Abstract
The aim of this study is to model the nature of nonlinear torsional magnetohydrodynamic waves propagating in solar jets as they are elevated to the outer solar atmosphere. The contribution of sequential processes to the transfer of energy is taken under consideration: the nonlinear cascade and shock formation. Thus a straight magnetic cylinder embedded in a plasma with an initial magnetic field and parallel flow to the cylinder axis is implemented. To resemble a jet where the oscillation wavelength highly exceeds the radius, the second-order thin flux tube approximation proves adequate. A Cohen–Kulsrud type equation is presented, and its solution highly depends on the parameter presented in this study, which itself is constituted of various environmental and equilibrium conditions that affect the perturbations of the variables as well as the nonlinear forces connected to Alfvén wave propagation. The shock formation time of torsional waves is inversely proportional to the density contrast of the jet, while the efficiency of energy transfer to shorter scales is directly proportional to the density contrast. While the parallel flow with a shear at the boundary expedites shock formation, its efficiency regarding energy transfer is dramatically enhanced by the plasma-β, significantly contributing to coronal heating. The observational and seismological aspect of the present study is that faster jets are less probable for observations at higher altitudes, as they experience energy transfer mostly at the base of the corona, while slow speed jets may be observed at higher altitudes contributing to solar wind acceleration.
Export citation and abstract BibTeX RIS
1. Introduction
Solar jets are prominent features of the solar atmosphere autographed by high velocity outflows in the shape of an inverse letter Y from lower to higher levels (Shibata et al. 2007; Moreno-Insertis et al. 2008). A breakthrough regarding the study of solar jets was observed when the X-ray telescope on board Hinode not only enabled the visualization of 10 times the number of jet sightings provided by previous instruments (Golub et al. 2007), but also a far better resolution regarding the oscillations hosted by jets (Cirtain et al. 2007; Vasheghani Farahani et al. 2009). The fact of the matter is that outflows together with the wave dynamic aspect of solar jets provide insight into two famous physical procedures in solar physics: the solar wind acceleration and coronal heating.
Solar jets depending on their size are put into three main divisions, namely, X-ray jets, EUV jets, and spicules jets. The X-ray jets are the largest with foot point distances of about 104 km, which are also the hottest as they are observed in the X-ray band. The EUV and spicule jets, respectively, have a foot point distance of 103 and 102 km (Shibata et al. 2007). Nonetheless, the influence and character of smaller size jets regarding solar weather and wind acceleration must not be undermined. The reason for this statement is that although these jets might carry less energy because of their size compared with larger jets, e.g., X-ray jets, they come into play more frequently with speeds ranging between 57 and 265 km s−1 providing mass for the solar wind (Cho et al. 2019), which is a crucial aspect regarding life on Earth. This is while X-ray jets are observed to posses speeds up to 800 km s−1 with an extent about 105 km (Cirtain et al. 2007). The fact of the matter is that no matter how far into the solar atmosphere the jets extend, subject to environmental conditions, they host physical processes that provide knowledge not only for astrophysics but for various domains of science, e.g., fluid dynamics, plasma physics, atmospheric sciences, etc. The present study exactly complies with the matter since it presents the distribution, transfer, and transport of energy due to Alfvén wave (Alfvén & Lindblad 1947) propagation in jets in connection with environmental conditions and possessions as they reign over two aspects of wave dynamics: shock formation (Suematsu et al. 1982; Im et al. 2009; Ciardi et al. 2013) and nonlinear cascade (Vasheghani Farahani et al. 2012; Told et al. 2015; Grošelj et al. 2018). It is worth noting that the study of jets is being pursued in other scientific domains, namely, the liquid helix connected to the teapot effect (Jambon-Puillet et al. 2019), offset jets connected to power plants and dams (Boroomand & Mohammadi 2019), sediment transport by jets (Boroomand et al. 2007), and nozzle shapes and whipping instability connected to jets (Blanco-Trejo et al. 2020), etc.
