Abstract
To investigate the inactivation process of Penicillium digitatum spores through neutral oxygen species, the spores were treated with an atmospheric-pressure oxygen radical source and observed in-situ using a fluorescent confocal-laser microscope. The treated spores were stained with two fluorescent dyes, 1,1'-dioctadecyl-3,3,Y,3'-tetramethylindocarbocyanine perchlorate (DiI) and diphenyl-1-pyrenylphosphine (DPPP). The intracellular organelles as well as the cell membranes in the spores treated with the oxygen radical source were stained with DiI without a major morphological change of the membranes. DPPP staining revealed that the organelles were oxidized by the oxygen radical treatment. These results suggest that neutral oxygen species, especially atomic oxygen, induce a minor structural change or functional inhibition of cell membranes, which leads to the oxidation of the intracellular organelles through the penetration of reactive oxygen species into the cell.
Export citation and abstract BibTeX RIS
1. Introduction
The use of non-equilibrium cold plasma has recently attracted much attention in biological fields, such as medicine, agriculture, and fisheries, because of its many advantages including low-temperature processing and short processing time. There have been many reports on the inactivation of microorganisms using non-equilibrium atmospheric-pressure plasma (NEAPP).1,2) Various factors, such as ultraviolet (UV) light, neutral and charged species, and electric fields, are simultaneously produced by NEAPP, and these may synergistically cause the inactivation of microorganisms. In particular, there have been many reports indicating that reactive oxygen species (ROS), including ground-state atomic oxygen [O(3Pj)], hydroxyl radicals (OH), singlet oxygen molecules [O2(1Δg)], superoxide anions (
O2−), and ozone (O3), are effective at inactivating microorganisms, such as Escherichia coli and Bacillus spp.3–24) However, in all of the studies, multiple ROS including charged species, in addition to UV radiation, were involved, and no lifetimes or inactivation rates were determined for individual species. It is therefore indispensable to systematically discuss inactivation effects of individual species on the basis of quantitative measurements.
In previous works, we have inactivated Penicillium digitatum spores using plasma treatments and investigated inactivation mechanisms by considering intracellular changes.25–27) P. digitatum spores cause the formation of green mold on citrus fruits, which is a difficult-to-inactivate postharvest disease. The spores, which are classified as fungi, differ from the bacteria that are typically employed when researching the plasma inactivation in that they have a much more resistant structure, a different composition, and different cell membrane functions. Various biological responses causing inactivation by plasma treatment, namely, (1) lethal oxidation of biomembranes, (2) electrostatic disruption of cell membranes, and (3) biological responses such as apoptosis, are considered. Plasma can trigger a complex sequence of biological responses in tissues and cells; therefore, the inactivation mechanism with plasma treatment is complicated. In many cases of plasma treatment, the morphology of bacteria is changed by effects such as etching, cracking, leakage, and size reduction.9,11,14,16,17,19,28,29) On the other hand, in the case of cancer cells, plasma can induce apoptosis due to a signaling pathway.30–33) Joshi et al. reported that oxidative stress leads to oxidative DNA damage and membrane lipid peroxidation in E. coli treated with a dielectric barrier discharge plasma.34) We observed the change in spores treated with NEAPP by a fluorescent microscopy and compared it with those treated with UV light or moist heat. The intracellular organelles as well as the cell membranes in the spores treated with the plasma were stained with the dye without a major morphological change of the membranes, while the organelles were not stained in the spores treated with UV light or moist heat. Another dye revealed that organelles were oxidized by plasma treatment, in contrast to UV light or moist heat treatments. Moreover, we found an electron-spin-resonance (ESR) signal from the P. digitatum spores by real-time in-situ ESR measurements. The signal was identified to be semiquinone and disappeared with the oxygen plasma treatment.27) These results suggest that only plasma treatment induces a minor structural change or functional inhibition of cell membranes, which leads to the oxidation of the intracellular organelles without major deformation of the membranes through the penetration of reactive oxygen species generated by the plasma into the cell. In these studies using the NEAPP, multiple ROS including charged species, in addition to UV radiation, were involved, and no inactivation rates were determined for individual species. It is, therefore, indispensable to discuss inactivation effects of individual species.
