ABSTRACT
The lithium problem influencing primordial and stellar nucleosynthesis is one of the most interesting unsolved issues in astrophysics. 6Li is the most fragile of lithium's stable isotopes and is largely destroyed in most stars during the pre-main-sequence (PMS) phase. For these stars, the convective envelope easily reaches, at least at its bottom, the relatively low 6Li ignition temperature. Thus, gaining an understanding of 6Li depletion also gives hints about the extent of convective regions. For this reason, charged-particle-induced reactions in lithium have been the subject of several studies. Low-energy extrapolations of these studies provide information about both the zero-energy astrophysical S(E) factor and the electron screening potential, Ue. Thanks to recent direct measurements, new estimates of the 6Li(p, α)3He bare-nucleus S(E) factor and the corresponding Ue value have been obtained by applying the Trojan Horse method to the 2H(6Li, α 3He)n reaction in quasi-free kinematics. The calculated reaction rate covers the temperature window 0.01 to 2T9 and its impact on the surface lithium depletion in PMS models with different masses and metallicities has been evaluated in detail by adopting an updated version of the FRANEC evolutionary code.
Export citation and abstract BibTeX RIS
1. INTRODUCTION
Low-energy cross sections producing or destroying lithium isotopes are among the key ingredients for understanding several astrophysical topics ranging from the big bang to Galactic or stellar nucleosynthesis. Both the stable 7Li and 6Li isotopes have been the subjects of several studies, the former being of primary interest in primordial as well as in stellar nucleosynthesis while the latter is mainly of interest in stellar processes. Recent developments in the detection of 6Li have proceeded despite observational difficulties. 6Li is of interest since it can be used to constrain 7Li-depleting mechanisms and their efficiency. Attempts to solve the long-standing "lithium problem," i.e., the inability of current stellar models to predict the observed surface lithium abundance, has triggered several theoretical works (see, e.g., Deliyannis et al. 2000; Pinsonneault et al. 2000; Charbonnel et al. 2000; Sestito & Randich 2005; Baraffe & Chabrier 2010; Vick et al. 2010). However, uncertainties on the efficiency of some physical processes such as superadiabatic convection, microscopic diffusion, and radiative acceleration (see, e.g., Richard et al. 2002, 2005; Michaud et al. 2000; Turcotte et al. 1998; Gratton et al. 2001) affect the predicted abundances of light elements in stellar atmospheres. In addition, the predicted light element depletion is strongly sensitive to the adopted physical inputs, such as nuclear reaction rates, equation of state, and opacity of the stellar matter (see, e.g., Tognelli et al. 2012). An idea of the uncertainties on the current theories is provided by the discrepancies among recent evolutionary models computed by different authors (Siess et al. 2000; Yi et al. 2001; Dotter et al. 2008; Di Criscienzo et al. 2009; Tognelli et al. 2011). Recently, to solve the discrepancies between inferred and observed abundances (see, e.g., Talon & Charbonnel 1998; Brun et al. 1999; Pinsonneault et al. 1999; Montalban et al. 2000; Talon et al. 2010; Pace et al. 2012), non-standard processes, such as rotation-induced mixing, gravity waves, magnetic fields, and accretion/mass loss, have also been invoked. However, in spite of the strong effort made by the scientific community, the lithium problem remains one of the most interesting open problems in astrophysics. Moreover, the 6Li case is affected by observational difficulties mainly due to the low 6Li abundances in stellar envelopes. High-quality spectra and spectral analysis based on updated atmospheric models are accordingly required (see, e.g., Asplund et al. 2006; Steffen et al. 2012). In most stars, 6Li is completely destroyed during the pre-main-sequence (PMS) phase, where the base of the convective envelope reaches temperatures higher than the 6Li fusion threshold. Moreover, as the convective envelope extension increases with metallicity, one expects to observe residual surface 6Li mainly in low-metallicity halo stars. However, even in such cases, only upper limits are found (Cayrel et al. 1999; Nissen et al. 2000). Recent observations (Asplund et al. 2006, 2008; Garcia Perez et al. 2009) surprisingly found a relatively high value in about ten low-metallicity stars, finding a 6Li plateau as a function of [Fe/H]. However, the reliability of these determinations has been questioned by other works (Spite & Spite 2010; Steffen et al. 2012). Also, in the case of intermediate-metallicity stars, only a few observations are available, as reported in Nissen et al. (1999).