Why focus on Alfvén waves in jets now? The answer lies in the concept of Alfvén waves. Either by themselves or by inducing compressive oscillations (Vasheghani Farahani & Hejazi 2017), these waves are the most favorable candidates for transferring energy (Uchida & Kaburaki 1974; Jess et al. 2009; Soler et al. 2019) in the solar atmosphere, besides the energy transported from the solar photosphere to the solar system by the solar wind (Hollweg 1990; De Pontieu et al. 2007; Ofman 2010; Matsumoto & Suzuki 2014). When discussing the induced compressive perturbations in jets, we must ask what their role is in the propagating nature of the Alfvén wave in the presence of a parallel flow while elevating from the solar photosphere to the corona and beyond. The motivation for the present study is rooted in the observation of solar jets in various layers of the solar atmosphere that host MHD waves (Cirtain et al. 2007; Jess et al. 2009; Cho et al. 2019), where the conditions for shock formation and energy transfer from larger scales due to the nonlinear cascade is modeled by implementing the MHD theory.
Before proceeding, it is worth stating that the present study was inspired by a series of studies on the nonlinear effects connected to torsional Alfvén waves in cylindrical plasma structures. That is, by implementing the second-order thin flux tube approximation (Zhugzhda 1996), various aspects of the interaction between the torsional Alfvén wave and the compressive perturbations have been highlighted. For a static and untwisted magnetic cylinder embedded in a vacuum medium, where the perturbations of the total external pressure do not count, the induced compressive perturbations by nonlinear effects connected with the torsional Alfvén wave in the long wavelength limit are independent of the plasma-β, which is expressed by the relation provided by Vasheghani Farahani et al. (2011) that connects the induced density perturbations to the nonlinear forces connected by the torsional Alfvén wave. Another aspect of this configuration is that regarding propagating torsional Alfvén waves the two nonlinear forces, namely the centrifugal and magnetic tension, cancel out each other's effects (Vasheghani Farahani et al. 2011). When the external medium is not vacuum and thus the perturbations of the external medium are taken into consideration, a more general expression for the induced density perturbations due to the nonlinear torsional Alfvén wave in the presence of a parallel flow is provided, where because of the shear at the boundary of the magnetic cylinder the influence of the plasma-β becomes prominent (Vasheghani Farahani & Hejazi 2017). This provided motivation for the present study to investigate the effects of the plasma-β on the back reaction of the compressive perturbations on the torsional Alfvén wave due to the parallel flow with shear at the boundary in the context of nonlinear cascade and shock formation. It is known that, although the plasma-β is effective on the nonlinear cascade and shock formation of shear Alfvén waves, the nonlinear cascade and shock formation regarding torsional Alfvén waves are independent of the plasma-β, see Vasheghani Farahani et al. (2012) for details.
2. Model and Equilibrium Conditions
In order to resemble a solar jet at its equilibrium state, consider a vertical magnetic cylinder that possesses an equilibrium magnetic field (Bzi0) along its axis aligned with the z-component of the cylindrical coordinates (r, ϕ, z), which are embedded in a static magnetic medium and also possess an equilibrium magnetic field (Bze0) along the z-axis, where the indices i and e, respectively, represent the medium internal and external to the jet, see Figure 1. As jets are nonstatic, a steady flow along the z-axis (U0) is also taken into account, which stands for the shear at the boundary between the internal and external media. The equilibrium density and pressure are represented by ρ0 and p0. As jets are large-scale events with oscillations observed by satellites, e.g., Hinode, STEREO, and SDO, a macroscopic proves adequate for working out their properties and characteristics in the context of coronal seismology. Hence, in the present study the MHD theory is implemented for modeling the wave dynamics hosted by these rich physics plasma structures. The ideal MHD equations are





where considering the plasma or fluid medium together with the shape of the structure, enables modeling of various MHD wave propagations (Roberts & Webb 1978; Edwin & Roberts 1983; Nakariakov & Roberts 1995; Terra-Homem et al. 2003) as well as the physical mechanisms they experience (Goossens et al. 1992; Vasheghani Farahani et al. 2014; Chen et al. 2018; Karamimehr et al. 2019). By Taylor expanding the physical variables B, v, ρ, and p around the cylinder axis located at r = 0 before substituting the physical variables by the sum of their equilibrium (B0, v0, ρ0, and p0) and perturbed parts (B1, v1, ρ1, and p1) would enable expressions for the perturbations of the physical variables with respect to each other (Zhugzhda 1996; Vasheghani Farahani et al. 2010). It is worth noting that the Taylor expansion of the physical variables around the tube axis, taking into account only the linear dependencies with respect to the radius r (the second-order thin flux tube approximation), prevents us from accounting for the effects of the radial structure (Edwin & Roberts 1983) of the torsional Alfvén wave.