In order to clarify the inactivation effects of neutral oxygen species, we developed an atmospheric-pressure oxygen radical source that supplies only neutral oxygen species.35,36) We measured the densities of O(3Pj), O2(1Δg), and O3, produced by the radical source using vacuum ultraviolet absorption spectroscopy (VUVAS), and showed that the inactivation rate corresponded to the O(3Pj) density against the O2/(Ar + O2) gas flow ratio and exposure distance, while inactivation efficiencies of O2(1Δg) and O3 are negligible.37) We concluded that O(3Pj) is an effective species for inactivating P. digitatum spores.37,38)
In this study, we observed the changes in spores of P. digitatum treated with an oxygen radical source by in-situ fluorescent confocal-laser microscopy in order to investigate the biological responses causing the inactivation through neutral oxygen species. A confocal-laser microscope has a high resolution, especially in the depth direction. From fluorescent cross-sectional images with a z-axis resolution of approximately 0.2 µm, we can analyze the intracellular changes of the spores treated with oxygen radicals in detail. We performed two fluorescent assays to investigate the effects of ROS. One assay involves the use of 1,1'-dioctadecyl-3,3,Y,3'-tetramethylindocarbocyanine perchlorate (DiI) as a probe for a vital fluorescence membrane dye. DiI belongs to a family of cyanine dyes that have been used to investigate the structure and dynamics of cell membranes and artificial lipid bilayers.39–44) The octadecyl group of DiI binds to the acyl groups of phospholipids in the cell membrane owing to the long carbon chain, resulting in the staining of cell membranes. Therefore, we can analyze the changes in the function and morphology of cell membranes. The other assay involves the use of diphenyl-1-pyrenylphosphine (DPPP). DPPP reacts with lipid peroxide in biomembranes and produces DPPP oxide.45,46) Therefore, DPPP assay is used to prove the oxidation of microorganisms.
2. Experimental procedure
Figure 1 shows a schematic diagram of the in-situ fluorescent confocal-laser microscope system with an oxygen radical source. The oxygen radical source (Fuji Machine Tough Plasma) was described in detail in Refs. 35 and 36. The radical source was based on atmospheric-pressure high-density O2/Ar plasma, which generates electrons with a high density of about 1016 cm−3. Charged species and optical radiation from the O2/Ar plasma were blocked by electrodes and the exit aperture of the radical source, so that only neutral species are supplied to the samples. The exit configuration of the radical source used in this study was a slit aperture of 0.5 × 16 mm2. The treatment area was purged with the gases from the radical source using a plastic cover to eliminate the influence of atmospheric gases. The flows of Ar and O2 gases were sufficient to purge the ambient gas from the area surrounded by the plastic cover. A P. digitatum spore suspension of 10 mg/ml was prepared from distilled water, 1% surfactant (Tween20), and spores. Then, 1 µl of the suspension was spotted on a 35-mm-ϕ dish and dried.
Fig. 1. Schematic diagram of experimental setup of in-situ fluorescent confocal laser with an oxygen radical source.
Download figure:
Standard image High-resolution imageFluorescent images were obtained using an inverted confocal-laser microscope (Olympus FV1000-D) equipped with a 100× oil immersion objective lens (Olympus UPLSAPO100×O). Two fluorescent dyes were used to investigate the effects of oxygen radicals. A staining solution consisted of a mixture of 1 µl of DiI aqueous solution (Lonza Walkersville Vybrant® DiI Cell-Labeling Solution, 1 mM) and 99 µl of 1% Tween20. The irradiation of DiI with light from a mercury lamp filtered at a wavelength of 549 nm, where the DiI is bound to the acyl groups of phospholipids in various membranes, produces green fluorescence at 565 nm. Cell membranes and intracellular organelles, such as nuclei, mitochondria, endoplasmic reticula, and Golgi bodies, have membrane structures that are made of phospholipids. Thus, we can analyze the physical destruction of cell membranes or the disappearance of their function. To observe the fluorescence, an excitation filter (500–550 nm), a barrier filter (565–625 nm), and a dichroic mirror (long pass; 560 nm) were used in the microscope.
Another staining solution consisted of a mixture of 10 mg of DPPP aqueous solution (Dojindo Laboratories) and 999 ml of dimethylsulfoxide. DPPP reacts with lipid peroxide and forms DPPP oxide. Lipid peroxide is produced via the oxidation of lipids in biomembranes, which also exist in the intracellular organelles. Thus, DPPP assay can be used to prove the oxidation of P. digitatum by radical treatment. The irradiation of DPPP oxide with light from a mercury lamp filtered at a wavelength of 352 nm produces blue fluorescence at 380 nm. An excitation filter (340–390 nm), a barrier filter (long pass; 400 nm), and a dichroic mirror (long pass; 400 nm) were used for DPPP observation.