Our understanding of the framework discussed above improves with advances in both observational techniques and theoretical models. For this reason, the low-energy bare-nucleus cross sections of lithium-burning reactions must be known precisely since they are one of the key physical inputs for stellar evolutionary codes. However, direct measurements of nuclear reactions at astrophysical energies are usually difficult. In such a case, the corresponding Gamow window lies at energies at which the cross section values decrease exponentially to the nano or picobarn regime because of the Coulomb barrier between the interacting nuclei (Rolfs & Rodney 1988). Thus, the energy region of interest for astrophysics, ranging for the Li-case from a few keVs up to hundreds of keVs, is usually explored by means of the extrapolated bare-nucleus S(E)b factor

because it smoothly varies with energy, thus removing the exponential drop of the cross section (Rolfs & Rodney 1988).
Indeed, low-energy direct measurements exhibit a cross section enhancement because of the electron screening phenomenon caused by the electron clouds surrounding the interacting ions. This phenomenon represents an additional difficulty in measuring the bare-nucleus cross section (Assenbaum et al. 1987; Strieder et al. 2001). The enhancement factor fenh measured in the laboratory is given by the relation:

where σsh and σb are the shielded and bare-nucleus cross sections, respectively, and Ue is the electron screening potential measured in the laboratory (Rolfs & Rodney 1988).
Focusing on the 6Li(p, α)3He reaction, the direct measurement of Engstler et al. (1992) covers the energy range between 10 keV and 500 keV, with an extrapolated zero-energy S(E) factor of Sb(0) = 3.09 ± 1.23 MeV barns. The 40% error accounts for the uncertainties due to the determination of the absolute cross section. The corresponding electron screening potential values are Ue = 470 ± 150 eV for atomic lithium targets and Ue = 440 ± 150 eV for molecular lithium ones, as explained in Engstler et al. (1992). The most recent 6Li(p, α)3He cross section measurements of Cruz et al. (2005, 2008) span the energy ranges 30–100 keV and 90–1740 keV, respectively, using different lithium-implanted targets. The extrapolated zero-energy S(E) factor is Sb(0) = 3.52 ± 0.08 MeV barns, while the electron screening potential is Ue = 237 ± 111 eV () (Li2WO4 target; Cruz et al. 2005, 2008).
A way to avoid extrapolation to the lowest energies is provided by the application of the Trojan Horse method (THM; Baur 1986; Spitaleri 1990; Cherubini et al. 1996; Spitaleri et al. 1999, 2011). This method allows one to measure the S(E)b factor for charged-particle-induced reactions at astrophysical energies without invoking both Coulomb penetrability and electron screening effects (see Tumino et al. 2007, 2008). In addition, thanks to the validity of the pole invariance, one can approach a given binary reaction using different Trojan Horse nuclei, as explained in Pizzone et al. (2011). However, the TH S(E)b factor needs to be normalized to the available direct measurements performed at higher energies (Spitaleri et al. 2004), thus making the TH a complementary experimental technique for nuclear astrophysics. Two measurements of the 6Li(p, α)3He reactions have been performed applying the THM, with the aim of extending the information on the bare nucleus astrophysical S(E) factor down to the ultra-low-energy region where direct measurements are affected by screening (Engstler et al. 1992, and the NACRE compilation of Angulo et al. 1999). The first measurement described in Tumino et al. (2003) allowed us to investigate the behavior of the 6Li(p, α)3He cross section in the center-of-mass energy range from 2 MeV down to 40 keV. This experiment, besides investigating the astrophysically relevant reaction discussed above, represents proof of the TH validity below and above the Coulomb barrier (the Coulomb barrier for 6Li-p is about 1.1 MeV). Further experiments are discussed in Tumino et al. (2006) and Pizzone et al. (2011). In a second TH experiment, discussed in Tumino et al. (2004), the same two-body reaction has been studied in the low-energy region from 400 keV down to 10 keV. The evaluation of the zero energy S(E) factor was S(0) = 3.00 ± 0.19 MeV barns, while the corresponding electron screening potential was 450 ± 100 eV (details can be found in Tumino et al. 2003, 2004). The two data sets were then included in the work of Pizzone et al. (2005), where this study was devoted to calculating the TH reaction rate using the experimental fit of these two investigations and evaluating its impact during the PMS phase.
