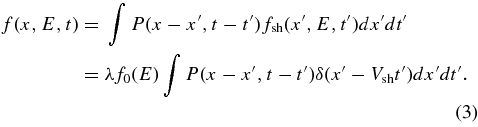
The integral in Equation (3) can be easily computed by assuming a constant speed Vsh for the moving shock. If the shock motion is time dependent the integral in Equation (3) is not straightforward and the particle time profiles cannot be computed explicitly. To fix the ideas, we assume that the shock comes from x < 0 for t < 0. For normal diffusion, i.e., for a Gaussian propagator, a simple exponential decay is obtained for the observer at x = 0, i.e., f(0, E, t) ∝ exp [ + V2sht/D] (for t < 0) (Fisk & Lee 1980; Lee 1983); conversely, for superdiffusion, i.e., for the propagator shown in Equation (2) (Perri & Zimbardo 2007, 2008a), a power-law decay at some distance from the shock front is derived. Thus,

When the exponent γ = μ − 2 assumes values 0 < γ < 1, a superdiffusive transport with α = 2 − γ is obtained (Perri & Zimbardo 2007, 2008a). Power-law propagators, having 1 < μ < 2, can also be found for ballistic transport (Zumofen & Klafter 1993; Perri & Zimbardo 2008b).
Multiple crossings of the termination shock occurred at 83.7 AU at the end of 2007 August (Richardson et al. 2008; Burlaga et al. 2008). The V2 data show that the shock normal magnetic field angle was between 743 and 82
8 (Richardson et al. 2008), corresponding to a supercritical quasi-perpendicular collisionless shock. Low-energy ion profiles have been studied during a period of time of about one year, from the middle of 2006 to 2007 August. In particular, the 1 day averages of ion fluxes for the four energy channels PL05 (215–540 keV), PL06 (540–990 keV), PL07 (990–2140 keV), and PL08 (2140–3500 keV) from the LECP experiment on board V2 have been analyzed (data from sd-www.jhuapl.edu/VOYAGER/vgr_data_files.html; P.I.: S. M. Krimigis). The V2 data are displayed in Figure 1: the upper panels show the solar wind velocity and the thermal speed (data from space.mit.edu/pub/plasma/vgr/v2/daily; P.I.: J. Richardson), which allow us to identify the shock crossing, which is denoted by a vertical dashed line. In the bottom panel, particle fluxes in the energy channels PL05, PL06, PL07, and PL08 have been plotted in log–lin axes. Lower energy channels have not been considered because they are near the background level until 32 days before the TS crossing (Decker et al. 2008). However, all energy channels are affected by a significant level of background due to high-energy cosmic rays. The background correction has been estimated as the lowest value of the ion flux in each energy channel for the analyzed period; this procedure gives results in excellent agreement with background-subtracted particle profiles published in Decker et al. (2008).
Figure 1. Upper panels show the time evolution of the magnitude of the proton bulk velocity and the proton thermal speed measured by the PLS instrument on board V2 (P.I.: J. Richardson); the lower panel displays the energetic particle data measured by the LECP instrument on board V2 (P.I.: S. M. Krimigis). The shock crossing time is indicated by a vertical dashed line.