For scanning electron microscopy (SEM) observation, spores were fixed in 3% glutaraldehyde solution for 1 h. The suspension was centrifuged at 1,000 × g for 5 min, washed twice with 1% Tween20 solution, and adjusted to a concentration of 0.6 mg/ml. Then, 10 µl of the suspension was spotted on a Nano-percolator (JEOL), air-dried, and coated with gold particles for 20 s.
3. Results and discussion
Fluorescent and transparent microscopic images of P. digitatum spores stained with DiI are shown in Fig. 2 [(a) control (transparent), (b) control (fluorescent), (c) oxygen radical treatment (transparent), and (d) oxygen radical treatment (fluorescent)]. The O2/(Ar + O2) flow rate ratio and total flow rate were 0.6% and 5 slm, respectively. The spore samples were exposed to radicals 10 mm downstream from the radical head for 1.5 min. The spores were recovered with 300 µl of 1% Tween20, mixed with 3 µl of DiI solution, and incubated at 30 °C for 30 min. Then, 1 µl of the mixture was spotted on a glass-bottom dish (Matsunami) and dried. Figure 2(b) shows that the cell membranes of the spores were stained with DiI. This result shows that the cell membranes had a function of protecting the cell from being infiltrated with DiI, and only the phospholipids in the outer membranes were stained. Namely, the cell membranes in intact spores of P. digitatum are impermeable to DiI because they have selective permeability. The organelles inside the cells treated with oxygen radicals also emitted fluorescent light, as shown in Fig. 2(d). The fluorescent light was observed mainly from nuclear membranes and intracellular organelles, which consist of membrane structures including phospholipids. These results indicate that the neutral oxygen species cause the disappearance of the cell membrane's function, and no major morphological variation was observed in the spore cell membranes. Previously, we showed that the function of the cell membrane was not impaired by other inactivation methods, such as UV radiation and moist heat treatment.26) On the other hand, Cerioni et al. reported that the permeability of the cell membrane of P. digitatum spores increased with oxidative stress, such as by the application of hydrogen peroxide, and that the intracellular organelles became completely disorganized according to the results of transmission electron microscopy (TEM) observation.47) Therefore, oxidative stress leads to inhibition of the selective permeability of the cell membrane and the intracellular structure of spores.
Fig. 2. Fluorescent and transparent microscopic images of P. digitatum spores stained with DiI: (a) control (transparent), (b) control (fluorescent), (c) oxygen radical treatment (transparent), and (d) oxygen radical treatment (fluorescent).
Download figure:
Standard image High-resolution imageFigure 3 shows the time evolution of DiI staining of P. digitatum spores treated with oxygen radicals. Before oxygen radical treatment, the spores were stained with DiI, then the DiI solution containing the spores was treated. The O2/(Ar + O2) flow rate ratio and total flow rate were 0.6% and 5 slm, respectively. At 0 min treatment (before radical treatment), the fluorescence arising from not only the cell membrane, as shown in Fig. 2(b), but also the DiI solution around the spores, as a background, was detected. The fluorescence of the DiI solution disappeared, in contrast with that of the cell membrane, because the DiI dye easily interacts with oxygen radicals, resulting in photobleaching. The organelles inside the cell emitted fluorescent light after 0.5 min oxygen radical treatment. Then, the fluorescence gradually faded after 20 min treatment. This result indicates that the neutral oxygen radicals react with the surface of the cell membrane at the same time when the neutral oxygen radicals are exposed, which leads to the inhibition of the function of cell membranes, resulting in the penetration of ROS and dye into the spores. After 20 min treatment, the fluorescence of the intracellular organelles faded, which is attributed to the oxidation of bonding sites in phospholipids upon their reaction with DiI or to the oxidative degradation of DiI dye due to excess ROS.
Fig. 3. Time evolution of DiI staining of P. digitatum spores by oxygen radical treatment.