The present work is focused on the extraction of the TH S(E) factor for the 6Li(p, α)3He reaction, including the normalization procedure for the TH data discussed by Cruz et al. (2008). The reaction rate is also evaluated and compared with the most recent reaction rate calculation given by the JINA REACLIB of Cyburt et al. (2010).
2. THE TROJAN HORSE METHOD
The THM (Baur 1986; Spitaleri 1990; Spitaleri et al. 1999, 2011; Cherubini et al. 1996) allows the experimentalist to measure the astrophysically relevant cross sections at or very close to the so-called Gamow peak, i.e., in proximity to the energy region at which the reaction under evaluation has a large probability of occuring for a given astrophysical environment. Measurements at the Gamow peak are usually difficult to perform in the laboratory since low cross section values and background contribution result in a very small signal-to-noise ratio (see Rolfs & Rodney 1988; Adelberger et al. 2011). Among the indirect techniques recently developed for nuclear astrophysics measurements, the THM is particularly suitable for determining the cross section for charged-particle-induced reactions. The THM selects the quasi-free (QF) contribution of a suitable a + A → c + C + s reaction, performed at energies well above the Coulomb barrier, to extract the bare nucleus cross section of a charged-particle-induced reaction a + x → c + C at astrophysical energies. The THM allows one to study the A(x, c)C reaction without experiencing Coulomb suppression or electron screening effects. The QF A + a → c + C + s reaction between the projectile a and the target A is usually described by the pole diagram in Figure 1 (Shapiro 1967), where the Trojan nucleus A is chosen because of its large amplitude for the A = x⊕s cluster configuration. As shown in Figure 1, particle a interacts only with the cluster x of the TH nucleus A, while s acts as a spectator of the A(x, c)C virtual reaction. In the plane wave impulse approximation (PWIA), the cross section of the three-body reaction can be factored into two terms corresponding to the poles of Figure 1 via the formula (Neudatchin et al. 1965; Jacob et al. 1966):

where
- 1.KF represents the kinematical factor, dependent on the masses, momenta, and angles of the outgoing particles, which takes into account the final state phase-space factor;
- 2.|Φ(pxs)|2 is given by the Fourier transform of the radial wave function describing the x − s intercluster motion, usually in terms of Hänkel, Eckart, or Hulthén functions depending on the x–s system.
- 3.ll is the orbital angular momentum of particles s and x in the entry channel of the binary subreaction and
is a function of relative momentum and kinetic energy in the entry channel of the binary subreaction (A. Mukhamedzhanov 2012, private communication); and
- 4.[(dσl/dΩ)cm]HOES is the half-off-energy-shell (HOES) differential cross section for the two-body reaction at the center-of-mass energy Ecm.
Figure 1. Schematic representation of the quasi-free (QF) a + A → c + C + s reaction via the pole diagram as in Shapiro (1967). The TH nucleus A breaks up into x and s which are called the participant and the spectator of the binary a(x, c)C reaction, respectively.
Download figure:
Standard image High-resolution imageThe introduction of the penetration factor through the Coulomb barrier, described in terms of the regular and irregular Coulomb functions, and the normalization of the high-energy direct measurements make the extraction of the bare-nucleus S(E) factor possible (Equation (1)). The THM cross section represents the bare-nucleus cross section, as extensively discussed by Spitaleri et al. (2004, 2011). The application of the THM has shed light on different astrophysical problems, including the study of light element burning reactions (Pizzone et al. 2003; Romano et al. 2006; Lamia et al. 2007, 2008, 2012b, 2012c; Tumino et al. 2011a, 2011b), CNO reactions (see La Cognata et al. 2005, 2006; Sergi et al. 2010; La Cognata et al. 2010, 2011), and removing/producing neutron reactions (Tumino et al. 2005; Lamia et al. 2008; Gulino et al. 2010, 2012; La Cognata et al. 2012).