Figure 1. Diagram of a jet representing the equilibrium conditions under consideration in the present study, where B, p, ρ, and U0, respectively, represent the magnetic field, pressure, density, and a steady parallel flow that causes an infinite shear at the boundary of the jet with its exterior. The indices i and e stand for internal and external to the jet. See also Vasheghani Farahani et al. (2009) regarding kink oscillations in solar jets.
Download figure:
Standard image High-resolution image3. Results and Discussion
As the aim of the present study is to determine the Alfvén wave dynamics in jets, where, due to their cylindrical structure, symmetric torsional polarization is required, the azimuthal wavenumber must be taken zero. This means that the derivative of the physical variables with respect to the azimuthal angle is equal to zero. By considering weakly nonlinear dynamics governed by the MHD set of Equations ((1)–(5)), the jet collimation is balanced by the equation consisting of the forces connected with the internal and external pressures together with the centrifugal, magnetic tension, and ponderomotive forces as (Vasheghani Farahani & Hejazi 2017)
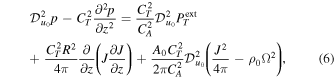
where A0 is the equilibrium tube cross section equal to πR2 and represents the local Alfvén speed, while the operator
is equal to ∂/∂t + u0∂/∂z. The plasma-β measures the ratio of the plasma pressure (p) and the magnetic pressure (B2/8π), while PT
ext represents the total pressure external to the jet. The current density J is equal to Bφ
/r and the vorticity Ω is equal to vφ
/r, where they are held accountable for the ponderomotive, centrifugal, and magnetic tension forces, respectively, on the rhs of Equation (6). Note that in obtaining Equation (6), since the jet radius is over an order of magnitude smaller than the jet length, the terms with higher orders of jet radius have been neglected with respect to terms proportional to the radius squared (R2). Equations (1)–(5) also allow us to provide the effects on the nonlinear terms in the presence of the steady parallel flow that causes an infinite shear at the boundary of the jet with its exterior on J, which is the working variable representing the torsional wave as
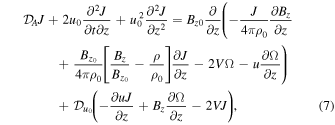
where, in the absence of the parallel flow while considering a nonvacuum, the external medium is simplified to Equation (17) of Vasheghani Farahani et al. (2012). In order to provide insight on the dynamics of the torsional Alfvén wave in jets, an evolutionary differential equation must be written in terms of the current density (J), which stands for the azimuthal magnetic field, as the polarization suitable for jets is circular (Vasheghani Farahani et al. 2011). A practical method in this regard is to consider the moving frame of reference as ξ = z − (CA + u0)t, where the situation indicates a system with variables that are slowly varying with respect to time τ = t. This allows us to provide expressions between the compressive perturbations and the representative of the nonlinear torsion, J2, by reflecting the effects connected with the parallel flow expressed as (Vasheghani Farahani & Hejazi 2017),

with




Regarding the parameter A in Equation (9) see Vasheghani Farahani et al. (2017).
Substitute the expression of Equations (8)–(12) in Equation (7), while considering the new frame of reference, to obtain a closed evolutionary equation with respect to J, which governs the properties and characteristics of torsional Alfvén waves in jets as

where we have
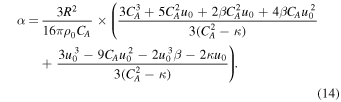
The evolutionary equation presented by Equation (13) is a Cohen–Kulsrud type equation that sheds light on the nature of torsional Alfvén waves at different altitudes and atmospheric conditions, providing implications for coronal seismology. It is worth noting that the constituents of the parameter α present the overall effects of the compressive perturbations together with the environmental conditions on the torsional Alfvén wave. In order to support the assumption that the variations are slowly varying in time, which led to Equation (13), it is worth naming observations of macroscopic plasma structures that exhibit long period and slowly varying large-scale oscillations (Nakariakov et al. 1999, 2006; Cirtain et al. 2007; Jess et al. 2009; Cho et al. 2019). It could be readily noticed that Equation (13) allows us to establish facts regarding torsional Alfvén wave dynamics in solar jets. The presence of the parameter α before the nonlinear term provides valuable information on the action and interplay of all effective compressive perturbations together with the atmospheric conditions internal and external to a solar jet that establish the nature of Alfvén waves. Equation (13) is a general equation suitable for wave dynamics in magnetic structures with outflows that create a shear at the boundary of the collimated plasma structure, especially jets. In the case of static magnetic structure where no parallel flow with a shear at the boundary is present between the internal and external medium (u0 = 0), which best fits a solar loop while the external medium is considered vacuum, the general evolutionary Equation (13) simplifies to Equation (21) of Vasheghani Farahani et al. (2012).