Download figure:
Standard image High-resolution imageFigure 4 shows the ratio of the number of spores intracellularly stained with DiI as a function of treatment time. The ratio increases to a maximum value of 87% until 3 min at an exposure distance of 10 mm, and then gradually decreases. On the other hand, at an exposure distance of 20 mm, the ratio increases to a maximum value of 85% until 7 min. The decimal reduction time (D-value), which corresponds to the time required to inactivate 90% of the spores, increases from 1.2 to 19.8 min with increasing exposure distance from 10 to 20 mm, and more than 99.99% of the spores are inactivated after the treatment for 7 min. From the results of the density measurement of O2(1Δg), O(3Pj), and O3 by VUVAS, the O(3Pj) density drastically decreases from 2.3 × 1014 to 1.3 × 1013 cm−3 as the exposure distance is changed from 10 to 20 mm. On the other hand, the O2(1Δg) density is almost constant at 8.0 × 1014 cm−3 and the O3 density is below the detection limit of about 2.5 × 1013 cm−3. These results indicate that O(3Pj) is the crucial species responsible for the inactivation of P. digitatum spores, with only minor roles being played by O2(1Δg) and O3.37) The rate of increase of the ratio at 10 mm is larger than that at 20 mm until 5 min as shown in Fig. 4. This is because the dose of O(3Pj) to the spores at 10 mm is larger than that at 20 mm. The decrease in the ratio of the number of intracellularly stained spores after 3 min at 10 mm is considered to be caused by excess ROS, which peroxidizes acyl-group sites in phospholipids to react with DiI. A similar behavior should occur at 20 mm. From these results, it is concluded that the treatment with neutral oxygen radicals introduces molecules, such as dye molecules and ROS, into spore cells.
Fig. 4. Ratio of the number of spores intracellularly stained with DiI as a function of treatment time.
Download figure:
Standard image High-resolution imageFluorescent and transparent microscopic images of P. digitatum spores stained with DPPP are shown in Fig. 5 [(a) control (transparent), (b) control (fluorescent), (c) oxygen radical treatment (transparent), and (d) oxygen radical treatment (fluorescent)]. The O2/(Ar + O2) flow rate ratio and total flow rate were 0.6% and 5 slm, respectively. Spores were exposed to radicals 10 mm downstream from the radical head for 7 min. After the spores were recovered with 300 µl of 1% Tween20, 60 µl of the suspension was mixed with 6 µl of 1 mg/ml DPPP solution (Dojindo Laboratories) and incubated at 60 °C for 60 min. Then, 1 µl of the mixture was spotted on a glass-bottom dish and dried. Figure 5(d) shows that fluorescent light was emitted from intracellular organelles treated with oxygen radicals. DPPP reacts with lipid peroxide and forms DPPP oxide. Lipid peroxide is produced via the oxidation of lipids in biomembranes, which also exist in the intracellular organelles. This result indicates that the cell was oxidized by oxygen radical treatment.
Fig. 5. Fluorescent and transparent microscopic images of P. digitatum spores stained with DPPP: (a) control (transparent), (b) control (fluorescent), (c) oxygen radical treatment (transparent), and (d) oxygen radical treatment (fluorescent).
Download figure:
Standard image High-resolution imageFigures 6(a) and 6(b) show SEM images of control spores and spores after 7 min oxygen radical treatment, respectively. The images indicate that no major morphological variation occurs even in spores treated with neutral oxygen radicals for 7 min.
Download figure:
Standard image High-resolution imageFig. 6. SEM images of P. digitatum spores: (a) control and (b) oxygen radical treatment.
Download figure:
Standard image High-resolution imageFrom the results of DiI staining, the function of the spore membrane was inhibited by oxygen radicals, which led to the penetration of molecules including DiI and ROS into the spores. The results of DPPP staining suggest that the production of lipid peroxide (LOOH) in the spores was induced by neutral oxygen species. In general, the radical-initiated autoxidation of lipids, which produces LOOH, occurs naturally in vivo without the induction of ROS.48) The increase in the amount of LOOH by oxygen radical treatment enhances DNA damage and enzyme inactivation, leading to the rapid inactivation of spores. In this study, we found that neutral oxygen species, especially O(3Pj), cause not only a minor structural change or functional inhibition without a major morphological change of the cell membranes but also the oxidation of intracellular organelles, resulting in the inactivation of spores.
4. Conclusions
To investigate the inactivation process of Penicillium digitatum spores by neutral oxygen species, the spores were treated with an atmospheric-pressure oxygen radical source, which eliminates the effects of radiation and charged species. The treated spores were stained with two fluorescent dyes and observed in-situ using a fluorescent confocal-laser microscope. The intracellular organelles as well as the cell membranes in the spores treated with the oxygen radical source were stained with DiI without a major morphological change of the membranes. Moreover, DPPP staining revealed that the organelles were oxidized by oxygen radical treatment. The experiment using oxygen radical source showed that the dose of atomic oxygen strongly affected the oxidation of the intracellular organelles and the inactivation rate, whereas the effects of O2(1Δg) and O3 are small. From these results, it was found that neutral oxygen species, especially atomic oxygen causes a minor structural change or functional inhibition of cell membranes, which leads to the oxidation of the intracellular organelles through the penetration of reactive oxygen species into the cell.