3. THE THM APPLIED TO THE 6Li(p, α)3He REACTION
The 6Li(p, α)3He reaction has been studied via the THM applied to the 2H(6Li, α3He)n QF reaction, using a deuteron as the TH nucleus due to its obvious p–n structure (Tumino et al. 2003). The full details of the experimental set-up and data analysis can be found in Tumino et al. (2003). Here only the most relevant details will be addressed. The experiment was performed at the SMP Tandem van de Graaff accelerator at the Laboratori Nazionali del Sud in Catania by means of a 25 MeV lithium beam impinging on a 250 μg cm−2 thick CD2 (deuterated polyethylene) target. The experimental set-up consisted of two ΔE − E telescopes with 20μ silicon detectors as ΔE and 1000μ position sensitive detectors as E detectors. The two telescopes were placed on opposite sides of the beam, covering, in the laboratory set-up, the angular range 145–24° and 28
3–37
7, respectively. The detection set-up allowed us to cover the QF-angular region, i.e., the kinematic region where a strong contribution of the QF-mechanism is expected. Because of the large amplitude of the s-wave in the p–n intercluster motion, the QF-angular region corresponds to low values of the undetected neutron momentum. However, as explained in Lamia et al. (2012a), the d-wave component in the deuteron wave function leads to a contribution of less than ∼1% in the corresponding p–n momentum distribution. In order to select the region corresponding to a dominant contribution of the QF-component, only events measured in coincidence for neutron momenta ranging from −30 MeV/c and 30 MeV/c were considered in the analysis of Tumino et al. (2003), in agreement with the prescription given in Shapiro (1967), Spitaleri et al. (2011), and references therein.
After normalizing to the direct measurements of Engstler et al. (1992), a value of S(0) = 3.00 ± 0.19 MeV barns was obtained for the zero-energy S(E) factor. The quoted error is only the statistical one, while an additional ∼11% error is due to normalizing to the direct data of Engstler et al. (1992).
The second 2H(6Li, α3He)n TH experiment was performed at the 4 MV Tandem accelerator of the Dynamitron Tandem Laboratorium in Bochum, using a 14 MeV 6Li beam impinging on a CD2 target. The lower beam energy in this second measurement focused on the energy range ∼10–400 keV, relevant for astrophysics. Once normalized to the direct data at that time, the results obtained in this second experiment were compared with the previous results of Tumino et al. (2003), as discussed fully in Tumino et al. (2004).
The TH data of this second experiment are compared with the 6Li(p, α)3He data of Cruz et al. (2005, 2008), thus allowing us to perform a new normalization procedure taking into account the most recent direct measurements. In particular, the more accurate high-energy data of Cruz et al. (2008) affected by an overall error of about ∼6%, together with the direct measurements proposed in the NACRE compilation (Angulo et al. 1999), constitute a single set of experimental results to which the TH data have been normalized. The results of this procedure are shown in Figure 2, where the TH data discussed here are shown as solid red squares, while the direct measurements of Cruz et al. (2005, 2008) are shown as open diamonds (blue and red, respectively). The other direct measurements, as given in the NACRE compilation of Angulo et al. (1999), are also shown. Solid black points represent the data of the previous TH experiment discussed in Tumino et al. (2003). The two sets of TH data shown in Figure 2 have been fit by the function (solid black line in Figure 3)

leading to the value of S(0) = 3.44 ± 0.35 MeV barns for the zero-energy S(E) factor. The total error in S(0) accounts for the statistical error on the TH experimental points (∼7% an average), and on the direct data as well (∼7% an average) and a ∼3% uncertainty due to the normalization procedure. The electron screening potential Ue was extracted by fitting the low-energy (<70 keV) direct data of Engstler et al. (1992) and Cruz et al. (2005) using the formula

leaving Ue as the only free parameter. Sb was parameterized with the expression given in Equation (4) (solid line in Figure 3). The value of the electron screening potential extracted here is Ue = 355 ± 100 eV (dashed line in Figure 3), where the error mainly comes from the ∼12% uncertainty on the low-energy direct data of Engstler et al. (1992). The recent determination of the electron screening potential reported in Lamia et al. (2012c) of Ue = 425 ± 60 eV for the 7Li+p case agrees, within the experimental uncertainties, with the value extracted here, thus confirming the isotopic independence for electron screening phenomena (see Assenbaum et al. 1987 for details). It is worth noting that the experimental value of Ue extracted for the lithium case strongly deviates from the theoretical upper limit of 175 eV. The discrepancy between experimental and theoretical screening potential values has been systematically observed in several reactions (see, for example, Engstler et al. 1992 and Assenbaum et al. 1987); thus electron screening continues to be an interesting problem for low-energy charged-particle-induced reactions.