The solution to Equation (13) provides a theoretical understanding and hence seismological applications of torsional Alfvén waves in jets, written in terms of the azimuthal magnetic field (Bφ = JR) normalized by the equilibrium longitudinal magnetic field (Bz0), presented in the form of a Gaussian pulse as
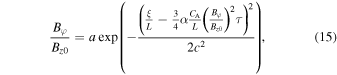
where the amplitude, width, and characteristic length-scale are, respectively, represented by a, c, and L. It is worth stating that the Gaussian pulse, which was the choice for the initial profile of the torsional perturbation of the present study, is not the only profile acting as a solution to Equation (13), thus other initial profiles of the torsional perturbation could be featured instead of the Gaussian profile presented by Equation (15).
The essence of the solution presented in this study (Equation (15)) is observed in the panels and curves constituting Figure 2. This is in a sense that by substituting the parameters associated to various atmospheric conditions, which constitute the parameter α, their role and interplay on the evolution and life of torsional Alfvén waves in various layers of the solar photosphere, chromosphere, and corona is highlighted. It could be observed from the top left panel of Figure 2 that as the density contrast (ρi /ρe ) reduces from infinity to a value around 4, the location of the shock formation shifts from the spatial value of 3 to around 9, which indicates that a static plasma structure, for instance, a solar loop or a very slow jet that hosts torsional Alfvén wave propagation with a lower density contrast, would live longer compared to higher density contrasts. The nonlinear cascade of torsional Alfvén waves for such conditions is pictured in the top right panel of Figure 2 with four curves. The green curve is when the Gaussian pulse is at time zero for all density contrasts, while the blue, red, and black curves, respectively, correspond to the spectrum when the density contrasts are equal to 4, 9, and infinity at the normalized location equal to 3 where the first shock is formed, which is connected to the density contrast equal to infinity (see also Vasheghani Farahani et al. 2012). It could be deduced by the top panels of Figure 2 that since the cascade flattens for higher density contrasts in comparison to lower density contrasts, which look exactly like a water fall, the energy transfer to smaller scales is stronger for higher density contrasts. Now that the contribution of the density contrast regarding Alfvén wave shock formation and spectrum is understood, it is time to provide insight on effects connected with the parallel flow with a shear at the boundary regarding a plasma structure, which specifically resembles a solar jet. The middle and bottom panels of Figure 2 correspond to conditions where a parallel flow with a shear at the boundary of the jet with its external medium exists. As the left panels of Figure 2 are snapshots of the wave evolution before experiencing shocks, it could be readily noticed by the middle and bottom left panels that the presence of a parallel flow with a shear at the boundary expedites the shock formation. The fact of the matter is that shock formation is directly proportional to the parallel flow speed. The efficiency of the parallel flow speed in expediting shock formation itself is directly proportional to the solar jet plasma-β where in the bottom left panel of Figure 2 the plasma-β is taken equal to unity while for the middle panel the plasma-β is taken equal to 0.1. By comparing the middle and bottom panels, it could be noticed that when the parallel flow normalized by the internal Alfvén speed (CA ) is equal to 0.2, the first shock (shown in black) is performed at the location around 4 when the internal plasma-β is equal to 0.1, while for the bottom panel the shock (shown in black) is performed at the location around 3.5 when the internal plasma-β is equal to unity. The corresponding cascades of the profiles just about to experience shocks at photospheric and coronal conditions are shown in the middle and bottom right panels of Figure 2 where the black curves clearly show the efficiency of nonlinear flattening due to higher speed flows. To state clearer, for both plasma-β values equal to 0.1 and 1 the blue curves are the last to experience shocks that correspond to flow speeds equal to 0.2, which could be readily observed to possess the least flattening.