Acknowledgements
This work was partly supported by a Grant-in-Aid for Scientific Research on Innovative Areas, "Frontier Science of Interactions between Plasmas and Nano-interfaces" (No. 21110006) from the Ministry of Education, Culture, Sports, Science and Technology of Japan.
Biographies
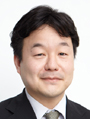
Hiroshi Hashizume received the bachelor and master degrees of agriculture from Nagoya University, Nagoya, Japan, in 1999 and 2001, respectively, and the Ph. D. of pharmacy from Nagoya City University, Nagoya, Japan, in 2011. From 2011, he has belonged to the department of electric and electronic engineering in Meijo University, Nagoya, Japan, as a postdoctoral fellow. His current research interests include plasma bio-applications, such as inactivation and growth regulation of microorganisms with plasma treatment. He is a member of the Japan Society of Applied Physics.
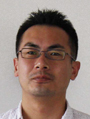
Takayuki Ohta received the B.E., M.E. degrees in Electrical and Electronic Engineering from Shizuoka University, Japan, in 1998 and 2000, respectively, and the Ph. D. (Eng.) in Quantum Engineering from Nagoya University, Japan, in 2003. From 2003 to 2011, he worked in the faculty of systems engineering, Wakayama University, Japan. He is currently an Associate Professor in the faculty of science and technology, Meijo University, Japan. His research interests are plasma processing such as advanced material and biological applications, and optical sensing techniques using interferometric or spectroscopic method. He is a member of the Japan Society of Applied Physics and the Institute of Electrical Engineers of Japan.
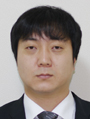
Keigo Takeda received the B.E., M.E. degrees from Wakayama University, Wakayama, Japan, and Ph. D. from Nagoya University, Nagoya, Japan, in 2002, 2004, and 2007, respectively. His current research interests include reaction mechanisms of activated species in plasma processes for bio and medical applications, advanced materials synthesis, fine processing technology, etc. He is a member of the Japan Society of Applied Physics.
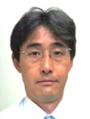
Kenji Ishikawa is a project professor of the plasma nanotechnology research center of Nagoya University, Japan from 2009. Currently he also joins the plasma medical science global innovation center of Nagoya University, Japan. He is currently engaged in studied on novel plasma innovative technologies such as plasma nanoscience, plasma medicine, and plasma agriculture. His research interest focuses on plasma-biochemical reactions involving free-radicals on living organisms. He received the Ph. D. degree from Tohoku University, Japan. He was awarded the best poster award from the Material Research Society (MRS) in 2003, the best paper or the 72nd symposium on semiconductor and integrated circuits technology from the electrochemical society of Japan in 2009, the plasma science award of APCPST&SPSM in 2012, the 11th plasma electronics award from the Japanese Applied Physics Society (JSAP) in 2013.
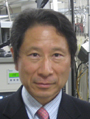
Masaru Hori received the B.E., M.E. degrees from Waseda University, and Ph. D. from Nagoya University, Nagoya, Japan, in 1981, 1983, and 1986, respectively. During 1986–1992, he worked in Toshiba Corporation. He joined Nagoya University, Nagoya, Japan, in 1992, where he is currently an Professor in the department of electrical engineering and computer science and a Director in the Plasma Medical Science Global Innovation Center. In 1997, he was stayed in Cavendish Laboratory, University of Cambridge in United Kingdom as an invited researcher. His current research interests include radical controlled plasma process sciences and technologies which are extending to bio and medical applications as well as advanced material and device ones. He is a member of the Japan Society of Applied Physics.
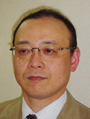
Masafumi Ito received the B.E., M.E., and Ph. D. degrees in electronic mechanical engineering from Nagoya University, Nagoya, Japan, in 1996, 1998, 1992, respectively. During 1991–1997, he belonged to electronic information engineering and quantum engineering in Nagoya University as a research associate and an assistant professor to study laser sensing and plasma diagnostics. During 1997–2009, he belonged to opto-mechatronics in Wakayama University as an associate professor and a professor to study optical sensing system for controlling plasma and plasma inactivation of spores. From 2009, he belonged to electric and electronic engineering in Meijo University, Nagoya, Japan. His current research interests include plasma bio-applications and basics and optical sensing. He is a member of the Japan Society of Applied physics, the Institute of Electrical Engineers of Japan, and the Optical Society of Japan.