Figure 2. TH measurement (red squares) normalized to the available direct measurements of Elwyn et al. (1979), Kwon et al. (1989), and Cruz et al. (2008). The previous TH measurements discussed in Tumino et al. (2003) are also shown as black points.
Download figure:
Standard image High-resolution imageFigure 3. TH measurement (red squares) normalized to the available direct measurements of Elwyn et al. (1979), Kwon et al. (1989), and Cruz et al. (2008). The previous TH measurements discussed in Tumino et al. (2003) are also shown as black points. The solid line is the bare-nucleus S(E) factor as given in Equation (4). The low-energy (<70 keV) measurements of Engstler et al. (1992) and Cruz et al. (2005) have been fitted (dashed line) with the formula given in Equation (5), with an electron screening potential of Ue = 355 ± 100 eV.
Download figure:
Standard image High-resolution imageThe 6Li(p, α)3He reaction rate has been extracted here by integrating the TH Sb(E) (as in Equation (4)) over the explored energy range corresponding to temperatures 0.01 ≲ T9 ≲ 2. In the NACRE compilation, the low-energy part of the S(E) factor is described via the extrapolation made by Kwon et al. (1989). A more recent evaluation of the 6Li(p, α)3He reaction rate is provided via the library JINA REACLIB (Cyburt et al. 2010) which also refers to the previous TH investigation of Pizzone et al. (2005). Thus, in order to upgrade the reaction rate, the low-energy trend of JINA REACLIB has been replaced here by the TH measurement (Equation (4)) in the integration of the Sb(E) factor.
The TH reaction rate extracted here, namely NA〈σv〉THM, can then be expressed via the analytical formula proposed by JINA REACLIB7 (Cyburt et al. 2010), namely NA〈σv〉REACLIB, as

where fcorr(T9) is a correction factor given by
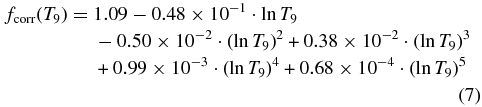
with the temperature T9 expressed in billions of Kelvin. The ratio between the TH reaction rate and that of JINA REACLIB is shown in Figure 4, while the ratio between the adopted and fitted TH reaction rates is reported in Figure 5. From Figure 5, one can set a maximum deviation of ∼2% between the adopted and fitted TH reaction rates for the present TH investigation. This deviation is quite small when compared with the experimental uncertainties discussed here.
Figure 4. Ratio between the adopted THM 6Li(p, α)3He reaction rate and that reported in the JINA REACLIB of Cyburt et al. (2010) (solid blue line), together with THM upper and lower limits (red dashed line). The black dashed area represents the upper and lower limits of the JINA REACLIB rate assuming the same uncertainties given in the NACRE compilation (Angulo et al. 1999).
Download figure:
Standard image High-resolution imageFigure 5. Ratio between the adopted and fitted values of the TH reaction rate. The maximum deviation of ∼2% is considerably lower than the experimental uncertainties discussed in the text.