Figure 2. Left panels represent snapshots of the torsional Alfvén wave profile against the normalized running frame of reference (ξ/L) extracted from the Gaussian solution of Equation (15) for various equilibrium and environmental conditions. Right panels represent the spatial spectra connected to the torsional Alfvén waves each corresponding to the pear snapshot presented in the left panels. Note that the middle and bottom panels are plotted for conditions where the plasma-β is equal to 0.1 and 1, respectively, corresponding to solar coronal and photospheric conditions. Regarding the static case surrounded by a vacuum external medium, see Vasheghani Farahani et al. (2012)
Download figure:
Standard image High-resolution image4. Conclusions
In the present study propagation of solar jets in various layers of the solar atmosphere that host nonlinear torsional Alfvén wave interaction with physical variables and parameters together with environmental conditions has been modeled by the MHD theory. Torsional Alfvén waves are the strongest candidates for energy transfer and transport in the solar atmosphere, which clearly means that the understanding of their nature together with their various propagating features would provide insight into the theoretical and seismological aspect of jets and torsional Alfvén waves regarding coronal heating and solar wind acceleration.
The Cohen–Kulsrud type evolutionary equation presented in this study enables the observation of the interplay of various physical variables and parameters together with equilibrium conditions and environmental effects on the shock formation and nonlinear cascade of torsional Alfvén waves connected to dynamic plasma structures not only in the solar atmosphere but also, due to the generality of the equation, in various fluid environments and structures subject to conditions. The findings are summarized as follows:
- 1.A general evolutionary equation for the nature of the torsional Alfvén wave in dynamic plasma structures (especially jets) has been obtained (Equation (13)) that possesses information regarding the influence of the jet density contrast, the internal and external plasma-β effects, and the effects of parallel flow at the jet boundary in the presence of the nonlinear forces, namely, ponderomotive, magnetic tension, and centrifugal, that shape the shock formation, cascade, and evolution of nonlinear Alfvén waves.
- 2.As shock formation regarding torsional Alfvén waves in coronal loops present in a vacuum external medium is independent of the loop plasma-β (Vasheghani Farahani et al. 2012), when a density contrast between the internal and external medium is present (Equations (8)–(9)), the parameters connected to the perturbations together with the nonlinear forces (Equations (8)–(12)) and Equation (14) gain dependence on the internal plasma-β. This dependence is inversely proportional to the shock formation time, which influences the cascade together with energy transfer to shorter scales.
- 3.In the absence of a parallel flow, which resembles a solar loop, neither the variation of the density contrast nor the variation of the external medium plasma-β could temporally or spatially affect shock formation, nonlinear cascade, and evolution of torsional Alfvén waves (Vasheghani Farahani et al. 2012). This is while the presence of the parallel flow with a shear at the boundary, which resembles a jet, advances the shock formation and hence the release of energy (Figure 2).
- 4.In the presence of a parallel flow with a shear at the boundary that resembles a solar jet, the efficiency of the parallel flow regarding shock formation is directly proportional to the jet plasma-β. In finite plasma-β conditions, which are suitable for solar photospheric conditions, the shock formation time and location are short causing energy release in lower altitudes. This is in a sense that shock formation in higher speed jets is dramatically enhanced in photospheric conditions compared to coronal conditions (Figure 2).
- 5.The presence of the parallel flow expedites the cascade flattening providing significant energy transfer to shorter scales. The efficiency of the flattening is dramatically enhanced by the internal plasma-β where for photospheric conditions the energy transfer is much more efficient for higher speed jets in comparison to coronal conditions (Figure 2).
The seismological aspect of the present study is that faster collimated plasma outflows, which resemble jets, create faster parallel flows in the solar atmosphere, preventing them to achieve very high altitudes in the solar atmosphere since their shock experience is expedited. These jets are not significant actors for the solar wind acceleration but instead provide energy transfer to the environment contributing to early stage coronal heating and confirming the observations reported by Cho et al. (2019). This is while jets with slower parallel flows experience retarded shock compared to faster parallel flow jets, allowing them to contribute toward solar wind acceleration. This means that if high speed jets are triggered in the solar photosphere, it may be impossible for them to live long enough to sense higher altitudes of the solar corona.