Download figure:
Standard image High-resolution imageThe quasi-periodic variations of the ion flux in all of the energy channels, visible from the middle of 2006 to the middle of 2007, can possibly be ascribed to solar modulation. In particular, the 15-day-long marked decreases in the ion fluxes around the middle of 2007 are caused by the passage of a transient disturbance through the heliosphere (Decker et al. 2008). On a longer timescale these variations are averaged out. Ion data are investigated by plotting the upstream particle fluxes in log–log axes as a function of the difference between the observation time t and the shock crossing time, Δt = |t − tsh| (see Figure 2). In order to minimize the background contribution to the temporal particle profiles, the data displayed in Figure 1 have been filtered via a threshold method (Stone et al. 2008): only the data for which the background correction was less than 60% of the signal have been considered acceptable. This is a very conservative filter that allows us to analyze data cleaned with a good level of accuracy. After the background correction and filtering, only PL06, PL07, and PL08 channels retain a number of points in the tails of the temporal profiles suitable for a statistical analysis. In order to investigate the behavior of the tails, we made the fit both with a power-law decay for the ion flux, J = A(Δt)−γ, which indicates a superdiffusive transport, and with an exponential decay, J = Kexp(−Vsh|VrΔt|/D), leading to a normal diffusion. Here, Vsh is the shock speed in the upstream solar wind rest frame, and Vr is the relative velocity between the observer, i.e., the spacecraft, and the shock. By considering an error for the flux values given by , since the data analyzed are countings and a Poissonian statistic is reasonable, we estimated the reduced χ2 both for the power-law fit and for the exponential fit (Perri & Zimbardo 2007). The filtered data and the corresponding fitting lines in log–log axes are displayed in Figure 2. Thus, by looking at the results of the fits shown in Table 1, we can see that the reduced χ2 indicates that the power law fits the tails of the ion profiles better than the exponential function, i.e., the transport for ions in those energy channels is superdiffusive. The exponent of the power laws lies in the range γ = 0.68–0.71, leading to a mean square displacement 〈Δx2(t)〉 ∼ t1.29–t1.32. Both the exponential and the power-law fits have been performed over a temporal interval of 200 days, starting from 10 days from the TS front. This time span is much longer than the timescale of the temporal fluctuations shown in Figure 1, so that those variations are effectively averaged out. It is worth noting that a recent MHD numerical simulation by Washimi et al. (2007) indicated that the TS is expected to move at nearly constant speed in this period of time, i.e., from 2007 January to August; therefore our analysis, which assumes a shock moving at constant velocity (see Equation (3)), is appropriate for these data sets. On the other hand, by fitting the data without applying the background (less than 60%) filter, and for time intervals up to 300 days, the results of the fit weakly change, and the power laws always fit the data better than the exponentials.
Figure 2. Power-law fits of the energetic particle fluxes in various energy channels.
Download figure:
Standard image High-resolution imageTable 1. Fit Parameters for the Ion Time Profiles at the Termination Shock
Energy (keV) | γ | α | χ2pl | χ2e |
---|---|---|---|---|
540–990 | 0.70 ± 0.07 | 1.30 | 0.22 | 0.40 |
990–2140 | 0.71 ± 0.08 | 1.29 | 0.18 | 0.25 |
2140–3500 | 0.68 ± 0.15 | 1.32 | 0.05 | 0.07 |
Download table as: ASCIITypeset image
An analysis similar to that reported above could be performed to the termination shock crossing of V1 in 2004 also. However, the particle anisotropy observed by LECP on V1 indicates that the energetic particles are not coming from the termination shock "in front" of the spacecraft, but mostly from the termination shock flanks (Decker et al. 2005). This requires better knowledge of the overall termination shock shape, and a modification of the technique used here; this will to be done in future work.
3. DISCUSSION AND CONCLUSIONS
Our analysis shows that the propagation of energetic ions accelerated at the solar wind termination shock corresponds to superdiffusive transport. This is the first direct experimental evidence of ion superdiffusion in space plasmas, and adds to the novelties brought about by the V2 TS shock crossing. Several considerations are in order.