Download figure:
Standard image High-resolution imageIn Figure 4, the 6Li+p reaction rate deviates by ∼15% at T9 = 10−3 and by ∼5% at T9 = 1 from NA〈σv〉REACLIB. In Figure 6, a summary of the available measurements of the zero-energy S(E) factor and of the electron screening potential are given, leading to average values of 〈Sb(0)〉 = 3.37 ± 0.24 MeV barns and 〈Ue(E)〉 = 346 ± 88 eV. The shaded area in both panels represents the ranges of S(E) and Ue determined by the standard deviations of the different measurements. Even if the S(0) measurements performed over the last decades are consistent, large uncertainties still affect the electron screening potential. Because of such results, together with the deviation from the theoretical upper limit of 175 eV (adiabatic approximation) electron screening continues to be one of the most interesting and unsolved issues of nuclear astrophysics.
Figure 6. S(E = 0) and Ue published values for the 6Li(p, α)3He reaction, with corresponding errors (where given in the original works), as listed in Table 1.
Download figure:
Standard image High-resolution imageTable 1. Recent Results for the 6Li(p, α)3He Sb(0) Factor and Ue Potential
Year of | Paper | S(0) ± ΔS(0) | Ue ± ΔUe |
---|---|---|---|
Publication | (MeV barns) | (eV) | |
1992 | Engstler et al. (1992) | 3.09 ± 1.23 | 470 ± 150 |
440 ± 150 | |||
2000 | Barker (2002) | 3.56 | 260 |
2003 | Tumino et al. (2004) | 3.00 ± 0.19 | ∼450 ± 100 |
2008 | Cruz et al. (2008) | 3.52 ± 0.08 | 237 ± 111 |
2011 | Wang et al. (2011) | 3.63 ± 0.13 | 310 ± 109 |
218 ± 38 | |||
2012 | Present work | 3.44 ± 0.35 | 355 ± 100 |
Download table as: ASCIITypeset image
4. ASTROPHYSICAL IMPLICATIONS
As already discussed in Section 1, the complex problem of 6Li and 7Li abundance evolution is still under debate. Thus, any improvement in one of the many physical inputs/mechanisms that affect stellar surface lithium abundance is worth analysis. In this context, it is interesting to study how the 6Li stellar abundance is affected by the significant variation of the 6Li(p, α)3He reaction rate found with the THM with respect to the JINA REACLIB and NACRE results.
We performed such an analysis for a range of stellar masses and chemical compositions with measured 6Li abundances. In particular, we did model calculations for four different masses (0.6 M☉, 0.8 M☉,1.0 M☉, 1.2 M☉) and three different metallicities ([Fe/H] = −0.5, −1.0, and −2.0).
The present models have been computed with a version of the FRANEC evolutionary code (Degl'Innocenti et al. 2008; Dell'Omodarme et al. 2012) recently updated with particular attention on the physical inputs relevant for the PMS phase (see Tognelli et al. 2011 for details).
For the initial 6Li abundance at different metallicities, we adopted the "standard case" predictions by Prantzos (2012), namely ALi = 1.7, 1.3, and 0.3 for [Fe/H] = −0.5, −1.0, and −2.0, respectively. Note that, while for intermediate metallicities (i.e., [Fe/H] ≈ −0.5), the ALi values of different chemical Galactic models seem to be in quite good agreement (see, e.g., Vangioni et al. 2000; Alibes et al. 2002; Prantzos 2012) and are similar to the ones obtained from abundance observations in the interstellar medium (Howk et al. 2012). For low metallicities, a precise value of the original abundance has not yet been determined (see Prantzos 2012 for details).
However, the quoted uncertainty in the initial abundance is not relevant as the present work is based only on differential analysis.
We focused our calculations on PMS models, as, in standard evolution, surface 6Li is essentially burned in this phase. Figure 7 shows the time evolution of the surface 6Li abundance normalized to the original abundance obtained adopting three different 6Li(p, α)3He reaction rates, namely THM, JINA REACLIB, and NACRE. Surface lithium depletion strongly depends on the temperature at the bottom of the convective envelope. The higher the metallicity, or the lower the stellar mass, the deeper and hotter the base of the convective envelope. This qualitatively explains the different 6Li depletions shown in Figure 7 for various masses and metallicities. Note that among the most massive models (i.e., 1.2 M☉), which have the thinnest external convective envelopes, only the one with the highest metallicity (i.e., [Fe/H] = −0.5) manages to deplete surface 6Li. The figure also shows that, as expected, the adoption of the THM reaction rate leads to results more similar to those obtained with the JINA REACLIB rate compared to those obtained with the NACRE rate, reflecting the previously discussed behavior of the different 6Li burning cross sections.
Figure 7. Time evolution of the surface 6Li abundance, normalized to the original one, when the three different labeled 6Li(p, α)3He reaction rates are adopted in the models. Each panel corresponds, as indicated, to a different chemical composition for which four different masses are evolved. See the text for details.
Download figure:
Standard image High-resolution imageIn general, the effect of adopting different 6Li burning reaction rates, although not negligible, is less important than the effects due to errors in the input parameters (e.g., original metallicity, helium abundance, external convection efficiency) and on the uncertainties in some other physical inputs adopted in the calculations (e.g., opacity evaluation), (see, e.g., the discussion in Pizzone et al. 2005 and Tognelli et al. 2012).
One should also take into account that until 6Li abundance observations in binary stars or 6Li values for different stars in a cluster are available, the uncertainty on the stellar mass will seriously affect any comparison between theory and observations.
5. CONCLUSIONS
The 6Li(p, α)3He reaction represents the main destruction channel for 6Li in several astrophysical environments. Here, we have reported an upgraded reaction rate for such reactions using recently obtained direct data available in the scientific literature to normalize the TH experimental data. The direct data of Cruz et al. (2005, 2008) have in fact been used as new normalization points together with the other experimental data provided by the NACRE compilation (see Angulo et al. 1999). The procedure discussed here returns the values of S(0) = 3.44 ± 0.39 MeV barns and Ue = 355 ± 100 eV. The electron screening potential extracted here strongly deviates from the theoretical upper limit of 175 eV, confirming the systematic deviation already observed in several charged-particle-induced reactions performed at low energies. In addition, the TH Ue value extracted here agrees, within the error, with the value of 425 ± 60 eV deduced by Lamia et al. (2012c) thanks to the recent 7Li+p data analysis, thus confirming once again the isotopic independence discussed in Assenbaum et al. (1987). If compared with the previous TH investigation of Tumino et al. (2004), the extracted S(0) and Ue values deviate by ∼15% and ∼25%, respectively.
The reaction rate determination shows that NA〈σv〉THM deviates from ∼5% to ∼15% as the temperature decreases from 1 down to 10−3 T9. To evaluate the astrophysical implications of these results, we focused our calculations on PMS models since the surface 6Li is essentially burned in this stellar phase. The fate of the surface 6Li abundance normalized to the original one has been studied using three different 6Li(p, α)3He reaction rates, namely the THM, JINA REACLIB, and NACRE with an updated version of the FRANEC evolutionary code.
The different behaviors of the 6Li depletion shown in Figure 7 clearly reflect the influence of the temperature at the bottom of the convective envelope on the final amount of lithium, i.e., the higher the metallicity, and/or the lower the stellar mass, the deeper and hotter the base of the convective envelope.
However, uncertainties on the nuclear reaction rate are less important than those affecting other input parameters such as original metallicity, helium abundance, and external convection efficiency or other physical inputs adopted in the calculations such as the EOS and/or radiative opacity coefficients. So far, the present uncertainties on the stellar mass seriously affect any comparison between theory and observations, thus leaving the 6Li depletion problem an open question in astrophysics.
This work has been partially supported by the Italian Ministry of the University under Grant RBFR082838. The authors thank J. Cruz for useful suggestions given during the writing of the present paper.
Footnotes
- 7
The analytic expression of the JINA 6Li(p, α)3He reaction rate can be found at the link https://groups.nscl.msu.edu/jina/reaclib/db/li6(p, a).