First, Perri & Zimbardo (2007, 2008a) have found, analyzing the Ulysses data at about 5 AU, that electron transport is superdiffusive, while proton transport is mostly diffusive. Now, the diffusive particle motion in the direction parallel to the magnetic field is due to pitch-angle diffusion, which in turn is due to the resonant interaction between the particle gyromotion and magnetic fluctuations (Jokipii 1966). In other words, in Perri & Zimbardo (2007, 2008a) the superdiffusive behavior of electrons was interpreted as the result of their weak interaction with turbulence, since the magnetic power spectrum is a decreasing power law, so that electrons would resonate with a very low turbulence level, leading to small pitch-angle diffusion. In this connection, proton transport at 5 AU was found to be diffusive because their larger gyroradius allows the resonant interaction with a stronger level of fluctuations. The finding reported here, i.e., that ion transport at the termination shock is superdiffusive, is probably due to the fact that the magnetic turbulence level decreases with the distance from the Sun (Roberts et al. 1990; Zank et al. 1996), although it does not die out because of the input to turbulence given by pick-up ions (see, e.g., Chalov et al. 2004). Indeed, when the magnetic turbulence level goes down, pitch-angle scattering becomes weaker, so that the parallel velocity does not change sign very frequently; a persistent, long-range correlated velocity is one of the characteristic features of Lévy random walks and superdiffusion, as explained in the Introduction. Further, numerical simulations of particle transport in the presence of turbulence show that the transport regimes for parallel transport are dependent on the turbulence level and on the turbulence anisotropy; in particular, parallel superdiffusion is found for low turbulence levels (Zimbardo et al. 2006; Pommois et al. 2007; Shalchi & Kourakis 2007). Note that, in this analysis, we consider that particle transport is mostly parallel; however, the transport perpendicular to the magnetic field gives some contribution to the particle motion, even if it is smaller than the parallel transport (Lee 1983), so that in a first approximation it can be neglected. On the other hand, assuming a planar, one-dimensional shock, from the study of particle time profiles it is not possible to separate the contribution of the parallel transport from the perpendicular one.
One of the major surprises found by the V1 termination shock crossing of 2004 December 16, and confirmed by V2, was that the flux of ACRs did not peak at the termination shock (Stone et al. 2005; Decker et al. 2005; Stone et al. 2008), contrary to expectations. Also, the V2 crossing of the termination shock on 2007 August 31–September 1 found that the supra-thermal ion spectrum is different from that expected from simple diffusive shock acceleration (DSA) (Decker et al. 2008), a process which requires particles to cross the shock many times by moving diffusively. Indeed, the observed spectral index γs ∼ −1.25 implies, according to DSA, a density compression ratio of about 3 (Decker et al. 2008), while from V2 plasma and magnetic field data a compression ratio at the termination shock of about 2 was found (Richardson et al. 2008; Burlaga et al. 2008). In addition, strongly anisotropic distributions upstream of the termination shock were observed by both the V1 and the V2 LECP instrument (Decker et al. 2005, 2008). This implies that one of the basic assumptions for the use of the Parker cosmic ray transport equation, i.e., the near isotropy of the particle distribution, is not satisfied, so that a focused transport approach should be adopted (e.g., le Roux et al. 2007). Here we show that another basic assumption, i.e., that particles propagate diffusively, is not satisfied. The observation of particle anisotropy and beaming is also consistent with superdiffusive transport.
In this connection, our results show that transport parallel to the magnetic field is faster than diffusive. This means that particles can propagate quickly away from the shock, so that the probability of encountering the shock again is decreased with respect to the diffusive case. In other words, the fast, superdiffusive parallel transport found for low energies indicates that ions are not diffusing back to the shock to be further accelerated. The possibility that superdiffusive transport decreases the efficiency of the DSA mechanism matches well the observations of both Voyager spacecraft that ACRs are not accelerated locally at the shock, and that the observed spectral indexes do not correspond to those expected from DSA (Decker et al. 2008). This implies that other acceleration mechanisms have to be investigated, like shock drift acceleration (Ucer & Shapiro 2001), adiabatic compression acceleration (Jokipii & Giacalone 2007), acceleration of supra-thermal tails (Fisk et al. 2006; Fisk & Gloecker 2007), or second-order Fermi acceleration (Ferreira et al. 2007; Perri et al. 2007).
We thank J. Richardson for making available the PLS data on the web, and S. Krimigis for making available the LECP data on the web. This research was partially supported by the Italian INAF and by the Italian Space Agency, contract ASI no. I/015/07/0 "Esplorazione del Sistema Solare."